Information Storage and Processing Analysis: The Barn Owl Ear
Alessio Palladino, Laura Jahchan, Aidan Licoppe, Shu Yuan Zhang
Abstract
This report intends to discuss the organ of the ear from an information storage and processing perspective. To do so, the ear of the barn owl is used as a reference. The function of the barn owl ear from such a perspective will first be examined, followed by a detailed architecture of the organ and a rundown on how the structure operates. A comparative analysis is then made with other owl species whose ear architecture is slightly different compared to that of the barn owl. Finally, the analysis shifts to the ageless nature of the barn owl ear.
Introduction
Birds, as a taxonomy, have a rather peculiar sense of hearing. Unlike most mammals who have an external pinna that directs the sound into the ear, birds are devoid of this external part of the ear. A bird's frequency range lies between 1 to 4 kHz, similar to most mammals (Crowell et al., 2015). However, birds are less sensitive to sound overall. The amplitude — or loudness — of a noise needs to be above a certain threshold for the birds to be able to perceive it. That means that most birds are incapable of hearing soft or faint sounds.
Although that is not the case for the nocturnal owl — known to have a very precise and perceptive auditory system, — making it a truly efficient predator for its night hunts. The owl's frequency range is wider than most birds: owls are particularly adept at hearing at frequencies beyond 5 kHz (Krings et al., 2019). They are also known to have a stronger sensitivity to sound between 5 kHz and 8.5 kHz, which allows for sound localization. Owls can detect the slight bristle of leaves a hundred meters away. The owl's evolution has granted the species with a unique structure of its auditory system. This paper seeks to explore the asymmetry of the owl's ears.
Function: Sound Localization
Hearing is an essential component of the sensory system for a large variety of organisms, including vertebrates such as whales and bats, and insects like moths (Göpfert et al., 2016). Among them, the barn owl, which is a nocturnal hunter capable of finding and tracking its prey in the darkness only relying on sounds, is a prime example of how acoustic information aids an animal in its survival (Payne, 1971).
In contrast to shrews, most bats, and cetaceans, which use the active process of echolocation to navigate their environments, owls rely on passive listening as is common to other mammals. Various scientists have taken interest in how the barn owl manages to process and interpret these minuscule vibrations in the air to execute accurate sound localization (Peña and DeBello., 2010). Neuroethologist Masazaku Konishi, based on the field observations of behaviorist Roger Payne, speculated that the owl brain could have a map of auditory space, which accurately directs the birds' talon strikes to prey under groundcover (Peña and DeBello, 2010). By demonstrating that the areas of space that the owls respond to were largely independent of the nature and intensity of sound stimuli, Konishi and Knudsen discovered. with overwhelming evidence, that the barn owls can create a physiological map of auditory space within the midbrain auditory area, as shown in Fig. 1, and thus easily locate the sources of sounds (Knudsen and Konishi, ““A neural map of auditory space in the owl”). This extraordinary ability to detect movements out of sight grants the barn owls a significant advantage over the rodents and small mammals crawling on the ground, especially during the nighttime when vision is obstructed, and hunt their prey with astounding elegance.

Architecture
To achieve the incredible computational feat of constructing auditory space maps with auditory information, the brain of the barn owl has evolved tremendously, tailored to the execution of this crucial operation (Peña et al., 2010). The specialization in the neurological structure of the brain and the auditory nerve, combined with the highly efficient mechanical structure of the ear, allows them to perceive their surroundings through sound with exceptional accuracy.
Outer Ear
The ear opening of a barn owl is placed just behind the eyes, right on the edge of the facial disk groove, as is shown in Fig. 2 (“A Barn Owl's amazing powers of hearing” | The Barn Owl Trust, n.d.).
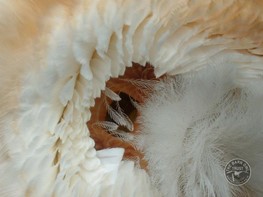
Just like all the other species of owls, barn owls have circular, heart-shaped facial disks covered with loose, fluffy, acoustically transparent feathers that help to protect the ear from whatever bodily harm that the capricious climate could pose. Beneath them are several layers of densely packed, stiff, acoustically reflective feathers known as the ruff (Greij, 2019). Every feather of the ruff has a wide shaft and short barbs, and bends forward near the tip. This structure can help to track and focus sound, funneling sound waves to the ear opening, functioning similar to the outer ear of mammals. In addition, two prominent grooves in the ruff run from either side of the beak up past the owl's ear openings, which serve to filter and direct high-frequency sounds typical of rodent vocalizations (Greij, 2019).
It is worth noting that the ears of the barn owl are asymmetrical: one is found out to be higher than the other as is shown in Fig. 3. This peculiar structure allows them to use not only interaural time differences to determine the horizontal coordinate, but also uniquely use interaural level differences for the vertical component. How the asymmetry is tied to sound localization will be further discussed in the Operation and Sound Localization section of this paper.

Middle Ear
The external auditory meatus in the barn owl, curved in a complex way that differs in the left or the right ears due to skin folds, has a length of about 15 mm from the ear opening to the eardrum (Keller et al., 1998). The eardrum of the barn owl is a triangular-shaped structure at the bottom of the ear canal as is shown in Fig. 4 (Kettler et al., 2016).
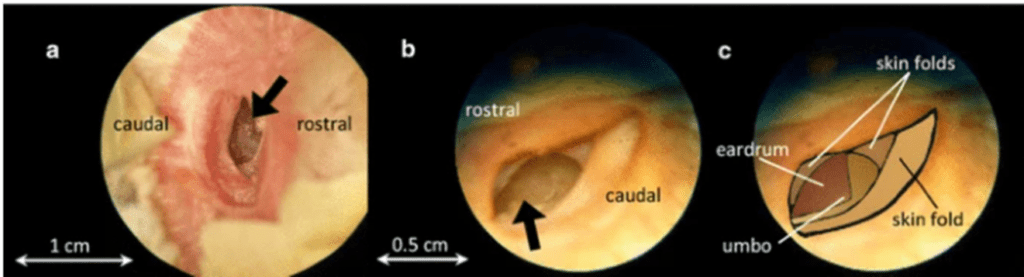
The middle ears of all birds are connected by air-filled interaural canals. For barn owls, it is formed by the Eustachian tubes joining medially before opening into the oral cavity with additional middle ear coupling established by connections from the tympanic cavity and the Eustachian tube into the sphenoid bone cavities (Kettler et al., 2016). Fig. 5 shows the reconstruction of a barn owl skull (Kettler et al., 2016): the interaural canal, an air-filled cavity with a diameter of approximately 3mm at the narrowest cross-section, is indicated by the V-shaped structured marked by green.
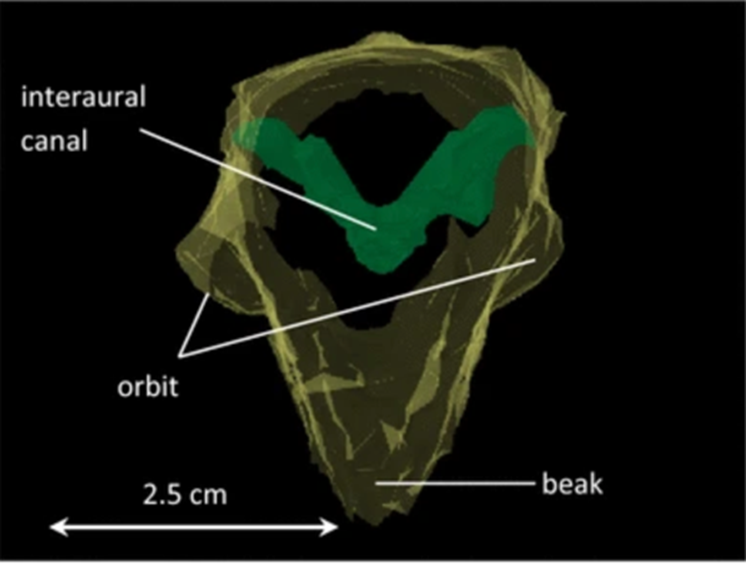
Despite its remarkably broad audible frequency spectrum compared with the other birds, ranging from 200 Hz to 10 kHz, the barn owl does not use the entire hearing range for precise sound localization. Konishi (1973, as cited in Konishi, 2012) reported that the precision of sound localization would decrease for frequencies below 5 kHz. However, regarding the influence of sound transmission through the interaural canal on eardrum directionality, the low frequency — less than 3 kHz directionality — is generated by acoustic coupling via the interaural canal. Similarly, transmission gain is high for low frequencies, but comparatively lower than the value for high frequencies (Kettler et al., 2016). Therefore, the directionality that we have observed in the above-mentioned frequency regions has no influence on sound-localization behavior; the absence of interaural differences, a major cue for elevational sound localization in barn owls, at low frequencies, makes it difficult to localize frequency sounds compared to frequencies between 5 and 8 kHz, which correspond to the rustling sounds made by the prey (Konishi, 1973, as cited in Konishi, 2012). Accordingly, the interaural canal is not involved at all in sound localization but might still be significant in intraspecific communication since most energy in the calls of adult barn owls is carried by frequencies below 3 kHz (Payne, 1971).
Inner Ear
The tremendous range of hearing for barn owls is closely connected to the unusual structure of its inner ear (Smith et al., 1985). As was shown by Schwarzkopf (1968), the cochlea of owls is significantly longer than that of many other birds, reaching the total length of approximately 12 mm. Furthermore, the basilar papilla of barn owls has two unique features, namely a proliferation of lenticular short cells and a thickening of the basilar membrane on the basal end, both of which contribute their enhanced auditory systems (Smith et al., 1985).
The membranous cochlear duct is composed of the basilar papilla and related nerve fibers, the tectorial membrane, the tegmentum vasculosum, and the macula of the lagena. Due to the asymmetry in the anatomy of ears, the left cochlea –12.5 mm with basilar papilla 11.5 mm in length – is slightly larger than its right counterpart, which is about 12 mm with basilar papilla of 10.5 mm (Smith et al., 1985). The overall structure of the cochlear duct in barn owls is similar to that of other birds. The basilar papilla, formed by supporting a sensory cell, is arranged in a compact mass on a fibrous basilar membrane. The free apical surfaces of hair cells and supporting cells are covered by a thick tectorial membrane which has a wide attachment area at the epithelial cells. The basilar membrane is suspended between the two fibro-cartilaginous plates and it separates the perilymph of scala tympani from the endolymph of scala media which is closed off by a heavy tegmentum vasculosum shown in Fig. 6 (Smith et al., 1985).
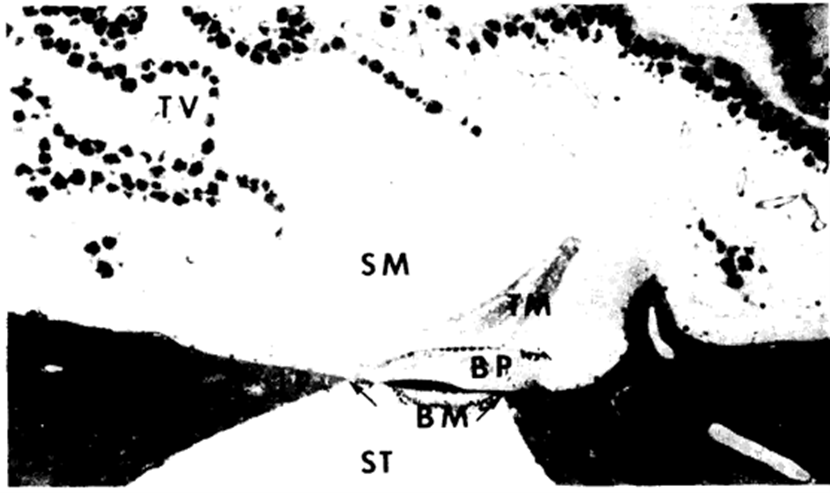
The sensory cells can be divided into three major categories based on their length: tall, intermediate, and short. The tall hair cells have a height that is greater than surface diameter and are present only on the distal half of the barn owl's papilla as is demonstrated in Fig. 7 (Smith et al., 1985).

They make their appearance first at about 5 mm from the proximal end and increase steadily in number towards the distal end, where they are predominant. The intermediate cells are more widely distributed over the papilla but are not especially populous at any place (Smith et al., 1985). On the other hand, the short hair cells have enlarged apical ends with diameters greater than their heights. These sensory cells include the type with broad apical end covered by a cuticular plate, which is also common in other birds. This common type of short cells has a wider distribution than the tall hair cells in the barn owl, found on all parts of the papilla except for the proximal and distal tips, with the former occupied solely by the lenticular cells (Smith et al., 1985). The lenticular cells, which are an uncommon type of short hair cells, have a large luminal surface, but only about one-third of this free area is covered by the cuticular plate. They can be differentiated from the other short cells even at a light-microscopic level of magnification since the cuticular plate plus ciliary bundle are on the superior side of the cell. In contrast, the ciliary bundles of other short cells are on the inferior side or more centrally placed (Smith et al., 1985). The lenticular cells are numerous on the proximal one-third of the papilla and occupy the entire first millimeter of basilar membrane. This abundant presence is rarely observed in other types of birds; Tanaka and Smith (1978) found a few on the basal tip of the chicken's papilla, which proved to be crucial to high-frequency reception. Through the unusual arrangement of cell types, the barn owl manages to acquire a higher sensitivity for sound waves of high frequency greater than 5 kHz, thus greatly improving the efficiency of sound localization.
The basilar membrane is a fibrous, extracellular structure, almost identical to that of all vertebrates (Smith et al., 1985). In birds, it is attached to the two fibro-cartilaginous plates with a narrow and elongated shape. It is observed to have two thickenings: the vestibular dens fibrous mass and the tympanic loose fibrous mass. The latter is present in other birds such as the pigeon and the chicken. However, the dense vestibular fibrous mass seems to be unique to the barn owl based on the current data (Smith et al., 1985). The thickenings facilitate high frequency hearing and could be an important factor contributing to the exceptional high-frequency discrimination required for sound localization demonstrated in the barn owls (Quine and Konishi, 1974).
Midbrain and Forebrain
Auditory perception depends on multi-dimensional information in acoustic signals encoded by auditory nerve fibers, which are then transported to the midbrain where the information is processed (Fischer et al., “Multidimensional stimulus encoding in the auditory nerve of the barn owl”). Compared to other bird species, owls with asymmetrical ear have substantially larger auditory midbrains for the integration of sound localization cues and the internal representation of the external auditory space (Ashida, 2015). Ascending projections from the interaural time differences (ITD) and the interaural level differences (ILD) pathways systematically converge at the level of lateral shell of the central nucleus of the inferior colliculus (ICcl), the neurons of which change their output spiking rates according to the specific combinations of ITD and ILD. These neurons, tuned to different frequencies but to the same ITD, project to neurons of the external nucleus of the inferior colliculus (ICx) (Fischer et al., “Multiplicative auditory spatial receptive fields”).
The ICx in the midbrain is the location where inputs from the ICcl converge over multiple-frequency channels and the barn owl's auditory space map is constructed (Ashida, 2015). An ICx neuron is excited only when the stimulus sound comes from a certain spatial direction, that is, each ICx neuron has its own preferred, specific combination of ITD and ILD. Thus, the horizontal direction of a sound and the vertical direction are plotted two dimensionally in this section of the midbrain. It is the central location where the external auditory world is mapped internally (Ashida, 2015).
ICx neurons then project to the optic tectum (OT), the avian homologue of the mammalian superior colliculus, where auditory and visual information is integrated. The barn owl's OT inherits both the auditory space map from the ICx and the retinotopic inputs from the visual system (Mazer, 1998). The barn owl has an excellent sight, and the visual input to the OT, which demonstrates an astoundingly clear agreement with the auditory map is used to further calibrate the auditory space map (Ashida, 2015).
Neurons in the OT project to premotor neurons in the brainstem controlling head and neck muscles and induce an owl's head turns (Masino and Knudsen, 1992). Thus, the architecture for the reception and computations of the sound localization cues is completed. This intricate yet highly efficient structure enables the barn owl to capture their prey with astonishing accuracy even in the darkest of nights, to adapt to the environment and establish its unique way of survival.
Operation and Sound Localization
The barn owl has developed an exceptional sound localization ability as a species over its evolution to locate its prey in the dark solely by acoustic signals. The owl can perform this localization through a process called binaural acoustic information processing, or binaural hearing. This refers to the individual's ability to extract information from its surroundings using two ears considered in terms of sound localization cues, interaural time, and interaural intensity differences. Through highly specialized brainstem pathways converging input onto individual neurons, the owl is then able to process these cues very effectively (McAlpine, 2008).
As previously mentioned, barn owls' ears are asymmetrical, with their left ear higher and pointing downwards and their right ear lower and pointing upwards, allowing directly for their ability to localize sound. Because of this, the horizontal location of a sound source is processed through interaural time difference (ITD), and the vertical direction is processed through interaural level difference (ILD) (Singheiser et al., 2012). Given that the ITD and ILD axes are almost orthogonal instead of the typically parallel position of ears, the combination of ITD and ILD provides a 2-dimensional coordinate of the source. Through analysis of owl reaction, the maximum ranges for ITD and ILD were found to be 500-600 μs and 30-40 dB respectively (Ashida, 2015).
An investigation was performed on the mechanisms in which the barn owl determines the azimuth — angular distance — and elevation related to its ITD and ILD respectively. The measure of its localization ability was determined by the accuracy that the owl would orient its head to a source of sound. As shown in Fig. 8, the azimuth is represented by α, and the elevation by θ. This investigation brought forth various conclusions (Knudsen and Konishi, “Mechanisms of sound localization in the barn owl”). Firstly, when localizing tonal signals — of one frequency, — the owl had the smallest margin of error from 4 – 8 kHz. The azimuthal component of this was unaffected from 1-8 kHz while the error of the elevation component increased significantly below 4 kHz. For wide band noise, in which the sound energy is distributed over a wide audible range, the owls mean margin of error was almost 3 times less than its optimal frequency for tonal localization which was at 6 kHz. Because the owl is very reliant on both ears for sound localization, when preventing hearing in the right ear, the owl will orient below and to the left of the sound source while, preventing the left ear, it will orient above and to the right. This result is a natural adjustment following the owl's orientation and angle of both ears (Knudsen and Konishi, “Mechanisms of sound localization in the barn owl”).

As the sound is processed through the owl's inner ear and cochlea, the auditory signal is reverberated from its approximately 16 000 hair cells in its basilar papilla, innervated about 30 000 auditory nerve (AN) afferents (Ashida, 2015). Starting at the AN, the processing of timing and intensity information begins. Entering the brain, the auditory nerve will branch into two pathways: one target being the nucleus magnocellularis (NM) — near the dorsomedial edge of the brainstem — and the other being the nucleus angularis (NA) which is located more laterally. Through selective pharmacological blocking of the cochlear nuclei, it was found that both the ITD and ILD pathways are processed independently of each other. The timing cue, coding for the ITD diverges into the pathway starting from the NM while the intensity cue, as well as most other sound information, coding for the ILD diverges into the pathway starting in the NA. Both pathways will finally converge in the midbrain of the barn owl at the lateral shell of the central nucleus of the inferior colliculus (ICcl). At this point, the signal will undergo the midbrain and forebrain auditory pathways where the signal is translated to sensory physical information (Singheiser et al., 2012).
For the ITD pathway, the timing signal is sent from the NA to the NM whose function is to effectively convey temporal information to the binaural nerves in the nucleus laminaris (NL). A NM neuron will receive 1-4 AN afferents containing large synaptic endings — endbulbs — in which the synaptic connection is so strong that there is almost always a spike caused in the NM. From here, the signal will reach the earliest point where binaural inputs converge: the NL (Ashida, 2015). The barn owl has about 15 000 NL neurons which will vary their output spiking rate periodically with time delay, determining changes in bilateral input timings of significantly less than 1 ms. Variation in ITD results in changes of oscillation amplitude to which the NL neuron will change its output spike rates. These outputs are channeled towards both the anterior part of the dorsal lateral lemniscus (LLDa) or the central nucleus of the inferior colliculus (ICcc) (Ashida, 2015). The primary role of the LLDa is to reduce spike count variability while passing the signal to the ICcc as well, though the benefits of convergence directly from the NL or through the LLDa remain unknown. The ICcc neurons will finally average out neuronal noise for inputs of various neurons with similar ITD tunings and send this signal further to the ICcl (Ashida, 2015).
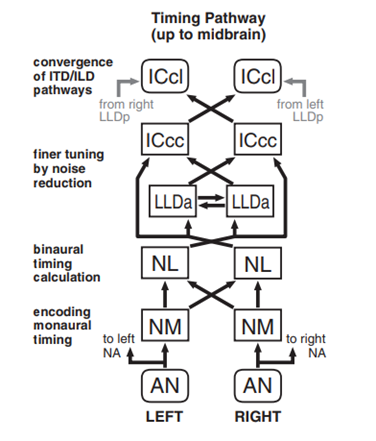
Contrarily, for the ILD pathway, the intensity signal as well as others are instead sent from the AN to the NA. The barn owl has around 17 000 NA neurons which function to receive tonotopic projections — signals referring to the spatial arrangement of where sounds of different frequencies are processed in the brain, — from the AN (Ashida, 2015). Comparatively to the NM neurons in the ITD pathway, NA neurons have longer time windows for synaptic integration – how the neurons combine the inputs received before the generation of an impulse –making them more suitable to process the intensity information given by the AN spike counts received. From the NA, the signal is then sent to the superior olivary nucleus (SO) which will also receive inputs from the NL and the opposite side SO, projecting these back onto the same side NM, NL, NA and the opposite SO (Ashida, 2015). The full role of this complex is unknown, though since it sends inhibitory inputs, it is suggested that its primary role is to balance same side and opposite side input signals to effectively eliminate the effects of ILD on ITD coding. The signals sent back to the NA are then transferred to the posterior part of the dorsal lateral lemniscus (LLDp). This is the location where ILD is first computed and where the LLDp will receive excitatory inputs from the opposite side NA as well as inhibitory inputs from the opposite side LLDp. These ILD-tuned responses are finally transferred to the ICcl in the midbrain converging with the ITD pathway (Ashida, 2015).

Comparative Study
One of the key characteristics of the barn owl's ear architecture is related to the asymmetrical nature of their ears which allows these animals to determine elevation and azimuth properties of auditory signals (Volman et al., 1990). However, it is not all species of owls that exhibit such asymmetrical characteristics. For instance, the great horned owl and the burrowing owl are two species that, instead, have symmetrical ears (Volman, 1994). This symmetrical nature of the organ represents the primitive condition (Volman, 1994). It has been hypothesized that the lack of asymmetrical ears would mean that such species are required to rotate their heads to evaluate sound elevation (Volman 1994). Varying ear architecture in different species of owls can be attributed to their varying needs when performing tasks like hunting. In fact, the time of day during which owls operate the most can potentially be related to the symmetrical or asymmetrical nature of their ears.
Take for instance the previously discussed barn owl. Such a species would strictly be considered nocturnal animals and hunting activities would thus be performed in the darkness of the night (Volman, 1994). Consequently, the nocturnal owl's ability to accurately locate prey greatly depends on its ability to quickly evaluate both the elevation and azimuth of auditory signals. On the other hand, great horned owls are described to be crepuscular or nocturnal and burrowing owls are considered crepuscular or diurnal (Volman, 1994). For reference, crepuscular animals operate during the twilight time of day and diurnal animals are active during daytime. It is evident that owl species that are not strictly nocturnal do not need to rely as heavily on their auditory senses to locate and attack their prey.
Taking this slightly further, burrowing owls are particular animals as they nest underground and are the only North American birds to do so (Moir, 2010). Since it lives on the ground, this owl species has developed hunting techniques that go beyond hunting from a perch. In fact, burrowing owls are also known to make use of their thin, long legs to run after their prey which includes ground insects and small rodents (“Burrowing Owl” | Canadian Wildlife Federation, n.d.). Moreover, the burrowing owl distinguishes itself from other species through its ability to more accurately see colors through their famous yellow irises in the light (Long, 1998). Thus, the need to quickly process two-dimensional auditory signals is not as crucial for their survival, allowing them to continue to live without the asymmetrical hearing techniques present in barn owls.
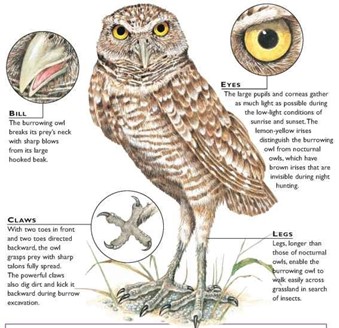
Ultimately, owl species with symmetrical ears have slightly different functions compared to those with an asymmetric system which has allowed their architecture to remain in line with their primitive conditions.
What Can Go Wrong, Or Rather, Why Can It No Go Wrong
One would naturally assume that, with time, an animal's hearing will degrade. Such a fact is true for many beings including humans, mice, gerbils, chinchillas, etc. (Katz, 2017). However, the barn owl distinguishes itself from such species through its ability to accurately hear auditory signals regardless of its age. In fact, a study published in the Proceedings of the Royal Society B demonstrated that barn owls even as old as 23 years of age were still just as able to respond to generated auditory signals when compared to their younger counterparts (Krumm et al., 2017). Thus, the barn owl is not known to have many particular auditory problems but is rather recognized for having ageless ears.
Age-related hearing loss, otherwise known as presbycusis, is a condition common to most mammals and is in part related to the loss of hair cells in the organ of Corti, a region of the ear found in the cochlea (Krumm et al., 2017). The problem lies in the fact that these species who suffer from presbycusis are not able to regenerate hair cells in this region of the inner ear. Hair cell regeneration in mammals has only been observed in vestibular sensory epithelia (Langemann et al., 1999). However, it has been previously studied that, in the case of certain animals like birds, damaged hair cells can be regenerated, thus encouraging Krumm et al. (2017) to evaluate if these properties can help prevent presbycusis throughout the barn owl's entire life cycle (Krumm et al., 2017). Moreover, mammalian age-related hearing loss may also be attributed to a decrease in endocochlear potential caused by the stria vascularis degeneration which has been studied in the case of gerbils (Gratton et al., 1996).
In the study conducted by Krumm et al. (2017), seven owls were divided into two age groups: young and old. To evaluate the animals, the barn owls were tested to see if they would fly to various perches as sounds of frequencies ranging from 0.5 kHz to 12kHz were randomly emitted for a duration of 300 ms. The owls had five seconds to react to the sounds, or else the attempt was deemed unsuccessful (Krumm et al., 2017). The following data was recorded:
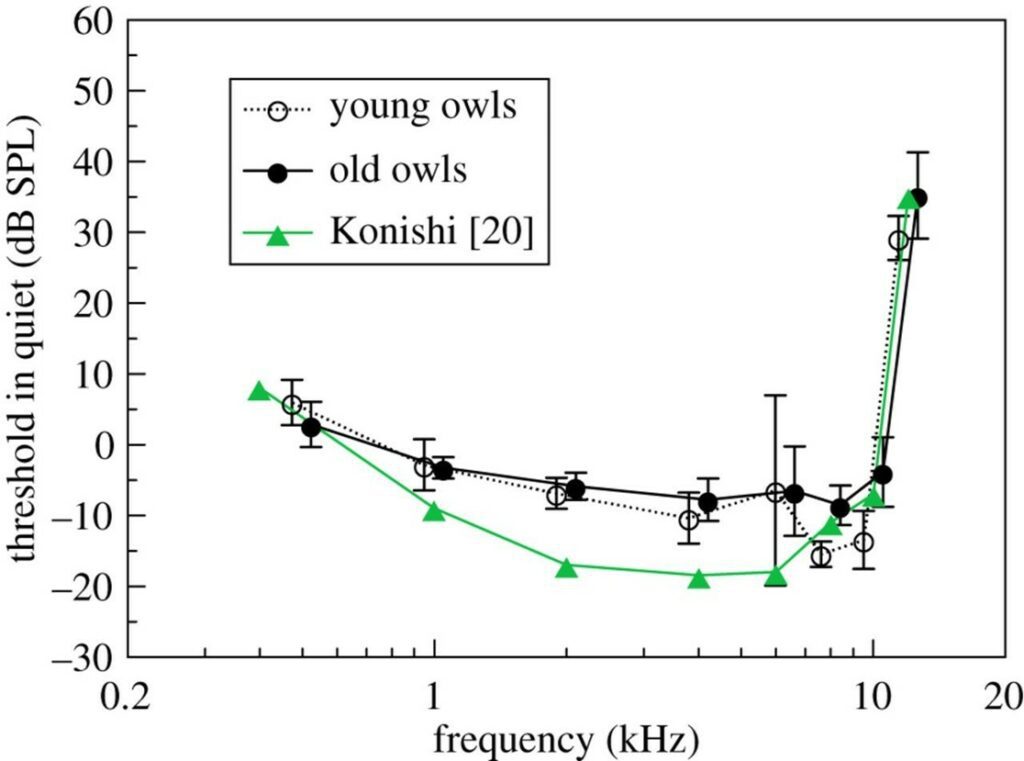
Although it was found that the owls of the younger group were more sensitive by an average of 2.8 dB compared to the older group, Fig. 12 demonstrates that the difference in threshold was not significantly large between the two groups. Thus, the researchers were amazingly able to conclude that the barn owl does not suffer from age-related hearing loss. Moreover, it should be noted that Fig. 12 includes a comparison with Konishi's study published in 1973. These values differ from Krumm et al.'s data (2017) for reasons including the varying threshold criteria, the number of owls tested “carefully” and the use of a different barn owl species group (Krumm et al., 2017).
Evidently, one would assume that barn owls must not be the only bird species on this Earth to not exhibit age-related hearing loss. Such an assumption would be valid as similar research has also been conducted on the European starlings. Langemann et al.'s 1999 study showed that European starlings do not exhibit presbycusis conditions either as can be seen in the auditory threshold graphs of Fig. 13 (Langemann et al.,1999). In fact, the thresholds of European starlings aged 6 to 12 months were, remarkably, almost identical to those aged 8 to 13 years.

Ultimately, one could only hope that a better understanding of the regenerative avian auditory system could one day lead to the invention of technologies that would allow humans to solve their mammalian, age-related loss of hearing. Much work, and future studies must be tackled as time progresses, but the possibilities do remain endless.
Conclusion
The asymmetric ears of the barn owl not only allow the bird to hear at higher frequencies but also to detect the location of the sound at a distance. This peculiar structure can be attributed to a long history of evolution and adaptation to the nocturnal and predatorily ways of the owl. The asymmetrical structure allows for sound location since an infinitesimal time separation exists when the sound reaches one ear and then the other. Another advantage the structure confers is a limitation of hearing loss through time, a condition that affects many mammals and often leads to hearing deficits. The asymmetry of the owl's ear is truly a remarkable result of evolution.
References
Ashida, G. (2015). Barn owl and sound localization. Acoustical Science and Technology, 36(4), 275-285. doi:10.1250/ast.36.275
Berleth, T., Mattsson, J., & Hardtke, C. (2000). Vascular continuity and auxin signals. Trends in Plant Science, 5 9, 387-393.
Bühler, P., & Epple, W. (1980). Die Lautäußerungen der Schleiereule (Tyto alba). Journal für Ornithologie, 121(1), 36-70. doi:10.1007/BF01643255
Crowell, S. E., Wells-Berlin, A. M., Carr, C. E., Olsen, G. H., Therrien, R. E., Yannuzzi, S. E., & Ketten, D. R. (2015). A comparison of auditory brainstem responses across diving bird species. Journal of Comparative Physiology. A: Neuroethology, Sensory, Neural, and Behavioral Physiology, 201(8), 803-815. doi:10.1007/s00359-015-1024-5
Federation, C. W. (n.d.). Burrowing Owl. Retrieved from https://www.hww.ca/en/wildlife/birds/burrowing-owl.html#:~:text=The%20Burrowing%20Owl%20has%20several,Owl%20hunts%20around%20the%20clock
Fischer, B. J., Anderson, C. H., & Peña, J. L. (2009). Multiplicative Auditory Spatial Receptive Fields Created by a Hierarchy of Population Codes. PloS One, 4(11), e8015. doi:10.1371/journal.pone.0008015
Fischer, B. J., Wydick, J. L., Köppl, C., & Peña, J. L. (2018). Multidimensional stimulus encoding in the auditory nerve of the barn owl. The Journal of the Acoustical Society of America, 144(4), 2116-2127. doi:10.1121/1.5056171
Gratton, M. A., Schmiedt, R. A., & Schulte, B. A. (1996). Age-related decreases in endocochlear potential are associated with vascular abnormalities in the stria vascularis. Hearing Research, 102(1-2), 181-190. doi:10.1016/s0378-5955(96)90017-9
Greij, E. (2019). Behavior: How owls can hunt even in total darkness. In. birdwatchingdaily.com. Retrieved from https://www.birdwatchingdaily.com/news/science/owl-behavior-hunting/
Katz, B. (2017). Barn Owls Do Not Suffer From Age-Related Hearing Loss, Study Shows. Retrieved from https://www.smithsonianmag.com/smart-news/barn-owls-do-not-suffer-age-related-hearing-loss-study-shows-180964969/
Keller, C. H., Hartung, K., & Takahashi, T. T. (1998). Head-related transfer functions of the barn owl: measurement and neural responses. Hearing Research, 118(1), 13-34. doi:10.1016/S0378-5955(98)00014-8
Kettler, L., Christensen-Dalsgaard, J., Larsen, O. N., & Wagner, H. (2016). Low frequency eardrum directionality in the barn owl induced by sound transmission through the interaural canal. Biological Cybernetics, 110(4), 333-343. doi:10.1007/s00422-016-0689-3
Knudsen, E. I., & Konishi, M. (1978). A neural map of auditory space in the owl. Science, 200(4343), 795-797. Retrieved from https://science.sciencemag.org/content/sci/200/4343/795.full.pdf
Knudsen, E. I., & Konishi, M. (1979). Mechanisms of sound localization in the barn owl (Tyto alba). Journal of comparative physiology, 133(1), 13-21. doi:10.1007/BF00663106
Konishi, M. (2012). How the Owl Tracks Its Prey. American Scientist, 100(November-December 2012). Retrieved from https://www.americanscientist.org/article/how-the-owl-tracks-its-prey
Krings, M., Müller-Limberger, E., & Wagner, H. (2019). EvoDevo in owl ear asymmetry—The little owl (Athene noctua). Zoology, 132, 1-5. doi:10.1016/j.zool.2018.10.002
Krumm, B., Klump, G., Köppl, C., & Langemann, U. (2017). Barn owls have ageless ears. Proceedings of the Royal Society B: Biological Sciences, 284(1863), 20171584. doi:doi:10.1098/rspb.2017.1584
Langemann, U., Hamann, I., & Friebe, A. (1999). A behavioral test of presbycusis in the bird auditory system. Hearing Research, 137(1-2), 68-76. doi:10.1016/s0378-5955(99)00139-2
Long, K. (1998). Owls: A Wildlife Handbook: Johnson Books. Retrieved from https://books.google.ca/books?id=7WDvVEfDVD8C
Masino, T., & Knudsen, E. I. (1992). Anatomical pathways from the optic tectum to the spinal cord subserving orienting movements in the barn owl. Experimental Brain Research, 92(2), 194-208. doi:10.1007/BF00227965
Mazer, J. A. (1998). How the owl resolves auditory coding ambiguity. Proceedings of the National Academy of Sciences, 95(18), 10932-10937. Retrieved from https://www.pnas.org/content/pnas/95/18/10932.full.pdf
McAlpine, D. (2008). Binaural Pathways and Processing. In M. D. Binder, N. Hirokawa, & U. Windhorst (Eds.), Encyclopedia of Neuroscience (pp. 383-388). Berlin, Heidelberg: Springer Berlin Heidelberg.
Moir, J. (2010). The Little Owls That Live Underground. Retrieved from https://www.smithsonianmag.com/science-nature/the-little-owls-that-live-underground-203543/
Payne, R. S. (1971). Acoustic Location of Prey by Barn Owls (Tyto Alba). Journal of Experimental Biology, 54(3), 535-573. doi:10.1242/jeb.54.3.535
Peña, J. L., & DeBello, W. M. (2010). Auditory Processing, Plasticity, and Learning in the Barn Owl. ILAR Journal, 51(4), 338-352. doi:10.1093/ilar.51.4.338
Quine, D. B., & Konishi, M. (1974). Absolute frequency discrimination in the barn owl. Journal of comparative physiology, 93(4), 347-360. doi:10.1007/BF00606802
Schwartzkopff, J. (1968). Structure and Function of the Ear and of the Auditory Brain Areas in Birds. In Ciba Foundation Symposium ‐ Hearing Mechanisms in Vertebrates (pp. 41-63).
Singheiser, M., Gutfreund, Y., & Wagner, H. (2012). The representation of sound localization cues in the barn owl's inferior colliculus. Frontiers in Neural Circuits, 6(45). doi:10.3389/fncir.2012.00045
Smith, C. A., Konishi, M., & Schuff, N. (1985). Structure of the Barn Owl's (Tyto alba) inner ear. Hearing Research, 17(3), 237-247. doi:10.1016/0378-5955(85)90068-1
Tanaka, K., & Smith, C. A. (1978). Structure of the chicken's inner ear: SEM and TEM study. American Journal of Anatomy, 153(2), 251-271. doi:10.1002/aja.1001530206
Trust, T. B. O. (n.d.). A Barn Owl's amazing powers of hearing. Retrieved from https://www.barnowltrust.org.uk/owl-facts-for-kids/barn-owl-hearing/
Volman, S. F. (1994). Directional Hearing in Owls: Neurobiology, Behaviour and Evolution. In M. N. O. Davies & P. R. Green (Eds.), Perception and Motor Control in Birds: An Ecological Approach (pp. 292-314). Berlin, Heidelberg: Springer Berlin Heidelberg. what-when-how. (n.d.). Burrowing Owl (Birds). Retrieved from https://what-when-how.com/birds/burrowing-owl-birds/