Comparative Analysis of the Biological Mechanics of Veins and Arteries in Animals
Akash Aniche, Hubert Laflamme, Deisha Paliwal, Julianna Raabel
Abstract
Outcomes of evolution, arteries and veins are dynamic vessels that serve to transport materials to the body's tissues and organs. This is both enabled and facilitated by the designs that endow the devices with this ability. This report introduces the concept of design and function regarding blood vessels, analyzed within the framework of biological mechanics and materials. The architectural components, operation, and function will be detailed, first through isolated analyses and then as complementary properties; the efficiency of the blood vessel system can be attributed to the intricate relationship between design and function. The consequences of a degradation of this relationship will be proposed. As well, a comparative study of the vessels in giraffes and Greenland sharks illustrates that, while evolution has established a design that optimizes the efficiency of function, these properties are not uniform across all organisms. Conversely, variants in the mechanics of architecture further equip organisms for the growth and survival conditions specific to their environments.
Architecture
The cardiovascular system comprises three macroscopic structures: the heart, blood vessels, and blood. Blood is pumped by the heart to arteries, which branch into arterioles with reduced diameters within organs. Arterioles carry blood to capillaries, which are exchange vessels with porous and thin walls. Capillaries converge into venules that unite to form veins to return blood to the heart. The responses of arteries and veins to the mechanical stress of pumping blood arise from the microscopic structures that compose it, chiefly, the three layers of the wall and the composite material formed by the underlying tissue's constituents.
Wall Layers
Arterial and venous walls comprise the same three layers: tunica intima, media, and adventitia. Tunica intima, the innermost layer of the vessel, contains one layer of elongated endothelial cells and derives support from a subendothelial layer with collagen (Taylor, 2020). Despite its functional importance, due to its thinness, the intima does not contribute considerably to the mechanical resistance of the vessel (Burton, 1954). The media consists of concentrically arranged smooth muscle embedded in an extracellular matrix of collagen and elastin (Xu and Shi, 2014). Elastin separates the media from the adventitia, the outermost layer that provides structural support through a dense network of collagen and elastic fibers (Taylor, 2020). A schematic of the layers of a coronary artery is illustrated in Fig. 1.
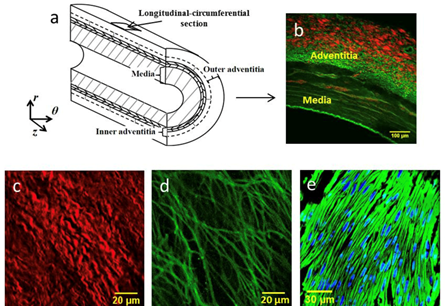
Although the basic structure of the wall is shared amongst vessels, the quantity and constituents of each tissue differ between arteries and veins. For a constant vessel diameter, a venous wall's thickness is approximately one third of an arterial wall (Basu et al., 2010) as illustrated in Fig. 2. The tunica media of arteries is thicker than veins, so arteries contain more smooth muscle to contract and higher quantities of elastin (Burton, 1954). The diameter of the arterial lumen is smaller compared to veins as displayed in Fig. 2. The presence of unidirectional valves in veins also distinguishes them from arteries. Venous valves are classified as bicuspid since they contain two elastic tissue flaps (Taylor, 2020).
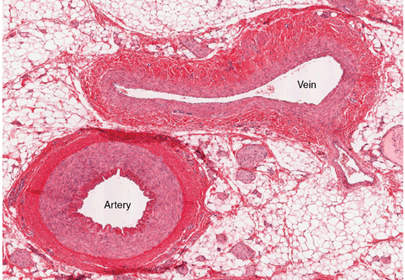
Elastin and Collagen
Elastin and collagen are the primary components of each layer, generating a composite material that dictates the mechanical behavior of vascular tissue. Elastin molecules form thin strands in vessels, as displayed in Fig. 3, capable of withstanding high strains without rupture when subject to low stress levels (Wagenseil and Mecham, 2012). Elastin is typically modelled as an isotropic material that provides recoil properties, compliance, and long-range elasticity to vessels. However, current studies postulate that it has unaccounted anisotropy at low strains (Xu and Shi, 2014). Elastic fibers serve as “entropic springs” that retain mechanical energy during the high pressure of systolic expansion and release it during the low pressure of diastolic relaxation (Peckham et al., n.d.).
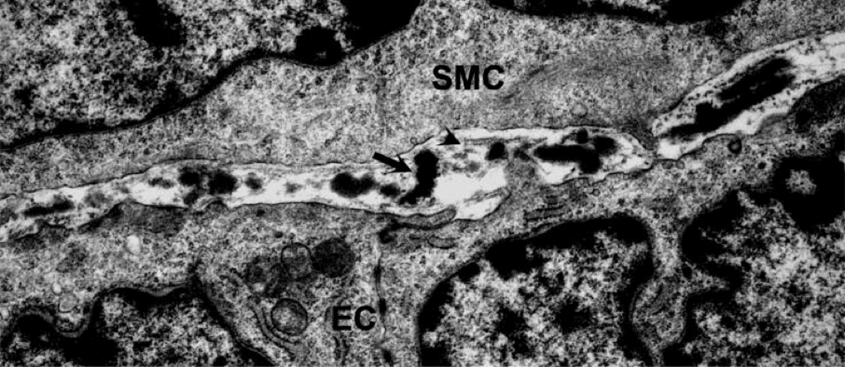
The network of collagen displays a hierarchical structure characterized by covalently bound molecules that form fibrils which aggregate as fibers. Collagen fibers in the adventitia display a higher angular dispersion and exist as thicker bundles than in the media where they are 7 co-aligned (Saitta Rezakhaniha, 2010). Fig. 4 displays the varying directions that the adventitia collagen fibers align themselves in. The load-bearing properties of vessel walls are derived primarily from collagen fibers, which render the walls anisotropic and result in nonlinear deformations (Saitta Rezakhaniha, 2010). Anisotropic materials exhibit varying physical properties when the axis of measurement is varied; correspondingly, vessels display more stiffness in the radial direction as compared to their longitudinal axes (Saitta Rezakhaniha, 2010).
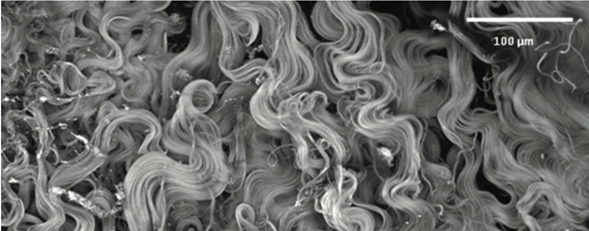
Blood vessels are viscoelastic, rather than elastic, so they assume the mechanical properties of both solids and liquids: resistance to deformation and the ability to flow, respectively. The degrees of linearity and isotropy can vary within and amongst veins and arteries. The collagen to elastin ratio is higher in veins than arteries, which presents consequences for the elastance and compliance of the vessels (Li, 2018). The presence of elastic fibers in arteries enables the wall recoil that is responsible for high arterial pressures (Li, 2018).
Arteries are further classified into two categories: elastic arteries and muscular arteries, whose structures are schematized in Fig. 5. Elastic arteries, including the aorta and carotid artery, are closer in proximity to the heart and have large diameters (Saitta Rezakhaniha, 2010). Conversely, muscular arteries, namely the external carotid, are farther from the heart with reduced diameters (Saitta Rezakhaniha, 2010). The proportion of collagen and elastin filaments in the tunica media of elastic arteries is higher than muscular arteries (Wagenseil and Mecham, 2012). Muscular arteries have a higher smooth muscle to elastin ratio, enabling their rapid vasoconstriction and dilation (Taylor, 2020).
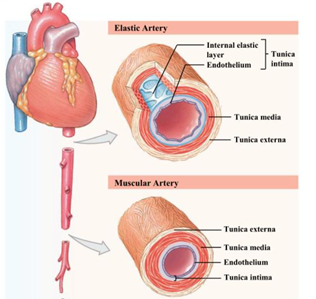
Function
The function of veins and arteries is to carry blood throughout the body to organs, transporting oxygen, cellular waste products, nutrients, and hormones. Arteries are responsible for carrying oxygenated blood away from the heart and to other organs, while veins carry blood back to the heart. Veins are under lower pressures and valves ensure the unidirectional flow of blood. The movement of blood flow is governed by forces exerted by blood vessels, namely shear forces and wall tension. These forces affect the flow of blood and the pressure inside vessels and can be modelled mathematically. Blood vessels are mechanically complex and dynamic, having the ability to dissipate and regulate pressure to meet the needs of the system. Mathematically modelling the pressure inside arteries demonstrates how forces exerted by blood vessels control the flow of blood. Close to the heart, the elastic walls of the arteries expand when blood is forced into them via a pressure pulse from the heart muscles. This pressure pulse propagates through arteries and can be modelled as a sinusoidal wave (Anliker et al., 1968). The recoiling of the artery maintains the pressure gradient and the flow of blood. As the blood moves further from the heart, the energy of the sinusoidal wave is dampened, as pictured in Fig. 6. As such, blood vessels must possess a mechanism by which they dissipate energy; this is accomplished through the interplay of various forces inside veins and arteries.
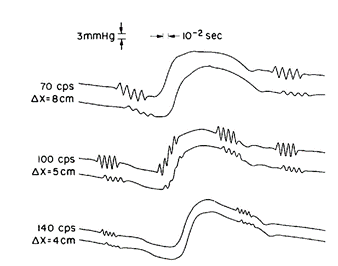
Force Analysis
An essential function of blood vessels is to transfer sufficient amounts of blood throughout the body. Blood vessels alter the resistance to blood flow by exerting shear stress, a form of frictional force. Paszkowiak and Dardik (2003) define shear stress as a frictional force that acts tangential to the direction of blood flow. Shear force is exerted on the endothelial surface of blood vessels. Approximating vessels as uniform, rigid cylinders, the shear stress can be derived from Poiseuille's Law,
\tau={4Q\eta\over (\pi r^3\ )}
(1) where Q is the rate of fluid flow and η the viscosity of the fluid (Milnor, 1980). This is a simplified model, since blood viscosity increases as its velocity increases, and vessels in fact are not uniform, rigid cylinders. However, this gives an approximation and is accurate under larger vessels, where the shear stress is high. It also highlights the relationships between rates of flow, the properties of the fluid, shear stress, and the structure of the vessel; the interaction of these properties greatly influences the function of blood vessels.
When vessels exert a high shear stress, blood flow is reasonably approximated as laminar flow, the smooth flow of a fluid in concentric, unmixing layers. Under such circumstances, vessels experience vasodilation, the widening of the vessel diameter (Levenson et al., 2001). This is the predominant physical state that blood vessels are in, as blood flows regularly and with a high momentum. The rate that the blood flows is proportional to the pressure gradient and inversely proportional to the resistance. Poiseuille's law for resistance establishes a relationship between the viscosity of a fluid, the architecture of the vessel, and the resistance to laminar flow. This equation is given by:
R={8ηl\over (πr^4 )}
(2)
where the fluid has viscosity η and the radius and length of the vessel are described by r and l, respectively (Ballermann et al., 1998). The ability of a vessel to resist motion of blood is a key function which is clearly dependent on the architecture of the vessel.
On the contrary, when shear stress is low or changing direction, the blood flow is turbulent; during turbulent flow, the velocity of a fluid is irregular and streamlines mix. Low shear stress promotes vasoconstriction, where vessels contract, reduce their diameter, and create pressure buildups. This is essential, as pressure must be reduced when the blood flows from the proximal to smaller, distal vessels. The significance of low wall shear stress is highlighted by studying its causal relationship with vascular diseases, particularly atherosclerosis. Atherosclerosis is the buildup of plaque in the artery walls, which can restrict blood flow and have life threatening consequences. The distribution of affected areas is shown to be mainly where the vascular tree of arteries is geometrically intricate (Katrisis et al., 2007). The more complicated the geometry of the arteries, the more irregular the blood flow and thus the lower the wall shear stress. To function properly, blood vessels must exert shear forces that change flow rates and resistance, controlling the quantity of blood reaching organs and ensuring the health of the circulatory system. Wall tension forces are another important force in vessels that are developed in the extracellular tissues and cytoskeletons of the cells in the wall tissues. Wall tensions can be derived using Laplace's Law for a cylindrical vessel, given as:
T=∆P×r
(3)
where ∆P is the transmural pressure gradient and r the radius of the vessel (Secomb, 2016). Transmural pressure is the pressure difference between the interior and exterior of the vessel. This highlights the relationship between the form and function of vessels, as the wall tension is directly proportional to the radius of the vessel. Wall tension and shear forces work together to mechanically alter blood flow, thus contributing to the function of veins and arteries.
Flow Direction
Blood vessels must ensure that blood is flowing in the correct direction throughout the body. This essential function is achieved through a pressure gradient and valves. Since arteries are under such high pressures, with the heart continuously forcing blood through them, the high-pressure gradient ensures that the blood flows away from the heart. Veins are under lower pressures and gravity resists the movement of blood from lower extremities back up to the heart, introducing the risk of blood flowing in the incorrect direction. Fortunately, the architecture of veins may ensure correct function via valves composed of two leaflets. Venous valves can have four states, each of which differently affects blood flow: opening, equilibrium, closing, and closed (Alimi et al., 1994). Pressure builds up behind the closed valve, pressing the leaflets of the valve open. The flow suspends the flaps of the valve in the open position, and they oscillate as the blood moves through it. Three streams of blood flow through the valve: the axial stream in the center of the valve and two lower speed streams called recirculation vortices (Bazigou and Makinen, 2013). This causes the area around the valve to distend. The equilibrium state occurs when the recirculation vortex pressure is in equilibrium with the pressures exerted from the axial stream. As the pressure beyond the valve surpasses the decreasing pressure approaching the valve, the leaflets of the valve close. The valve remains closed until the heart provides pressure, driving open the valve and repeating the process. This process ensures the unidirectional flow of blood through blood vessels.

Fractality of Vascular Trees
To ensure the proper delivery of nutrients and oxygen, each cell in the human body must maintain a proximity of 100 micrometers to a blood vessel or capillary. For that reason, blood vessels require fractal branching network to continuously subdivide and reach a smaller diameter, that of a capillary, which is about 5 to 10 micrometers (Sugden et al., 2017). This diameter is almost consistent across all mammals, independent of body size. Blood vessels formation, or angiogenesis, can be conceptualized in a similar way to the growth of a tree-like structure. That is, a sprout forms a smaller branch from a main stem. It is 15 important to note that blood vessels do not have perfectly uniform structures, and there is a certain degree of asymmetry in each subdivision of the “vascular tree”.
However, because of the same branching pattern that is continuous throughout, and the observation that each main stem almost always branches out into exactly two new daughter vessels, the vascular tree can be considered to have “pseudo-fractal” properties (Zamir, 1999). The basic unit of the vascular tree is thus a “Y”-shaped junction (Takahashi, 2014). However, angiogenesis is particular in that vessels cannot grow outwards in an undefined manner as they must form capillaries; arteries and veins must interconnect. Consequentially, the circulatory system can be considered as being comprised of two different vascular trees: arterial and venous, connecting at their tips. In early embryonic development, in large vascular beds, blood vessels generate due to the simultaneous sprouting of vessels. They form an interwoven network called an “immature vascular plexus” (Red-Horse and Siekmann, 2019). This structure then continues to grow and is remodeled into arteries, veins, and capillaries due to the mechanical forces exerted by blood flow, mainly shear stress and wall tension. These forces also ensure proper vessel diameter within the hierarchical structure.
Sprouting can occur in two ways. New sprouts can emerge from pre-existing arteries and veins in a non-specified, arteriovenous identity and later interconnect and interconvert themselves – re-specification into arteries or veins – to ensure proper circulation of blood. However, recent studies have shown that this remodeling often does not happen as expected as in a branching morphogenesis. It is not always a bottom-up branching-out pattern, but sometimes arterial growth is reversed and occurs in a top-down pattern. In the latter stages of embryonic development and during tissue regeneration, new vessels often sprout only from veins and not from arteries. These sprouts then progress towards existing arteries where they are reprogrammed into arteries once 16 blood flow is established. The migration of endothelial cells, in this case, happens against the blood flow as pictured in Fig. 8. This avoids the leakage of blood that is more likely to occur if the sprout emerges from an artery because of the high pressure (Red-Horse and Siekmann, 2019).
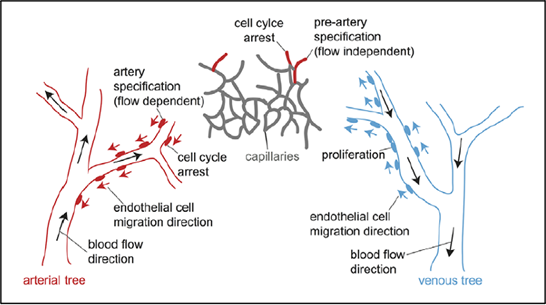
Deteriorations to Architecture and Function
Evidence for the argument of blood vessel architecture as a means of fulfilling biological function can be found where the architecture deteriorates; the biomechanics of arterial stiffening, aneurysms and venous diseases illustrate this notion. Arterial Stiffening Changes to the design and operation of elastin and collagen cause arteries to stiffen as a person ages. Elastin in the tunica media sustains fragmentation, causing mechanical load to be transferred to collagen, which is stronger and less flexible (Kohn et al., 2015). This causes an increase in the velocity of pulse waves, corresponding to higher systolic and pulse pressures along with a lower distensibility (Reesink and Spronck, 2019) as displayed in Fig. 9. Elastic fragmentation occurs primarily in elastic arteries with large stretch amplitudes rather than in muscular arteries, and results in a loss of compliance to the vessel (Segers et al., 2019). Aneurysms Weaknesses in arterial walls result in the distension that characterizes an aneurysm. Following the formation of an aneurysm, blood exerts continuous pressure and shear stress on the vessel wall, which can lead to an enlargement (Lasheras, 2007). Arterial rupture occurs when the wall's ability to withstand the forces exerted by the pulsatile flow is exceeded, resulting in death or severe damage (Lasheras, 2007). The consequences of an aneurysm, often fatal, demonstrate that the vessel can no longer satisfy its function when the structure that endows it with its biomechanical properties is altered (Lasheras, 2007).
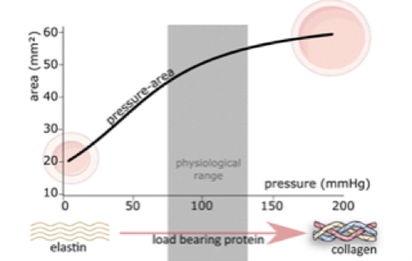
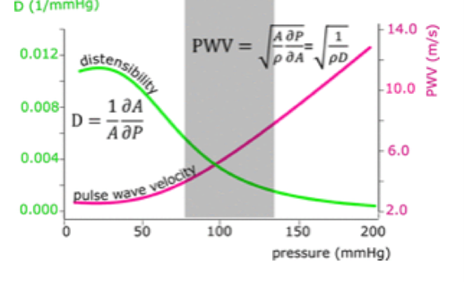
Venous Diseases
Vein mechanics depend on their layered tissue structure; a modification in this structure can lead to venous diseases. There are generally two classes of diseases that affect veins: venous thrombosis, which is the result of the formation of a blood clot, and venous insufficiency, which is characterized by an improper blood return to the heart. Venous thrombosis occurs when a blood clot is created in either superficial veins or deep within the legs. In response to the decreased efficiency of blood return, and the damaging increase in shear stress, veins undergo a change in their material and mechanical properties. The stiffness of collagen and elastin fibers within the venous tissue is increased, and consequentially, the distensibility of the vessel in response to pressure changes declines. The vessel wall thickness also changes as it is increased by a factor of about 1.5 to 1.8. These fundamental changes in the structure of the vessel seem to be enduring (Vekilov and Grande-Allen, 2018).
Venous insufficiency, which leads to varicose veins, originates from an obstruction in a vein or by leaking venous valves that allow backflow of blood. This results in an increased blood pressure within the vessel walls and also leads to a change in their structural properties. Each layer adapts a disorganized structure, their respective thicknesses change, collagen fiber deposits form, and their overall elasticity is diminished (Vekilov and Grande-Allen, 2018). The changes that occur further reduce the ability of the vessel to pump blood back to the heart. Venous diseases are thus a result of the alteration of the design of veins that hinder their ability to function correctly.
Operation
Veins and arteries are viscoelastic in nature, suiting their function well, and they must adapt to different situations. However, arteries and veins operate under different transmural pressures, explaining the difference in the rigidity of their material composition. Arteries are under the high systolic pressure generated from the heart when it contracts, and under low diastolic pressure when the heart is in between beats. Thus, they require thick, muscular walls to withstand this higher pressure without bursting, but they also need to be elastic to release energy during lower pressure. The healthy systolic and diastolic pressures are 120 and 80 millimeters of mercury, respectively (“High Blood Pressure/Hypertension” | Johns Hopkins Medicine, n.d.). This is often written as the fraction 120/80 mmHg, with the numerator representing the systolic pressure and the denominator being the diastolic pressure. On the contrary, veins are subjected to lower pressures as the wave has already propagated through the arteries and dissipated much of its energy. This gap between high and low pressure illustrates how the form and function of blood vessels are interrelated: the elastic material structure of blood vessels is essential for the lowering of pressure and for the smooth transition of blood from large vessels to small vessels which would rupture under such high pressures.
Compliance
The elasticity of blood vessels can be examined using measures of volume distensibility. Most commonly, this is done using compliance, defined as the relationship between a minute change in volume relative to a minute change in pressure (Boulpaep, 2016):
C=∆V/∆P
(4)
Compliance is a measure of how easily the volume of a vessel can be increased. Fig. 10 graphs the relative volume percentage against the transmural pressure; the compliance is given by the slope of the tangent line. Arteries have stiffer walls which resist changes in volume, and thus have a lower compliance than veins. The steep tangent slope demonstrates the high compliance of veins while under normal physiological pressures, which allows them to act as “capacitors” of the circulatory system and store high volumes of blood.
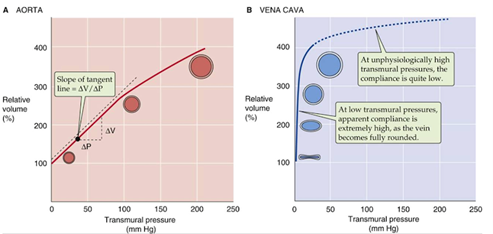
Neural Regulation
The smooth muscle composition inside arteries allows for contraction and expansion, essential to regulating under various stimuli. The nerves in the smooth muscle use sympathetic and parasympathetic pathways to regulate blood flow. When under emotional stress, arteries and arterioles use sympathetic pathways to increase the resistance in the vessels, thereby decreasing the blood flow to particular areas (Klabunde, “Neural Activation of the Heart and Blood Vessels”). A “fight or flight” response induces changes in the hormones adrenaline and noradrenaline, which increases the resistance in the vessel, decreasing blood flow to distal areas. In turn, cardiac activity increases to compensate. While exercising, a similar mechanism is followed, which increases the heart rate. In extreme circumstances, blood vessels may even alter their structure if their function requires it; the capillary system in people who exercise regularly is more intricate and composed of more capillaries than those who do not (“Exercise and your arteries” | Harvard Health Publishing | Harvard Medical School, 2019).
Parasympathetic pathways are used to “relax and digest”, meaning that the blood pressure returns to a normal level and the heart rate slows. The hormone acetylcholine triggers this response (Thomas and Segal, 2004). While exercising or experiencing emotional extremes, blood vessels lack parasympathetic effects and are controlled by sympathetic reactions. Parasympathetic intervention allows vessels to return to homeostasis. When the body requires a change to be made due to hormonal changes or stimuli, blood vessels adapt to meet its needs. To ensure the optimal operation and of blood vessels, hormones trigger neural regulation responses.
Applications in Animals
Humans
The effect of gravity on the cardiovascular system is non-negligible, and it is the reason behind the phenomenon that is experienced when lying down and standing up quickly after. This movement often results in dizziness and a brief loss of vision. When a person is lying down, gravitational forces are consistent across the thorax, abdomen, and legs since these components exist in the same horizontal plane (Klabunde, “Effects of Gravity on Venous Return”). As a result, pressure on the veins is consistent throughout the body. However, when a person suddenly stands up, the force of gravity causes the blood to accumulate in the lower extremities, which increases the blood pressure in the veins of the legs and feet. This increase causes the veins to expand to pump this sudden pool of blood back to the heart. Thus, there is a very large volume of blood being moved in the veins of the legs, but during this time, there is a very small volume of blood being moved in the veins and arteries of the upper body. As a result, the arterial pressure of the upper body is decreased greatly. The fall in arterial pressure reduces cerebral blood flow to the point of loss of motor abilities, or more severely, the person can experience syncope, also known as fainting (Klabunde, “Effects of Gravity on Venous Return”). Once the blood that had accumulated in the legs returns to the heart, the blood pressures recalibrate throughout the body. However, for the brief period during which a person stands up very quickly, the pressures are unequal, and the aforementioned behaviors can result. Fig. 11 displays the differences in venous volume and venous pressure in the legs and thorax of a person in the supine and upright positions.
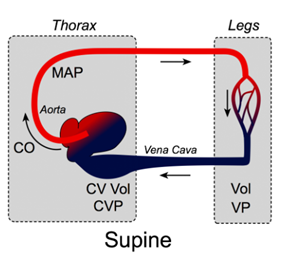
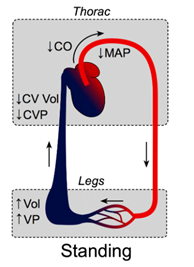
Giraffes
Giraffes present a compelling vascular system for analysis since their vessels confront internal pressures that humans and most animals do not experience. The main reason for this duress derives from the fact that a giraffe's head operates at a higher altitude. This equips them with an advantage for feeding and surveilling their surroundings. Nonetheless, a head that is distant from their heart presents a confounding anatomical issue: how can blood arrive at the brain when it is so far above the heart? By comparison, the human heart only has to pump blood along the arteries for a couple inches before it arrives to the brain so the flow of blood must have enough initial velocity to combat gravity for a short distance. Nevertheless, the blood flow of giraffes must combat the force of gravity over meters. From an engineering approach, the solution to this problem appears intuitive: increase the velocity that the heart pumps blood with so the blood can reach the brain. This is consistent with what occurs in giraffes; correspondingly, they exhibit higher blood pressures compared to other animals. In fact, studies have shown that giraffes have blood pressures of approximately 300/180 mm Hg, which is twice the blood pressure of humans and most animals (Lawrence, 2015). As a result, arteries, specifically those that run up the neck, must confront high pressures, so giraffes have adapted the mechanical properties of their arteries in consequence. Their neck arteries are highly elastic and muscular, to allow for stretching without rupturing when the blood arrives from the heart, as displayed in Fig. 12. Once the blood arrives at the brain, giraffes are equipped with a network of small, elastic blood vessels that can alter form to adapt to the position of the head. Thus, when the head is upright and at a distance of a few meters above the heart, the vessels constrict so that the blood does not flow too fast, which allows the brain to retain blood for a longer period of time before the next heartbeat (Baptista et al., 2019).
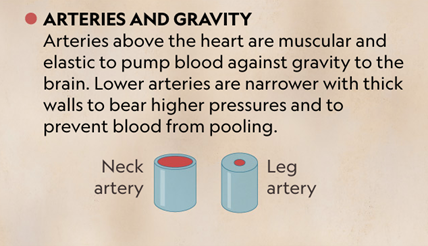
It is relevant to note that a giraffe's long neck also allows it to smell and eat what is on the ground while still standing, activities pertinent to their growth and survival. Thus, in a fraction of a second, their head can relocate from a few meters above their heart to a few meters below their heart. If humans were equipped with the same ability, they would instantly faint due to an extreme rush of blood towards their head. However, giraffes have developed an adaptation for this issue in the form of valves in veins above the heart. These valves only allow the unidirectional flow of blood from the head to the heart, so when a giraffe lowers its head, the brain is not confronted with the blood volume contained in the two-meter-long neck (Mitchell et al., 2009). Additionally, the network of small vessels near the brain that can alter form, expand when the giraffe lowers its head, enabling a faster flow of blood so it does not accumulate near the brain (Baptista et al., 2019). Fig. 13 offers a representation of the functioning of the valves, and Fig. 14 demonstrates a closed valve when the giraffe's head is lowered.

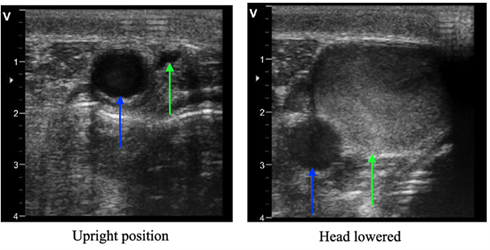
Humans, however, are not structurally equipped like giraffes to allow for a blood flow in veins returning to the brain instead of the heart. In fact, humans only have one valve between the heart and the brain, as compared to several valves in giraffes (Mitchell et al., 2009). This provides a rationale for why standing on one's head is an uncomfortable experience; there is an insufficient number of valves to inhibit the blood in the neck from returning to the brain, so it accumulates progressively. Since the human neck is not two meters long, the amount of blood is not significant; fainting is not an instant consequence of standing on one's head, but the amount of blood is considerable enough to render it uncomfortable. Hence, the adaptations giraffes have developed in their neck arteries and veins enable them to combat the changing arterial pressures and blood flow, allowing them to experience the full range of motion of their head without collapsing.
In addition to their long necks, giraffes have long legs, which, in turn, presents another problem: returning the blood back to the heart. With their long legs, if the heart sends an excess of blood at the same time in the arteries, it will begin to accumulate in the legs since veins will struggle to return the blood back to the heart. Indeed, in this case, the veins must work against gravity to bring the blood back to the heart, while the arteries have gravity working in their favor to bring the blood from the heart down to the legs. Thus, to limit the flow of blood down the legs, giraffes' arteries below their hearts have reduced diameters to limit the amount of blood that reaches the legs at the same time (Petersen et al., 2013). However, the heart pumps the blood with the same force as it would if the arteries were of a normal diameter so the pressure inside the smaller arteries is very high, which is why leg arteries are equipped with thicker walls (Baptista et al., 2019). Thus, as displayed in Fig. 15, giraffes have elastic neck arteries with thin vascular wall and large diameters, while their leg arteries are thick and rigid with reduced diameters.
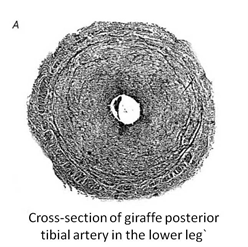
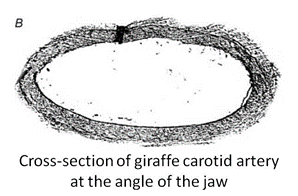
The reduced diameter of the leg arteries allows the high-pressure blood flow to maintain its momentum when it transfers into the veins. This way, the blood arrives in the veins with a high velocity to fight against gravity. Like humans, a giraffe's leg veins are also equipped with valves that prevent the back flow of blood. Thus, the sizes and structure of the components of veins and arteries in giraffes' legs prevent the accumulation of blood and enable active lifestyles, representing one extreme of the animal spectrum's vascular system.
Greenland Sharks
On the other end of the spectrum, many species of sharks have relatively low blood pressures compared to humans and giraffes. In lower vertebrates, such as fish and amphibians, blood pressure is associated with metabolic rate and the level of aerobic activity; higher blood pressure corresponds to a more active lifestyle. The Greenland shark is a slow-moving species that inhabits the low-temperature, high-depth waters of the North Atlantic Ocean (Bates, 2016). In its ventral aorta, it has an approximate average blood pressure of 2.3 to 2.8 kPa or 17.25 to 21 mmHg (Shadwick et al., 2018). To ensure proper blood delivery in its large body size, which is around five meters, with its extremely low blood pressure, Greenland sharks' blood vessels have developed an adaptation in the organization of their fibrous connective tissues. They must be able to inflate to a higher extent at lower pressures than other species. The primary composition – elastin, collagen, and smooth muscle cells – and the organization of the three layers – tunica intima, media, adventitia – is consistent with other organisms. However, in the tunica media, elastin fibers and smooth muscle cells are oriented parallel to the vessel's axis longitudinally in the region close to the tunica intima, and circumferentially in the region close to the tunica adventitia as displayed in Fig. 16. This arrangement equips the Greenland shark's arteries with a resistance to stretching when exposed to high pressures (Shadwick et al., 2018). The elastin fibers are also more loosely connected than in other species. The total area covered by the fibers is roughly half of what is considered typical for mammals. This dispersion of the fibers allows for more distensibility at lower blood pressure than in mammals, which is essential for the adequate functioning of the vessels of the Greenland shark in delivering blood throughout its body (Shadwick et al., 2018).
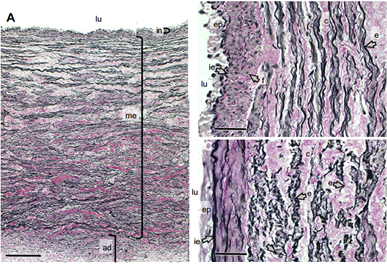
Conclusion
Veins and arteries are dynamic organs that function as the transportation mechanisms for oxygen, nutrients, and hormones in the cardiovascular system. The mechanical responses of arteries and veins to the forces that blood exerts on them arise from the microscopic structures that compose the vessels, including the layers of the vascular wall and the composite material formed by the underlying tissue's constituents. The architecture of these properties varies within and amongst veins and arteries, suited to the function they perform in the cardiovascular system. Blood vessels experience shear and transmural pressures. Together, these hemodynamic cues influence the diameter of the vessel and the rate of blood flow, thus ensuring the correct amount of blood reaches organs (Secomb, 2016). Arteries are under high pressure, which maintains the direction of blood flow. Unidirectional flow in veins is attributed to the presence of venous valves whose states are controlled by pressure changes. Veins and arteries are designed structurally, in a hierarchical pattern, such that their form allows for prime function. When the structure is flawed, or when an alteration occurs in their mechanical and material properties, the function is unable to proceed properly such as in the cases of arterial stiffening, aneurysms and venous diseases. Conversely, when structure and function work together efficiently, blood vessels operate smoothly. The viscoelastic composition of blood vessels influences their distensibility and ability to withstand pressure. The mechanical properties of vascular systems vary according to the survival and growth needs of an organism as illustrated by the architectural adaptations of giraffes and Greenland sharks that allow them to pursue the lifestyles specific to the environments they occupy. Viewing vessels as devices, while approaching their mechanics from an evolutionary lens, clarifies the relationship between design and function as mutually dependent, rather than exclusive, properties of biological systems.
References
Alimi, Y. S., Barthelemy, P., & Juhan, C. (1994). Venous pump of the calf: a study of venous and muscular pressures. Journal of Vascular Surgery, 20(5), 728-735. doi:10.1016/s0741-5214(94)70160-1
Anliker, M., Histand, M. B., & Ogden, E. (1968). Dispersion and attenuation of small artificial pressure waves in the canine aorta. Circulation Research, 23(4), 539-551. doi:10.1161/01.res.23.4.539
Ballermann, B. J., Dardik, A., Eng, E., & Liu, A. (1998). Shear stress and the endothelium. Kidney International, 54, S100-S108. doi:10.1046/j.1523-1755.1998.06720.x
Baptista, F. G., Maggiacomo, T., Conant, E., & Walljasper, S. (2019). Unique anatomy sets giraffe apart in the animal kingdom. National Geographic (October 2019 ). Retrieved from https://www.nationalgeographic.com/animals/article/graphic-shows-giraffes-unique-evolution-traits#close
Basu, P., Sen, U., Tyagi, N., & Tyagi, S. C. (2010). Blood flow interplays with elastin: collagen and MMP: TIMP ratios to maintain healthy vascular structure and function. Vascular health and risk management, 6, 215-228. doi:10.2147/vhrm.s9472
Bates, M. (2016). 272-Year-Old Shark Is Longest-Lived Vertebrate on Earth. Retrieved from https://www.nationalgeographic.com/animals/article/greenland-sharks-animals-science-age
Bazigou, E., & Makinen, T. (2013). Flow control in our vessels: vascular valves make sure there is no way back. Cell Mol Life Sci, 70(6), 1055-1066. doi:10.1007/s00018-012-1110-6
Boulpaep, E. L. (2016). Arteries and Veins. In Medical Physiology (3 ed., pp. 447-460): Elsevier, Inc.
Brøndum, E., Hasenkam, J. M., Secher, N. H., Bertelsen, M. F., Grøndahl, C., Petersen, K. K., . . . Wang, T. (2009). Jugular venous pooling during lowering of the head affects blood pressure of the anesthetized giraffe. Am J Physiol Regul Integr Comp Physiol, 297(4), R1058-1065. doi:10.1152/ajpregu.90804.2008
Burton, A. C. (1954). Relation of structure to function of the tissues of the wall of blood vessels. Physiological Reviews, 34(4), 619-642. doi:10.1152/physrev.1954.34.4.619
Chen, H., & Kassab, G. S. (2016). Microstructure-based biomechanics of coronary arteries in health and disease. Journal of Biomechanics, 49(12), 2548-2559. doi:10.1016/j.jbiomech.2016.03.023
Katritsis, D., Kaiktsis, L., Chaniotis, A., Pantos, J., Efstathopoulos, E. P., & Marmarelis, V. (2007). Wall Shear Stress: Theoretical Considerations and Methods of Measurement. Special Articles, 49(5), 307-329. doi:10.1016/j.pcad.2006.11.001
Klabunde, R. E. (2014, 23 Apr 2014). Neural Activation of the Heart and Blood Vessels. Retrieved from https://www.cvphysiology.com/Blood%20Pressure/BP009
Klabunde, R. E. (2016, 7 Dec 2016). Effects of Gravity on Venous Return. Retrieved from https://www.cvphysiology.com/Cardiac%20Function/CF017
Kohn, J. C., Lampi, M. C., & Reinhart-King, C. A. (2015). Age-related vascular stiffening: causes and consequences. Frontiers in Genetics, 6(112). doi:10.3389/fgene.2015.00112
Lasheras, J. C. (2007). The Biomechanics of Arterial Aneurysms. Annual Review of Fluid Mechanics, 39(1), 293-319. doi:10.1146/annurev.fluid.39.050905.110128
Lawrence, E. (2015). Giraffes have high blood pressure. Why don't they drop dead? In. novartis.com. Retrieved from https://www.novartis.com/stories/from-our-labs/giraffes-have-high-blood-pressure-why-dont-they-drop-dead
Levenson, J., Pessana, F., Gariepy, J., Armentano, R., & Simon, A. (2001). Gender differences in wall shear-mediated brachial artery vasoconstriction and vasodilation. Journal of the American College of Cardiology, 38(6), 1668-1674. doi:10.1016/s0735-1097(01)01604-7
Li, W. (2018). Biomechanical property and modelling of venous wall. Progress in Biophysics and Molecular Biology, 133, 56-75. doi:10.1016/j.pbiomolbio.2017.11.004
Medicine, J. H. (n.d.). High Blood Pressure/Hypertension. Retrieved from https://www.hopkinsmedicine.org/health/conditions-and-diseases/high-blood-pressure-hypertension
Milnor, W. R. (1980). Principles of hemodynamics. In V. Mountcastle (Ed.), Medical Physiology (14 ed.). St Louis CV Mosby Co.
Mitchell, G., van Sittert, S., & Skinner, J. (2009). The Structure and Function of Giraffe Jugular Vein Valves. South African Journal of Wildlife Research, 39, 175-180. doi:10.3957/056.039.0210
Paszkowiak, J. J., & Dardik, A. (2003). Arterial wall shear stress: observations from the bench to the bedside. Vascular and Endovascular Surgery, 37(1), 47-57. doi:10.1177/153857440303700107
Peckham, M., Knibbs, A., & Paxton, S. (n.d.). Arteries. Retrieved from https://www.histology.leeds.ac.uk/circulatory/arteries.php
Petersen, K. K., Hørlyck, A., Østergaard, K. H., Andresen, J., Broegger, T., Skovgaard, N., . . . Aalkjaer, C. (2013). Protection against high intravascular pressure in giraffe legs. American Journal of Physiology-Regulatory, Integrative and Comparative Physiology, 305(9), R1021-R1030. doi:10.1152/ajpregu.00025.2013
Red-Horse, K., & Siekmann, A. F. (2019). Veins and Arteries Build Hierarchical Branching Patterns Differently: Bottom-Up versus Top-Down. Bioessays, 41(3), 1800198. doi:https://doi.org/10.1002/bies.201800198
Reed, L. (2010, 19 Sep 2010). Pressure and Gravity. Retrieved from https://medicineoutofthebox.com/2010/09/19/pressure-and-gravity/
Reesink, K. D., & Spronck, B. (2019). Constitutive interpretation of arterial stiffness in clinical studies: a methodological review. Am J Physiol Heart Circ Physiol, 316(3), H693-h709. doi:10.1152/ajpheart.00388.2018
Saitta Rezakhaniha, R. (2010). Biomechanics of Vascular Wall: the Role of Structural Organization of Elastin and Collagen. doi:10.5075/epfl-thesis-4712
School, H. M. (2019, 29 Jun 2019). Exercise and your arteries. Retrieved from https://www.health.harvard.edu/heart-health/exercise-and-your-arteries
Secomb, T. W. Hemodynamics. In Comprehensive Physiology (pp. 975-1003). doi: https://doi.org/10.1002/cphy.c150038
Segers, P., Rietzschel, E. R., & Chirinos, J. A. (2020). How to Measure Arterial Stiffness in Humans. Arteriosclerosis, Thrombosis, and Vascular Biology, 40(5), 1034-1043. doi:doi:10.1161/ATVBAHA.119.313132
Shadwick, R. E., Bernal, D., Bushnell, P. G., & Steffensen, J. F. (2018). Blood pressure in the Greenland shark as estimated from ventral aortic elasticity. Journal of Experimental Biology, 221(19). doi:10.1242/jeb.186957
Sugden, W. W., Meissner, R., Aegerter-Wilmsen, T., Tsaryk, R., Leonard, E. V., Bussmann, J., . . . Siekmann, A. F. (2017). Endoglin controls blood vessel diameter through endothelial cell shape changes in response to haemodynamic cues. Nature Cell Biology, 19(6), 653-665. doi:10.1038/ncb3528
Takahashi, T. (2014). Branching Systems of Fractal Vascular Trees. In Microcirculation in Fractal Branching Networks (pp. 1-24). Retrieved from https://www.springer.com/gp/book/9784431545071
Taylor, T. (2020, 29 Jul 2020). Cardiovascular System. Retrieved from https://www.innerbody.com/image/cardov.html
Thomas, G. D., & Segal, S. S. (2004). Neural control of muscle blood flow during exercise. Journal of Applied Physiology, 97(2), 731-738. doi:10.1152/japplphysiol.00076.2004
Vekilov, D. P., & Grande-Allen, K. J. (2018). Mechanical Properties of Diseased Veins. Methodist Debakey Cardiovascular Journal, 14(3), 182-187. doi:10.14797/mdcj-14-3-182
Wagenseil, J. E., & Mecham, R. P. (2012). Elastin in large artery stiffness and hypertension. Journal of Cardiovascular Translational Research, 5(3), 264-273. doi:10.1007/s12265-012-9349-8
Xu, J., & Shi, G.-P. (2014). Vascular wall extracellular matrix proteins and vascular diseases. Biochimica et Biophysica Acta, 1842(11), 2106-2119. doi:10.1016/j.bbadis.2014.07.008
Zamir, M. (1999). On fractal properties of arterial trees. Journal of Theoretical Biology, 197(4), 517-526. doi:10.1006/jtbi.1998.0892