Biological Design for Lungs and Gills: Information and Storage Processing
Samuel Bernard, Nabhaan Farooqi, Camille Gagnon, Will Vyse
Abstract
This report describes two of the most important gas exchangers for all living animals: lungs and gills. Throughout the research, cutaneous respiration will also be explained; however, the most efficient oxygen diffusion rates are obtained through lungs and gills, which are our main concern. The purpose of this biological design project is to find out how different species evolved and developed unique respiration systems that allow them to maximize their oxygen intake without spending energy. This report contains information about lungs and gills, basic respiration regulation, how respiration homeostasis is maintained throughout the organisms no matter the environment they are in, and the respiratory rhythm center that responds to specific stimuli by innervating the organism's cranial nerves and effectors.
Gills Respiration Regulation
Counter-Current System
Water-breathing animals may consume oxygen as water flows, for fish in general, into their mouths and through their gill filaments allowing the oxygen to diffuse to the bloodstream using the counter-current system (Spencer, 2020). This physical property makes use of the gradient of concentration between oxygen in the bloodstream and oxygen in the water. As its name suggests, the counter-current system occurs when the bloodstream flows in one direction and water over the gill flows in the other. This allows the highly oxygen concentrated blood to leave the capillaries in the gills, making place for venous blood, lowering the oxygen concentration in the capillary. Since both liquids circulate in opposite directions, the deoxygenated blood makes indirect contact with oxygen-filled water passing through the gills, this unbalanced gradient of concentration starts the diffusion (“An Efficient Exchange: countercurrent oxygen exchange in fish” | Fishbio, 2015).

Pumps
Muscle Pumps
To push freshwater over their gills and out of their system through slits on both sides of the pharynx in a unidirectional flow, bony and cartilaginous fish must possess muscle pumps. Both species are equipped with buccal pumps. Whereas the bony fish, called teleost, also possesses an opercular pump, the elasmobranch, cartilaginous fish, has an analogous septal pump (Taylor, 2011).
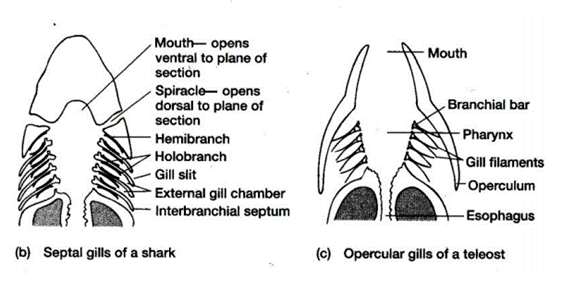
Opercular Pump (Bony Fish/Teleosts)
In both types of fish, the buccal pump allows water to enter the buccal cavity through the mouth. The pumping process is then separated into two phases: the suction phase and the pressure phase. During the suction phase, both the buccal and opercular cavities drop their pressure by expanding their volume allowing water from the exterior to enter their mouth and flow into these cavities. The opercular cavity has a slightly more negative pressure allowing water to go through the gill's filaments as water always follows the gradient of pressure (Lumb, 2017). The operculum located over the teleosts gill's as a means of protection also has an important role in gill ventilation. As the pressure lowers in both cavities, the opercular flaps close. Once the maximum volume is reached, the buccal and opercular muscles contract and compress both cavities, increasing the pressure inside drastically. The pressure then pops open the opercular flaps on both sides of the fish allowing the deoxygenated water to return to the exterior (Milsom, n.d.).
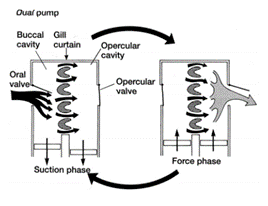
Septal Pump (Cartilaginous Fish/ Elasmobranch)
Contrary to the bony fish, the elasmobranch does not have operculums that protect the gills and therefore no opercular cavities. They are equipped however with spiracles and parabranchial cavities. Their gill ventilation also follows the same process as teleosts, a suction and pressure phase, also called inhalation and exhalation. During inhalation, the buccal cavity expands, lowering its pressure, allowing water to enter from its mouth and spiracle. As soon as the water starts to accumulate inside the buccal chamber, the parabranchial cavities expand, now lowering the pressure. While its volume inside the cavities increases, the flaps remain closed. Same as for the bony fish, water flows through the gills following the gradient of pressure. As water enters the parabranchial chamber, the septal and buccal muscles contract, opening the flaps and compressing the water out of the gills (Marshall and Hughes, 1965). As gill ventilation can be metabolically expensive, some elasmobranchs, notably sharks, will rely on ram ventilation. This type of respiration relies on the shark swimming forward with its mouth open, allowing water to enter and flow through the gills without using their jaw muscles (Milsom, n.d.).
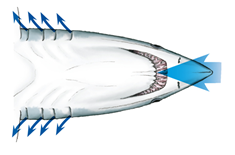
Respiratory Rhythm Generator (RRG)
For the gills to ventilate properly, their muscle pumps are innervated by four different cranial nerves: the Vth, the VIIth, the IXth, and the Xth. They will be explained in more detail further along the essay. The respiratory rhythm generator is located in the central nervous system (CNS), its main function is to send electric signals to the muscles that allow respiration (“21.10A: Neural Mechanisms (Respiratory Center)” | Medicine LibreTexts, 2020). This type of system is universal, meaning that mammals also possess a similar RRG, this basic respiratory system dates back to the first multicellular animals. Although researchers have discovered that the RRG is located in the brainstem for almost all animals, experiments are still conducted to know exactly where. Techniques for marking, such as micro lesions and dye injections, have however proved that the respiratory rhythm center is located in the medulla oblongata. Furthermore, these studies have shown that the respiratory rhythm center appears as “longitudinal strips of nervous tissues with respiration related neurons, on either side of the medulla” (Ballintijn, 1982). The cranial nerves mentioned above, the trigeminal nucleus, and the reticular formation (RF) compose the strips. All the cranial nerves (motor nuclei) receive afferent input or information from the trigeminal nucleus, they also send efferent and receive afferent information to the RF. Supposedly one site of the rhythm generator would start to form in the RF. That being said, for mammals, the rhythm generator that composes the RF is only active during the waking state. Studies have been conducted and discovered that even during anesthesia, the fish's rhythm generator seemed to be fully operational, which means that their rhythm generator may be able to rely on input coming from other parts in the CNS. Some sites have yet to be discovered (Taylor et al., 2010).
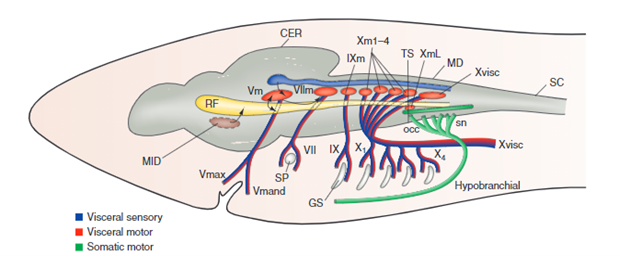
Cranial Nerves
The four dorsal cranial nerves mentioned above innervate the muscles around the jaws. These nerves come from the brainstem, they are composed of efferent cell bodies and afferent sensory projections. This allows the nerves to innervate mechanoreceptors and chemoreceptors on the gills, that detect changes in the environment around the fish (Tavighi et al., 2016). Their afferent sensory projections allow them to send input to the respiratory rhythm in the RF. The Vth trigeminal nerve innervates the muscles that are located in the mouth, the jaw-closing muscles. The VIIth facial nerve acts on the branchial muscles of the hyoid arch, and for elasmobranchs, innervates the spiracle, a small flap that allows water to enter the buccal cavity during inhalation. For the teleost, the facial nerve controls the operculum, opercular flaps. Both the IXth and Xth nerves, glossopharyngeal and vagus nerves, also called branchial nerves, innervate the mechanoreceptors and chemoreceptors. Furthermore, these nerves control the muscles in the gills arches, holding the gill arches and gill lamellae's curtain in place (Tavighi et al., 2016).
Hypoxia
Whereas air is composed of around 21 % oxygen, water only 1 %, which means that fish have a much more efficient respiratory system than other animals as they are still able to extract enough oxygen to function (“Dissolved Oxygen” | Water on the Web, 2017). What happens however when that small percentage drops even lower? This process is called hypoxia, when water does not have enough dissolved oxygen. Compared to other mammals who would probably die quite easily in such environments, fish must encounter hypoxia on a daily basis. The gill's chemoreceptors are sensitive to changes in water composition involving oxygen. As soon as they sense a decrease in oxygen, they are innervated by both the IXth and X nerves that send afferent input to the RF acting on the respiratory rhythm generator (Ballintijn, 1982). For elasmobranchs, the chemoreceptors are also located on the spiracle. On one hand, for water-breathing fish, the usual response is to increase ventilation. On the other, some fish will rely on their anaerobic metabolism since ventilation requires a lot of energy. However, air-breathing fish usually lower their gill ventilation and come up to the surface to only rely on their lungs (Taylor et al., 2010).
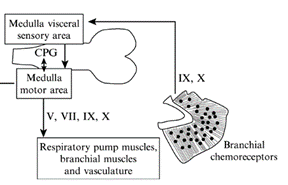
Ionic Composition and pH Variations
Involving pH changes in the water or ionic composition variations, fish cannot, compared to mammals who can modify their respiration, change their ventilation as a way to maintain homeostasis. The percentage of oxygen dissolved in water is too small for fish to be able to drastically modify their ventilation to regulate their inner pH or ionic composition. Instead, they rely on their cellular mechanisms in their gills (Pritchard, 2003). Usually, all the ions that are lost in their urine or through diffusion in their gills, they manage to retrieve in their gill epithelium during inhalation, or through food.
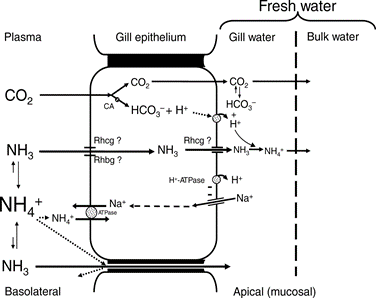
Pollution
When it comes to pollution, most fish have a protective layer of mucus that surrounds their epithelium. However, during inhalation, water enters their mouths and is in direct contact with their gills making it very easy for them to damage themselves. An experiment has been conducted with the zebrafish as it has to rely solely on its gills to breathe. The zebrafish were exposed continuously to cigarette smoke and observations were noted about the gills tissue. After a while, the gills of the Zebrafish had suffered changes and severe irritation. In order to protect themselves from the cigarette extracts, researchers discovered that the fish reproduced very similar aspects of immunopathology that are also found in terrestrial animals. The exposure to cigarette smoke extract actually increased their production of NF-KB-dependent proinflammatory gene expression in the gills which can also be found in mammalian lungs (Progatzky et al., 2016).
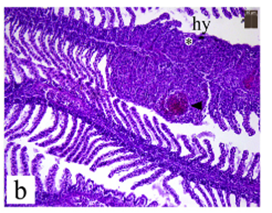
Salinity
There is rather a big difference between the gill's functions regarding ions in seawater compared to freshwater. Seawater has a much larger salt concentration than freshwater, this results in the diffusion of Na+ and Cl– from inside the fish to the water outside when in seawater, as the gills epithelium retains these ions easier (Maetz and Keynes, 1971). Usually fish who have a very light tolerance to salinity variations will remain in freshwater areas, whereas those who can withstand large concentrations or small concentrations of salt in the water will be found in seawater areas. Both teleosts and elasmobranchs have a different process to maintain osmotic regulation (ions). The cartilaginous fish are osmoconformers, which means that they can regulate their internal salt concentration to match the external environment (“Osmoregulators and Osmoconformers” | Biology for Majors II | Lumen Waymaker, n.d.). They regulate that way using secretion from their rectal gland. However, euryhaline teleosts perform osmoregulation, which means they maintain a stable internal salinity no matter the environment. As mentioned previously, when in freshwater, the salinity concentration is lower, they have to perform active absorption, passive gain of water, and excrete diluted water in order to retain their internal salt concentration. The opposite is also true in seawater.
One question still remains: how can euryhaline fish, fish that can osmoregulate, modify their salinity metabolism so quickly when the environment varies? Basically, these fish possess molecular osmosensors that act directly on DNA. The osmotic variations that modify their DNA and proteins trigger the effector mechanisms and the appropriate hormones, cytokines, and receptors to interact with the whole organism (osmosensing). The process then modifies the fish's regulation depending on the exterior's salinity. For example, some of the proteins involved with osmosensing are, for the tilapia, phospholipase C and mitogen-activated protein kinase (Kültz, 2015).
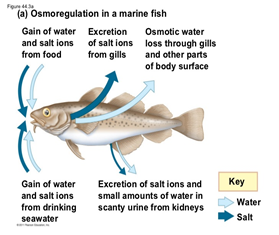
Myth or Reality: Can Fish Actually Drown?
If we were to look up the definition of drowning, we would learn that, according to the Oxford language dictionary, drowning means to “die through submersion in and inhalation of water”. As most of you know, the terrestrial animal lung is incapable of extracting oxygen from water if it were to fill their lungs. Fish, on the other hand, possess gills that allow oxygen from the water to diffuse to their bloodstream. Therefore, “through submersion in and inhalation of water”, fish are incapable of dying (Woods, 2018). They can, however, suffocate even in water, if the oxygen concentration is too low. Knowing that for fish to have access to oxygen water needs to pass through the gills in a unidirectional way, following the counter-current, some fishermen suffocate sharks and fish by dragging them backward, stopping them from gaining oxygen from the water (Cerullo, 2017).
The Challenge of Terrestrial Environment
Life on land has shaped the physiognomy of terrestrial animals. Internal and external structures can vary a lot among animals but stimuli integration that allow adequate ventilation is quite similar. Among those structures, terrestrial animals have evolved a diversity of respiratory system adaptations to obtain oxygen (Fig. 10), such as tracheae in insects, alveoli in mammals and parabronchi in birds (Hinic-Frlog, n.d.). Indeed, animals living on land do not need gills to efficiently extract oxygen from water; it is more abundant in ambient air. Therefore, animals have evolved lungs whose size and complexity are related to the metabolic rate of the animal although the operating of the lungs themselves is relatively similar and will be analyzed in this paper.

Terrestrial Animal Size and Metabolic Requirements
As of today, specialists are aware of the existence of around 4 300 species of mammals having bodies that vary in morphologies and weights from the mouse weighing a few grams to the hippopotamus weighing several tons and not to forget marine mammals like the blue whale that can weigh as much as 120 tons. This wide variety may raise questions like: Do all mammals need the same amount of oxygen? Or: Is the respiratory system of a small animal the same as a very large one? Well, mammals maintain their body temperatures in a narrow range, from 36 to 39 °C. This is why they are called homeotherms. Although it is true that some exceptions occur, such as the platypus that keeps its body temperature between 32 and 33 °C or animals in hibernation, the constancy in body temperature among mammals implies that one or many mechanisms are involved in temperature's regulation among mammals. This means that heat production and dispersion is determined by the metabolic activities of the cells and hence is proportional to body mass (Mortola, “Topics in Respiratory Physiology”). In other words, larger animals need less metabolic activity because the relation is inversely proportional. Indeed, “although the VO2 of the whole organism increases with the size of the species, after normalization by the animal's weight (VO2/kg) the opposite is true: VO2/kg progressively decreases with the increase in animal weight” (Mortola, “Topics in Respiratory Physiology”). This relation is both true within species and among species and it leads to the respiratory adaptations specialists can observe nowadays.
Just like they have an optimal temperature range, mammalian functions have an ideal blood pH range. Blood acidity varies with the concentrations of oxygen dissolved in it. Since both partial pressures of oxygen and carbon dioxide are similar among species while the volume of O2/kg is not constant for each mammal, it creates differences in ventilation, i.e., the action of breathing. This variation further implies that the ventilatory apparatus must be different among species, at structure or function level (Neary et al., 2019). to accommodate differences in ventilation.
Indeed, the modifications related to animals' size in the mammalian respiratory system design also appear to adapt to metabolic functions. As the structure of the respiratory system is very complex, a significant number of variations is possible to accommodate those needs. The respiratory system of mammals is very well proportioned in terms of sizes and gas quantities. For example, the mass of the lung represents approximately 1 % of the total's animal body weight for all animals (Hinic-Frlog, n.d.). Furthermore, lungs can only contain so much air depending on their mass and size, meaning that for the air to be at a predetermined and optimal pressure, the amount of air is proportional to the mammal's lung mass. Also, measurements of lung compliance which is a measure of lung expansion calculated by dividing volume by transpulmonary pressure reveal that this parameter is constant between species. This relation of proportion means that although many variations are possible, general aspects of the internal architecture follow proportion guidelines that are very similar. As a matter of fact, an example of these regularities is the volume of the conducting airways representing a constant fraction of total lung volume (Mortola, “Topics in Respiratory Physiology”). Surprisingly, this even applies to the giraffe, resulting in a very narrow trachea to compensate for its length. Another element that respects proportion is the gas exchange region. The radius of the alveoli being smaller for animals of small sizes, the subdivision of the periphery of the lungs varies and small species usually have a greater number of alveoli. It is important to notice that here, lung surface area is not directly proportional to body weight but rather to the oxygen uptake (VO2). This thus means that the most important variation is achieved structurally when the internal compartmentalization of the lungs varies. Changes is animal's size also cause the alveoli to be bigger among larger species, thus requiring slightly longer diffusion times for gas transfers to and from the pulmonary capillaries; this explains why the breathing rates of the large species tends to be low (Mortola, “Topics in Respiratory Physiology”). Stated differently, at equal pressure applied on the lungs by the diaphragm and intercostal muscles, a rat's lungs will inflate more quickly than an elephant's lungs because, relatively to body weight, the respiratory resistance is not as great, and the time not as long. The air in the rat's lungs has less surface to fill, causing the diffusion time to be shorter than for the elephant.
What Dictates Oxygen Needs?
We know that O2 is carried in the blood by the hemoglobin and CO2 is carried principally in the form of bicarbonate ions HCO3–. Now, since it is not the erythrocytes that vary among mammals, there has to be other divergent parameters, otherwise adaptation to certain circumstances like long-lasting low oxygen conditions would not be possible. Therefore, oxygen carried by the blood essentially depends upon the total mass of blood in circulation and the concentration of hemoglobin (Hackett and Roach, 1995, as cited in Paralikar and Paralikar, 2010; Mortola, “Topics in Respiratory Physiology”). Those two parameters are also approximately constant in all mammals. Thus, oxygen concentration in arterialized blood is relatively fixed but varies under certain circumstances like the one previously mentioned. For example, at high-altitude (Fig. 11) or in very small animals with high metabolic requirements (like shrews or bats), the hematocrit which is the proportion of red blood cells in the blood increases, favoring O2 delivery.

As mentioned earlier, mammals have different metabolic requirements based on the body weight but also on the environmental conditions, physical activity or simply phylogeny which is the evolution of a species. However, “the differences in metabolic requirements among mammals are not accompanied by differences in the O2 contained in the arterial blood, which is an almost fixed proportion of body weight, but by differences in the ability to unload oxygen in the tissue capillaries” (Mortola, “Topics in Respiratory Physiology”). This process is guided by the Bohr effect: when there is high [H+], largely determined by the quantity of CO2 in the blood, this lowers the blood pH, the hemoglobin affinity is decreased and oxygen flows in the tissue capillaries (Benner et al., 2020). Although the Bohr effect is applied at lung level and dictates the ventilation mechanism, the chemoreceptors are not located in the lungs themselves but rather in the respiratory center at the base of the brain. This does not mean there are no receptors in the lungs. Indeed, breathing pattern has to be controlled by the respiratory center in the brain and the way this regulation happens is by the presence of mechanoreceptors located in the chest walls and in the airways. It is very important that both series of receptors send information because the lungs and chest are closely related due to the chest arrangement. Receptors in the chest wall allow for reflex control of the respiratory muscles and the integration of their respiratory activity with other functions of the body, whether they are related to survival or not as well as (Mortola, “Topics in Respiratory Physiology”) As for the receptors in the airways, they send information via the vagus nerve to regulate depth and frequency of breathing (Benner et al., 2020). Combined in all mammals species, both sets of receptors, although they have similar general properties, have different reflex contribution since breathing frequencies get lower as the mammals' size increases. But then could that explain why whales, the biggest marine mammals can spend almost 90 minutes without breathing? In fact, it is a little more complicated than that.
Whales' Oxygen Management
When it comes to breathing, marine mammals like whales and dolphins are more like us humans than like fishes. In evolutionary history, cetaceans transitioned from land to sea, thus presenting the most drastic adaptations among mammals. They are constantly facing a big challenge that terrestrial mammals do not experience: their aquatic environment causes acute tissue hypoxia during diving (Nery et al., 2013). Therefore, “whales have evolved several physiological, anatomical, and behavioral strategies to cope with extended periods of limited O2 availability when submerged” (Nery et al., 2013). As expected, these adaptations need to allow whales to save oxygen. Thus, the metabolic rate is reduced and specific organs are mainly targeted by oxygen supply mechanisms because of selective vasoconstriction. Other than metabolic rate and vasoconstriction adaptations, the over-expression of myoglobin (Mb) in aerobic muscles, a protein that binds and delivers oxygen during extended periods of hypoxia is of crucial importance (Hecker, 1998; Nery et al., 2013). High myoglobin concentrations will allow whales to store more oxygen and produce nitric oxide during hypoxia, which regulates metabolism and blood flow in muscles and tissues. Indeed, nitric oxide reduces tissue damage that might happen when blood flow is restricted, which is crucial since selective vasoconstriction is used during prolonged dives. In other words, myoglobin in whales does not have different physicochemical characteristics such as a higher Bohr effect; it simply adapted to the physiological needs of the cetacea by optimizing the oxygenation-deoxygenation cycle (Nery et al., 2013).
Tracheal Systems
Insects do not have a respiratory pump like mammals do. In fact, their breathing system is a lot more efficient than any other animals, including fishes. Why is that? For insects, blood is not an intermediate in the oxygenation of their system since their respiration is independent of their circulatory system. In fact, their respiratory system is a tracheal system, which consists of “a network of small tubes that carries oxygen directly to the entire body” (Hinic-Frlog, n.d.). Chitin, a polymeric material is what the tubes consist of. As shown on (Fig. 12), insects have openings, called spiracles along the thorax and abdomen. Entering and leaving the tracheal system through these apertures, air is filtered and oxygen may pass into the body when the spiracles muscular valves are not closed. Spiracles also regulates CO2 diffusion and water vapor.
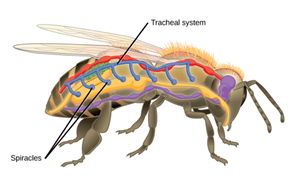
Interesting fact, gas movements take place differently in sedentary insects versus in active insects. Some insects rely on oxygen and carbon dioxide diffusion whereas active species ventilate the tracheal system mechanically. This is achieved by pumping movements of the abdomen and cause a resulting force on streams of air near the spiracles; sucking it in or pushing it out. In addition, among the insects that are very active, the volume of air displaced during ventilation is increased by air sacs; large and thin dilatations of the trachea (Wigglesworth, 2020). An imbalance in gas accumulation provides information that will travel to nerve centers to modify mechanical ventilation and contribute to adequate ventilation.
A Common Breathing Pattern
A quick glance of the mammalian resting breathing pattern reveals that it appears as a sinusoidal function (Fig. 13), of which “the amplitude is the tidal volume (VT) and the period is the total breath duration (Ttot)” (Mortola, “Topics in Respiratory Physiology”).
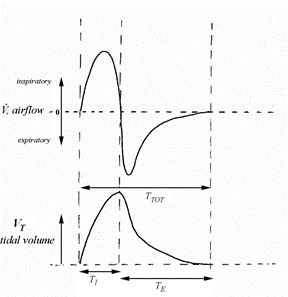
However, a closer look reveals that there are some major deviations from the sinusoidal function due to the fact that Ttot is not divided uniformly between the inspiratory and expiratory phases of the cycle. By testing it yourself you would realize that, as seen on (Fig. 13), the inspiratory time (TI) is generally shorter than the expiratory time (TE). Also, an independent analysis of the inspiratory and expiratory phases indicate that the shape of the graph is different: inspiratory phase is convex while expiratory is more concave. Nevertheless, it is quite fascinating to see that in (Fig. 14), even with all the variety in size and shape among mammals, the spirogram is similar. Visually, the function has the same characteristics. The spirogram even remains comparable when the posture is changed, going from supine (on the back) to prone (on the belly), sitting or standing, or even by walking slowly (Mortola, “The heart rate – breathing rate relationship in aquatic mammals”).
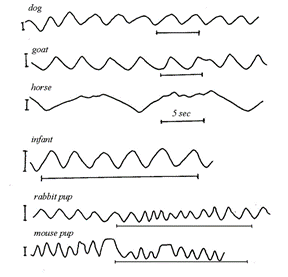
Thus, the ‘stability' of the spirogram is explained by the fact that the respiratory system has receptors to recognize mechanical load, such as compression of the lungs by the heart in supine position, granting the system the ability to respond so quickly that volume compensation occurs within the breath itself (Mortola, “Topics in Respiratory Physiology”). This concept brings up one of the fundamental aspects of breathing which is load compensation.
Load Compensation
Since the lungs are located in the chest, they have common muscles and structures with multiple non-respiratory organs. This design of the body is crucial for the blood to be oxygenated and reach the capillaries of the tissues. However, it causes the lungs to undergo other forces that do not result from breathing. Therefore, there needs to be a mechanism that controls the mechanical result of the neural output to counteract the continuously changing loads. As we know, breathing feels very natural most of the time, is not energetically demanding and even though mammals cannot see it, homeostasis of oxygen and carbon dioxide in blood is carefully regulated. This indicates that mechanisms have therefore evolved to closely track changes in lung volume and to instantly signal to the brain region responsible for stimuli integration if any deviations from the regular pattern is detected (Mortola, “Topics in Respiratory Physiology”). In other words, the respiratory system is an interdependent neuro-mechanical unit where the neural motor output is conditioned by the mechanical event. It follows the principles of homeostasis and that is why the functioning of this unit is not a direct pathway but rather based on a feedback loop: the neurons located in the section of the brain that controls the respiratory system send signals by the respiratory muscles and from the output travels from the peripheral nervous system, back to the brain thanks to lungs and chest receptors previously mentioned (Segizbaeva, 2010). As depicted in Fig. 15, “information about the changes in lung volume is re-inserted to the respiratory pattern generator via activation of the muscles proprioceptors (long proteins parallel to the muscle fibers that give signals to the nervous central system)” (Mortola, “Topics in Respiratory Physiology”) and airways receptors.
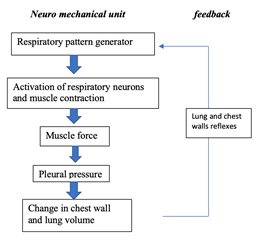
This happens really fast as it results from the autonomic nervous system. A slower reaction can also happen and it is caused by humoral stimuli, thus especially affecting gases dissolved in blood. It is different since this type of information controls ventilation instead of tidal volume when it reaches the neurons controlling the respiratory motor output. Now, let us look further on how most mammals' ventilation is conducted by the neural connexions.
Neural Basis of the Respiratory Output
In the late 1800s and early 1900s, it was noticed by vivisectionists (who performed experiments on live animals) that when the cerebrum of cerebellum was removed, no signs of modifications in the respiration were detected: it was not affected. On the other hand, breathing ceased when the roots of the vagus nerve in the medulla (Fig. 16) were cut. The vagus nerve is the longest of the cranial nerves which represents the main component of the parasympathetic nervous system (Wijdicks, 2019).
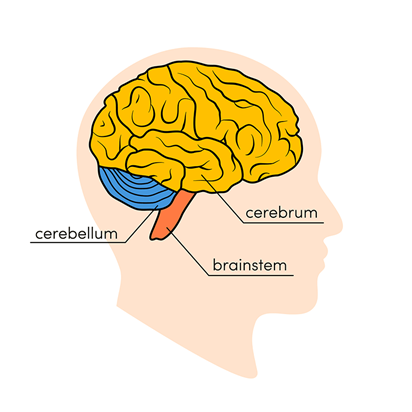
Later on, in the 1920s, another researcher, Lumsden brought a more precise concept and concluded through careful transections at the posterior part of the brain (close to the medulla) in different mammalian species that “the central respiratory system can be divided into three centers: pneumotaxic, apneustic and inspiratory centers, the last one being subdivided into the expiratory and gasping centers” (Mortola, “Topics in Respiratory Physiology”). Here, when we say centers, we refer to common groups of neurons that have the same function in the regulation of the respiratory system. Lumsden found through electrical stimulation that the first center (pneumotaxic) modulates/regulates the rhythm of the respiration by conveying signals to the relevant neurons in the medulla. Although the isolated medulla may generate an intrinsic respiratory pattern without the presence of neutrons, it will only do so if necessary, meaning if the information cannot travel back to the neurons. Also, theories might have been expressed regarding the different centers of control in the brain but one very important thing is that cellular assembling involved in generation of respiratory activity and breathing rhythmicity is still a mystery to specialists. The brain is indeed a very complex organ to analyze (Wijdicks, 2019). However, assumptions and hypotheses have been expressed regarding the mechanisms involved in regular neuronal activity and two possibilities emerged: the pacemaker cells of the brain (careful not to mistake with the pacemaker cells of the heart even if the function is similar) and the neural network (Mortola, “Topics in Respiratory Physiology”). The pacemaker cells' particularity is that they do not require interactions with other neurons. On their own, their basic pattern is to exert control over neuronal circuit function by their ability to generate rhythmic bursts of action potential (Harris-Warrick, 2010). Basically, the central point of the pacemaker neuron hypothesis is that even when completely isolated from all synaptic input, these cells continue to oscillate and fire rhythmic bursts of action potentials, providing the rhythmic synaptic drive to the following neurons to generate the rhythm. Pacemaker cells' presence in the subesophageal ganglion of both hermit crabs and lobsters was established by observing that rhythmic pattern continues in the absence of inputs to the cell (Harris-Warrick, 2010).
As for the second possibility: it represents the opposite because the neural network would imply interactions among cells, yet both pacemaker cells and the network result in a rhythmic output pattern of breathing (Mortola, “Topics in Respiratory Physiology”). However, neural network models cannot be further explained since no satisfactory explanation regarding the generation of rhythm in respiration has been fully proposed. (Harris-Warrick, 2010). Finally, the theory involving more than one network being responsible for respiratory activity also has to be considered as some evidence indicates that gasping reflexes at the end of a normal breathing cycle in hypoxia conditions may result in the implication of unusual neural pools. By unusual, I mean that the network is not usually controlling the regular breathing pattern.
The Maintenance of Homeostasis in the Avian Respiratory System
Introduction to the Avian Respiratory System
As with all animals, the primary function of the avian respiratory system is gas exchange in which molecular oxygen is delivered from the environment to the body tissues, and carbon dioxide is removed from the tissues and is expelled into the environment. Additionally, another important role of the respiratory system in birds is thermoregulation, a component of homeostasis involving the maintenance of internal body temperature (Ritchison, “Bird Respiratory System”). From a functional perspective, what sets the avian respiratory system apart from that of mammals or reptiles is that it is typically able to maintain a consistently higher rate of gas exchange over relatively lengthy periods of time (Powell, 2015). The efficiency of the avian respiratory system is not a random phenomenon, rather it is an absolute biological necessity for flight. The oxygen demand is very high in the muscles of a bird during flight, due to the consistent, high levels of energy required to maintain lift. Birds have evolved a unique respiratory system capable of some of the highest rates of oxygen delivery in the animal kingdom (West et al., 2007). The basic principles and biological mechanisms of the mammalian respiratory system are also found in the avian respiratory system, such as oxygen and carbon dioxide transportation in the blood, diffusion through vascular membranes, pulmonary gas exchange, and tissue gas exchange. Despite this, the avian respiratory system is truly unique from a structural perspective, since the functions of ventilation and gas exchange are split between two different sets of structures: nine large air sacs for ventilation, and two small lungs for gas exchange (Ritchison, “Bird Respiratory System”). This system is functionally advantageous over other vertebrate respiratory systems, especially in states of hypoxia.
The Anatomy of The Avian Respiratory System
The most unique aspect of the avian respiratory system is its heterogeneous partitioning of the respiratory structures, as opposed to the homogeneity of the partitioning in the mammalian respiratory system. What this means is that in birds, ventilation and gas exchange do not occur in the same organ, unlike other terrestrial vertebrate respiratory systems in which both processes occur together at the alveoli inside the lungs (Powell, 2015). In birds, the lungs only carry out gas exchange, but not ventilation, since they are small, rigid, and do not expand or contract the way mammalian lungs do. Instead, nine other structures located in the bird's chest and abdomen are used for ventilation (Ritchison, “Bird Respiratory System”). These are called the air sacs. The nine air sacs are far larger by volume than the two actual lungs which they are situated around. The air sacs are quite flexible and possess significant air pumping capacity due to their sheer size, relative to the rest of the body. In fact, even though birds' lungs typically only account for 1 % to 3 % of body volume, the respiratory system as a whole makes up 15 % of body volume, mostly accounted for by the air sacs (Powell, 2015). For comparison, a typical mammalian respiratory system only occupies about 7% of body volume (Powell, 2015).
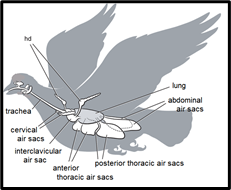
The air sacs permit a unidirectional flow of air through the lungs of birds. Unidirectional flow means that air moving through a bird's lungs is largely quite oxygen rich. In contrast, air flow is bidirectional in mammals, moving back and forth, in and out of the lungs. As a result, air coming into a mammal's lungs is mixed with oxygen-deprived air that has already been in the lungs for a while. The resulting mixed air has less oxygen than pure fresh air. This is why more oxygen is available to diffuse into the blood in a bird's lungs, relative to the lungs of a similarly sized mammal (Ritchison, “Bird Respiratory System”).
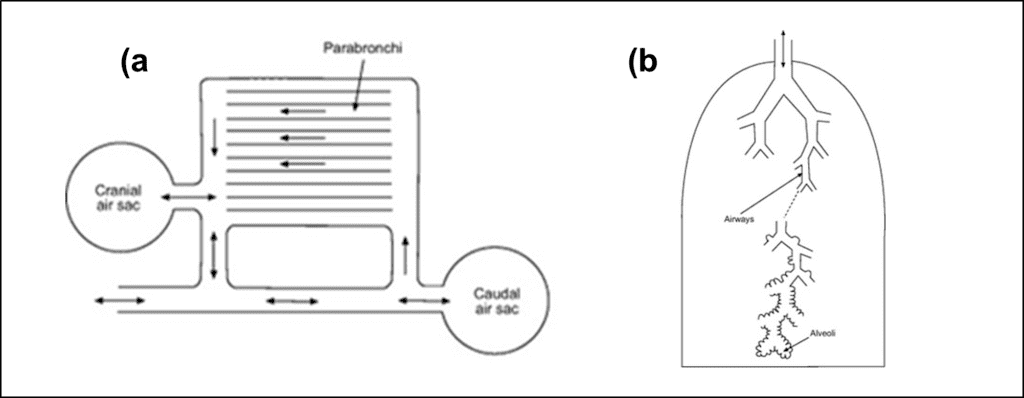
Much like mammals, birds can inhale and exhale air through both the mouth and a pair of nasal slits on the beak called nares. Another similarity is that birds also possess a larynx which separates the oronasal cavity from the trachea. Birds also have a thin slit-like glottis which opens up to the trachea at the larynx. In birds, laryngeal muscles are used to help with inhalation by contracting to open the glottis and decrease the resistance to inspiratory airflow (Ritchison, “Bird Respiratory System”). This is unlike mammals which do not contract the larynx on every inhalation, but rather use laryngeal muscles as a vocal organ.
In birds, the vocal organ is the syrinx, which is located at the lower end of the trachea at the junction where it splits into two separate tubes called the extrapulmonary primary bronchi. These continue on to the lungs, with one bronchus entering each lung. The extrapulmonary primary bronchi are typically quite short and connect to the lungs only a short distance from the syrinx (Powell, 2015). Once a primary bronchus enters a lung, it is called an intrapulmonary primary bronchus or a mesobronchium (Ritchison, “Bird Respiratory System”). The primary bronchus travels through the entire length of the lung, exiting at a small opening into the abdominal air sac.
Smaller tubes called secondary bronchi branch off from the primary bronchus. These secondary bronchi can be divided into two functional structures based on their orientation and connection to the primary bronchus. At the end of the lung closest to the bird's head is a collection of four or five secondary bronchi called ventrobronchi. This collection of ventrobronchi branches out from the primary bronchus in an approximately perpendicular orientation to form a fan-like structure called the cranial group. At the opposite end of the lung is a collection of six or ten secondary bronchi called dorsobronchi. This collection of dorsobronchi branches out from the primary bronchus in an approximately perpendicular orientation to form another fan-like structure called the caudal group (Powell, 2015). The primary and secondary bronchi in the avian respiratory system are conducting airways because they do not participate in gas exchange (Powell, 2015).
The parabronchi are very narrow tubes branching off the secondary bronchi. They are the functional units of gas exchange in the avian respiratory system, analogous to the alveoli in mammalian lungs. Gas exchange in the parabronchi occurs within small honeycomb-like holes proliferating the walls. Between the two fan-like structures at either end of the lung, formed by the cranial and caudal groups, are a connecting series of several hundred parabronchi. In particular these parabronchi are the paleopulmonic parabronchi (Powell, 2015). The primary bronchus, cranial and caudal groups of secondary bronchi, and the parabronchi comprise the fundamental scheme of airways in the avian lung (Duncker, 1972).
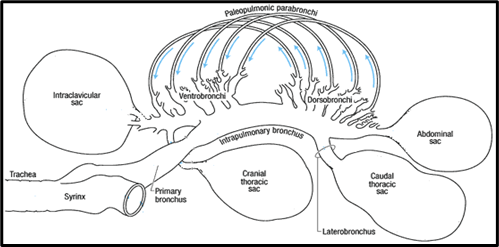
The Airflow of Avian Respiration
On the first inhalation, the air moves down the trachea into the posterior air sacs. The bird then exhales and the air moves into the lungs where gas exchange occurs in the parabronchi. On the next inhalation, the air is pushed unidirectionally through the parabronchi in the lungs and into the anterior air sacs. On the subsequent exhalation, the gas in the anterior air sacs is expelled from the body, via the trachea. This is what allows birds to have unidirectional airflow, which in turn always for greater renewal of the gas exchanging volume. The result is that birds may exchange gas with unmatched efficiency, despite having proportionally smaller respiratory surfaces than mammals (Ritchison, “Bird Respiratory System”).

Avian Respiratory Control and Regulation
In terms of direct control of the respiratory system, respiratory muscles are used. They generate the pressure forces to transport air in and out of the air sacs and through the parabronchi in the lungs. This pressure in the respiratory system is manipulated via changes in body volume caused by respiratory muscle activity, which involve the compression or stretching of certain air sacs, as seen in Fig. 22. This causes the air sacs to act as bellows and ventilate the system. Unlike mammals, the true resting body volume of a bird is midway between its inspiratory volume and its expiratory volume (Powell, 2015).
The activity of the respiratory muscles directly regulates a rate value known as ventilation. The ventilation V̇ of avian and mammalian respiratory systems is proportional to the tidal volume VT (the volume per breath) and is also proportional to the respiratory frequency, both of which are inversely proportional to each other when the ventilation is held constant. This means that the ventilation can be increased by breathing at a faster rate, or by breathing deeper (Powell, 2015).
V̇ =(V_T)(f_R)
(1)
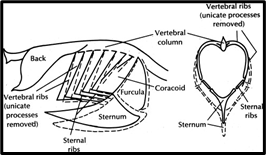
Ventilation can be considered the rate at which gas enters and leaves the cavities of the respiratory system. Therefore, when a bird's body demands greater rates of gas exchange, it increases its ventilation using the respiratory muscles to allow for faster breathing, deeper breathing, or both.
Tracheal volume is an important factor for determining the proportion of ventilation that does not contribute toward the rate of gas exchange. This is because the trachea is one of the largest conducting airways in most terrestrial vertebrates. The following function is used to model the observed relationship between tracheal volume and body mass in twenty-seven sampled bird species (Hinds and Calder, 1971). V represents tracheal volume in mL and Mb represents body mass in kilograms.
V=3.7(M_b)^{109}
(2)
This function predicts tracheal volume in a vast majority of birds with an error of less than 25 %. Tracheal volume is 4.5 times larger in birds than in comparably sized mammals (Hinds and Calder, 1971). Birds generally compensate for this increased dead space with a deep and slow breathing pattern (Bouverot, 1978). This is because deeper, slower breathing distributes more of the gas farther into the cavities of the respiratory system, where the respiratory surfaces are located, meaning a smaller proportion of inhaled air remains in the dead space of the trachea.
Avian Respiratory Regulation and Maintenance of Homeostasis
In birds, ventilation is regulated to meet the demands caused by changes in metabolic activity, as well as forebrain-controlled behavior, emotional inputs, and a wide range of sensory inputs such as body temperature. For example, birds lack the ability to sweat, so the respiratory system is important for thermoregulation, helping to low the body temperature when it is too high by means of evaporative water loss. This is useful for helping to prevent overheating during flight.
Unlike mammals, birds do not have a diaphragm and so they do not possess a phrenic nerve. This means that birds have different motoneuron pathways for respiration than mammals. Although it has not yet been unequivocally demonstrated, it is assumed that there is a central respiratory control center in the avian brain. It appears that the central neurological pattern generator appears to be located in the pons and medulla oblongata of the brain, just like with mammals (Ludders, 2001).
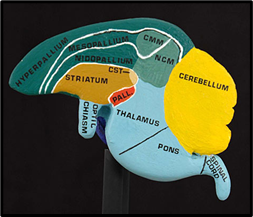
Evidence suggests that the chemical control of respiratory frequency and inspiratory-expiratory duration depend on feedback from both intrapulmonary as well as extrapulmonary receptors. In birds, the central chemoreceptors affect ventilation in response to changes in the arterial partial pressure of carbon dioxide and hydrogen ion concentration (Ludders, 2001). Peripheral extrapulmonary chemoreceptors (ECR), such as the carotid bodies found in carotid arteries, are influenced by the partial pressure of oxygen, and increase their discharge rate as the partial pressure of oxygen decreases, causing an increase in ventilation. These receptors decrease the rate of discharge as the partial pressure of oxygen increases or the partial pressure of carbon dioxide decreases (Ritchison, “Bird Respiratory System”). These responses are the same as those observed in mammals.
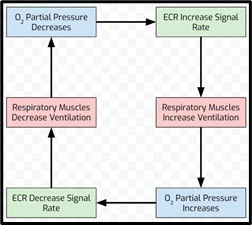
Unlike mammals, birds possess a unique group of peripheral receptors located inside the lungs, called intrapulmonary chemoreceptors that are highly sensitive to carbon dioxide and insensitive to decreases in the partial pressure of oxygen. The intrapulmonary chemoreceptors affect the tidal volume and the respiratory frequency rate on the scale of individual breaths. These receptors act as the key component of a uniquely avian inspiratory-inhibitory reflex that is highly sensitive to the extent of carbon dioxide washout from the lung during inspiration (Ludders, 2001). This inhibitory reflex helps to continuously optimize ventilation, which further contributes toward the high efficiency of the avian respiratory system.
An interesting example of respiratory regulation can be observed in many small birds. In these birds, the wishbone and sternum are mechanically coupled during flight, so that the beats of the wings assist ventilation (Jenkins et al., 1988). The very act of flight requires increased ventilation, so it is efficient to use some of the kinetic energy from wing beats to help aid in regulating greater ventilation, which in turn helps to regulate the increased demand for oxygen caused by the physical exertion of flight. Despite the lack of a physical connective respiratory mechanism in larger birds, wing movements are still coordinated with ventilation, which may be indicative of the coupling of neuromuscular circuits involved in ventilation with those that are involved in flight (Powell, 2015).
Conclusion
To conclude, organisms depend upon oxygen for survival, independently evolving diverse respiratory systems for acquiring oxygen from the environment. Habitat expansions constrain the use of different gas exchangers such as skin, gills, tracheae, lungs, and their intermediate stages that may coexist within the same species whether that coexistence is disjunct or simultaneous. After analyzing the stimuli integration regarding the breathing of the different organisms, we conclude that disparate systems exhibit similar directions of adaptation to obtain the oxygen they need in the right quantity. This implies that the respiratory apparatus adapts in various ways to regulate oxygen uptake but the information in case of hypoxia or hyperoxia for example, is treated in the brain by a similar system in all animals.
References
Ballintijn, C. M. (1982). Neural Control of Respiration in Fishes and Mammals. In A. D. F. Addink & N. Spronk (Eds.), Invited Lectures (pp. 127-140): Pergamon. Retrieved from https://www.sciencedirect.com/science/article/pii/B9780080279862500177
Benner, A., Patel, A. K., Singh, K., & Dua, A. (2020). Physiology, Bohr Effect. In StatPearls. Treasure Island (FL): StatPearls Publishing
Bouverot, P. (1978). Control of breathing in birds compared with mammals. Physiological Reviews, 58(3), 604-655. doi:10.1152/physrev.1978.58.3.604
Bretz, W. L., & Schmidt-Nielsen, K. (1972). The Movement of Gas in the Respiratory System of the Duck. Journal of Experimental Biology, 56(1), 57-65. doi:10.1242/jeb.56.1.57
Candela, L. (2020). Neural Mechanisms (Respiratory Center). In Anatomy and Physiology (Boundless). Retrieved from https://med.libretexts.org/@go/page/8001
Cerullo, M. (2017). SEE IT: Shark gets dragged behind motorboat at high speed in Florida. Retrieved from https://www.nydailynews.com/news/national/shark-dragged-behind-motorboat-high-speed-fla-article-1.3355087
Duncker, H.-R. (1972). Structure of avian lungs. Respiration Physiology, 14(1), 44-63. doi:10.1016/0034-5687(72)90016-3
Evans, D. H., Piermarini, P. M., & Potts, W. T. W. (1999). Ionic transport in the fish gill epithelium. Journal of Experimental Zoology, 283(7), 641-652. doi:10.1002/(SICI)1097-010X(19990601)283:7<641::AID-JEZ3>3.0.CO;2-W
Fedde, M. R. (1986). Respiration. In P. D. Sturkie (Ed.), Avian Physiology (pp. 191-220). New York, NY: Springer New York. doi: https://doi.org/10.1007/978-1-4612-4862-0_8
Fishbio. (2015). An Efficient Exchange. Retrieved from https://fishbio.com/field-notes/fish-biology-behavoir/an-efficient-exchange
Gilmour, K. M., & Perry, S. F. (2006). Branchial Chemoreceptor Regulation of Cardiorespiratory Function. In Fish Physiology (Vol. 25, pp. 97-151): Academic Press. Retrieved from https://www.sciencedirect.com/science/article/pii/S1546509806250039
Harris-Warrick, R. M. (2010). General principles of rhythmogenesis in central pattern generator networks. Progress in Brain Research, 187, 213-222. doi:10.1016/b978-0-444-53613-6.00014-9
Hecker, B. (1998). How do Whales and Dolphins Sleep Without Drowning?
Hinds, D. S., & Calder, W. A. (1971). TRACHEAL DEAD SPACE IN THE RESPIRATION OF BIRDS. Evolution, 25(2), 429-440. doi:10.1111/j.1558-5646.1971.tb01899.x
Hinic-Frlog, S. (n.d.). Respiratory Structures and Their Function. In Introductory Animal Physiology
Jarvis, E. (2005). Bird Brain. Retrieved from https://www.pbs.org/wgbh/nova/sciencenow/3214/03-brain.html
Jenkins, F. A., Dial, K. P., & Goslow, G. E. (1988). A Cineradiographic Analysis of Bird Flight: The Wishbone in Starlings Is a Spring. Science, 241(4872), 1495-1498. Retrieved from https://science.sciencemag.org/content/sci/241/4872/1495.full.pdf
Kostić, J., Kolarević, S., Kračun-Kolarević, M., Aborgiba, M., Gačić, Z., Paunović, M., . . . Vuković-Gačić, B. (2017). The impact of multiple stressors on the biomarkers response in gills and liver of freshwater breams during different seasons. Science of the Total Environment, 601-602, 1670-1681. doi:10.1016/j.scitotenv.2017.05.273
Kültz, D. (2015). Physiological mechanisms used by fish to cope with salinity stress. Journal of Experimental Biology, 218(12), 1907-1914. doi:10.1242/jeb.118695
Ludders, J. W. (2001). Inhaled Anesthesia for Birds. In Recent Advances in Veterinary Anesthesia and Analgesia: Companion Animals: International Veterinary Information Service. Retrieved from https://www.ivis.org/library/recent-advances-veterinary-anesthesia-and-analgesia-companion-animals/inhaled-anesthesia
Lumb, C. K. (2017). Chapter 25 – Comparative Respiratory Physiology. In A. B. Lumb (Ed.), Nunn's Applied Respiratory Physiology (Eighth Edition) (pp. 357-375.e352): Elsevier. Retrieved from https://www.sciencedirect.com/science/article/pii/B9780702062940000253
Maetz, J., & Keynes, R. D. (1971). Fish gills: mechanisms of salt transfer in fresh water and sea water. Philosophical Transactions of the Royal Society of London. B, Biological Sciences, 262(842), 209-249. doi:doi:10.1098/rstb.1971.0090
Mangieri, A. (n.d.). Class Chondrichthyes. Retrieved from http://angelenamangieri.weebly.com/class-chondrichthyes.html
Marshall, P. T., & Hughes, G. M. (1965). The physiology of mammals and other vertebrates (1 ed.): Cambridge University Press.
Medicine, J. H. (n.d.). Brain Anatomy and How the Brain Works. Retrieved from https://www.hopkinsmedicine.org/health/conditions-and-diseases/anatomy-of-the-brain
Milsom, B. (n.d.). Function of the Respiratory System. In: UBC Department of Zoology. Retrieved from https://www.zoology.ubc.ca/~milsom/PDFs/Lecture%2027.pdf
Mortola, J. P. (2015). The heart rate – breathing rate relationship in aquatic mammals: A comparative analysis with terrestrial species. Current Zoology, 61(4), 569-577. doi:10.1093/czoolo/61.4.569
Mortola, J. P. (n.d.). Topics in Respiratory Physiology for Undergraduate Students In: McGill University. Retrieved from http://www.medicine.mcgill.ca/physio/resp-web/default.htm
Neary, J. P., Singh, J., Bishop, S. A., Dech, R. T., Butz, M. J. A., & Len, T. K. (2019). An Evidence-Based Objective Study Protocol for Evaluating Cardiovascular and Cerebrovascular Indices Following Concussion: The Neary Protocol. Methods and protocols, 2(1), 23. doi:10.3390/mps2010023
Nery, M. F., Arroyo, J. I., & Opazo, J. C. (2013). Accelerated evolutionary rate of the myoglobin gene in long-diving whales. Journal of Molecular Evolution, 76(6), 380-387. doi:10.1007/s00239-013-9572-1
Paralikar, S. J., & Paralikar, J. H. (2010). High-altitude medicine. Indian Journal of Occupational and Environmental Medicine, 14(1), 6-12. doi:10.4103/0019-5278.64608
Powell, F. L. (2015). Chapter 13 – Respiration. In C. G. Scanes (Ed.), Sturkie's Avian Physiology (Sixth Edition) (pp. 301-336). San Diego: Academic Press. Retrieved from https://www.sciencedirect.com/science/article/pii/B9780124071605000130
Pritchard, J. B. (2003). The gill and homeostasis: transport under stress. American Journal of Physiology-Regulatory, Integrative and Comparative Physiology, 285(6), R1269-R1271. doi:10.1152/ajpregu.00516.2003
Progatzky, F., Cook, H. T., Lamb, J. R., Bugeon, L., & Dallman, M. J. (2016). Mucosal inflammation at the respiratory interface: a zebrafish model. American Journal of Physiology-Lung Cellular and Molecular Physiology, 310(6), L551-L561. doi:10.1152/ajplung.00323.2015
Ritchison, G. (n.d.). Bird Respiratory System Retrieved from http://people.eku.edu/ritchisong/birdrespiration.html
Segizbaeva, M. O. (2010). Loading and unloading breathing during exercise: respiratory responses and compensatory mechanisms. European Journal of Medical Research, 15 Suppl 2(Suppl 2), 157-163. doi:10.1186/2047-783x-15-s2-157
Spencer, E. (2020). How Do Gills Work? Retrieved from https://oceanconservancy.org/blog/2020/01/17/gills/
Tavighi, S., Saadatfar, Z., Shojaei, B., & Behnam Rassouli, M. (2016). Histomorphogenesis of cranial nerves in Huso huso larvae. Veterinary research forum : an international quarterly journal, 7(2), 111-116. Retrieved from https://www.ncbi.nlm.nih.gov/pmc/articles/PMC4959338/
Taylor, E., Leite, C., McKenzie, D., & Wang, T. (2010). Control of respiration in fish, amphibians and reptiles. Brazilian journal of medical and biological research = Revista brasileira de pesquisas medicas e biologicas, 43 5, 409-424.
Taylor, E. W. (2011). CONTROL OF RESPIRATION | Generation of the Respiratory Rhythm in Fish. In A. P. Farrell (Ed.), Encyclopedia of Fish Physiology (pp. 854-864). San Diego: Academic Press.
Walker, K. (2012). Osmoregulation. In. slideshare.net. Retrieved from https://www.slideshare.net/kristenw3/osmoregulation-12005537
Waymaker, L. (n.d.). Osmoregulators and Osmoconformers. In. Biology for Majors II. Retrieved from https://courses.lumenlearning.com/wm-biology2/chapter/osmoregulators-and-osmoconformers/
Web, W. o. t. (2017). Dissolved Oxygen. Retrieved from https://www.waterontheweb.org/under/waterquality/oxygen.html
West, J. B., Watson, R. R., & Fu, Z. (2007). The human lung: did evolution get it wrong? European Respiratory Journal, 29(1), 11-17. doi:10.1183/09031936.00133306
Westneat, M. (1998). Vertebrates: Comparative Anatomy, Function, Evolution.— Kenneth V. Kardong. 1998. Second Edition. McGraw-Hill, Boston, Massachusetts. Systematic Biology, 47(4), 762-763. doi:10.1080/106351598260743
Wigglesworth, V. B. (2020, 22 Dec. 2020). Insect. Retrieved from https://www.britannica.com/animal/insect
Wijdicks, E. F. M. (2019). Noeud Vital and the Respiratory Centers. Neurocritical Care, 31(1), 211-215. doi:10.1007/s12028-019-00686-8
Woods, R. (2018). Can Fish Drown? A Deep Dive into Whether it's Physically Possible. Retrieved from https://www.fishkeepingworld.com/can-fish-drown/