Feather Structure and Assembly
Ziyad Bouslama, Young Chae Han, Stephanie Makhlouf, Catherine Xu
Introduction
Birds are the only animals alive that have developed feathers, evolving from the scales of reptiles. Feathers are necessary for flight, insulation, and courtship displays. As a portrayal of the importance of feathers: for nearly two decades, researchers have been trying to imitate bird flight, a goal hindered by the use of rigid feather-like panels and a lack of understanding of the skeletal and muscular mechanics for wing morphing. Recently, researchers created the ‘Pigeon-bot', a robot with artificial limbs and fingers, but real pigeon feathers. They found that feather hooklets act like velcro, interlocking when the wings extend and unlocking automatically when they are flexed, which is a necessary trait for feather coordination during dynamic wing morphing (“PigeonBot's feather-level insights push flying bots closer to mimicking birds” | EurekAlert! | American Association for the Advancement of Science, 2020). This is one of the closest attempts that humans have made in recreating bird flight.
Differences in colors and shapes allow us to distinguish between species of birds and sometimes between males and females. Some birds can possess up to 20 000 feathers that can regenerate. Feather regeneration refers to the cycle by which birds shed worn feathers and replace them with new feather types at generally four different physiological stages including natal down, juvenal, alternate plumages, and basic plumages (Pittaway, 2000). The natal down plumage is produced during embryonic development to provide thermoregulation when the chick hatches and may consist of a few scattered down feathers – as found on most hatchling land birds – or it may be a dense, fuzzy covering – as found on ducklings. In some birds, the natal down is generally replaced a week or two after hatching by the juvenal plumage. The juvenal plumage replaces natal down via a complete molt called the prejuvenal molt. They start forming in the feather follicle during the late embryonic stage, then they completely replace natal down after hatching. The juvenal plumage is often duller than the natal down plumage (Pittaway, 2000).
The basic plumage is generally worn during fall, winter, and early spring. The adult basic plumage is the definitive plumage to which adults return to after every breeding season. In alternate plumage – the plumage usually used for breeding season – the adult alternate plumages of males differ from their adult basic plumages, whereas in females, both plumages are similar. Birds in their first alternate plumage are generally duller than those in the adult alternate plumage.
Feathers are highly ordered, hierarchical branched structures. While feathers can come in various shapes and sizes, they are all composed of the same parts and are constructed from the protein beta-keratin . At the early stages of the developmental stage, alpha-keratins are present in the cells of rachis. In later stages of the developmental process, beta-keratins make up 90 % of the barbs and barbules. In most cases, a feather is made up of either a single filament or numerous filaments of barbs, which are connected by a rachis to form a branched structure. This branched structure is derived from a hollow calamus, which extends into a central rachis. The barbs will also branch into barbules and hooklets in a more complex feather (Fig. 1). Following this basic structure, feathers come in various forms depending on their function and evolutionary modifications from the past (Benton et al., 2019). Furthermore, these feathers are not randomly placed on the avian body. They follow a specific geometrical distribution that starts from inside the egg and continues to evolve during the bird's life. In this report, we describe the mechanisms and chemistry behind feather assembly: specifically, feather bud distribution, feather growth, branch morphogenesis, barb keratinization, and feather pigmentation on a cellular and molecular level.
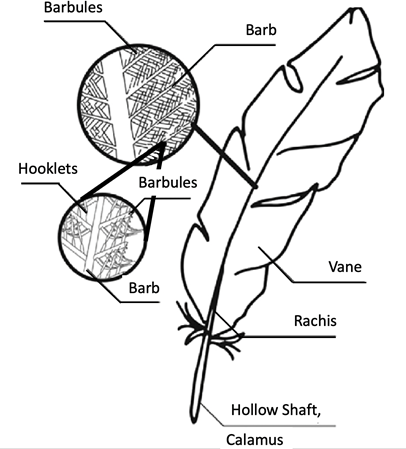
Feather Bud Distribution
The specific distribution of a bird's feather is expressed as early as the embryo stage. Here, the case of a chicken's embryo will be studied.
The formation of feather buds starts precisely at embryonic day 6.5 (E6.5). A line of feather buds starts forming on each side of the dorsal midline of the bird. New rows are added bilaterally and progressively towards the flank over a period of about three days. These layers of feathers buds are organized in hexagonal arrays: each feather bud has six other feather buds around it, in a hexagonal shape (Inaba et al., 2019). This hexagonal lattice represents the most efficient packing and ordered packing on a 2-dimensional space. It arises because it is the closest possible positioning of elements when each element projects a circular inhibitory influence around them, whether mechanical or chemical (Ho et al., 2019). Two theories arise to explain how and why the feather buds follow such a distribution.
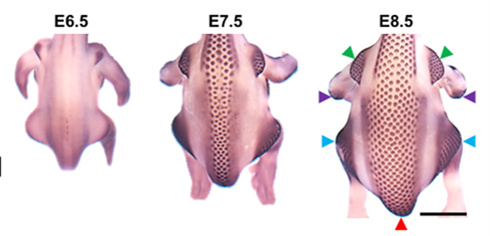
The first theory suggests a mechanical approach to the distribution of feather buds. During the embryo stage of the chicken or any other bird, the epithelium cells are mechanically unstable (Harris et al., 1984). The embryonic protective layer of skin cells is inherently and constantly in tension, as these cells are linked at intercellular junctions. At embryonic day 6, the dorsal region of the chicken embryo is relieved from tension and subject to compression (Shyer et al., 2017). These intervals of tension and compression form the whole pattern of feather buds that is observable at later stages of the embryo, where the feather buds, or feather primordium, consist of grouped epithelial cells from compression, mesenchyme cells rushing and aggregating underneath the bunched epithelial cells, and an arched membrane in between the two layers of cells (Shyer et al., 2017).
Further study shows that the dermis is responsible for the compression of the bird's skin: as the mesenchyme dermis cells push the membrane layer, it forces it to arch and results in the epithelium cells to group (Shyer et al., 2017).
Mesenchymal cells all have a potential to group together while increasing the potential of drawing other cells as the number of grouped cells rises. Then why do the dermis cells not aggregate into one large group? Experiments have shown that when mesenchymal cells grow on a stiff surface, the membrane layer for example, the resistance of the surface makes it such that only a limited number of dermis cells can aggregate, encouraging a dispersed array of grouped cells. The level of stiffness of the material has a direct influence on the pattern of the future feather buds that will appear (Shyer et al., 2017).
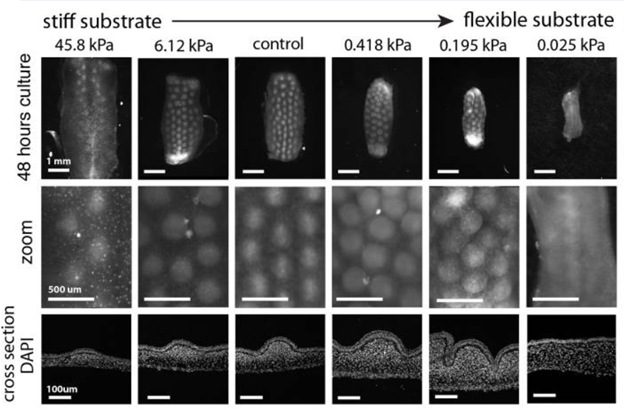
The second theory suggests interacting intercellular signals involving Turing reaction-diffusion patterns. As mentioned previously, the feather buds are implemented along the dorsal line by waves, with each feather bud being regularly spaced. These waves and the spacing between the feather buds are monitored by the presence of specialized cell signaling proteins. Studies have shown that three types of proteins play a major role in the patterning of feather buds on a bird's skin.
The first one is the bone morphogenetic protein (BMP), which are group cell signaling molecules. They are mainly known for inducing bone formation in most animals, but it has been shown to play a crucial role in all organs; mutations in the genes involved in BMP result in changes to the molecule that can be lethal or severely harmful to the animal (Wang et al., “Bone Morphogenetic Protein (BMP) signaling”). The second protein concerns the fibroblast growth factor proteins (FGF), which are also signaling molecules that send information to cells through FGF receptors. These proteins regulate a vast spectrum of biological functions, from cellular survival, migration, and differentiation to cellular proliferation. They are also further researched as they represent a potential for regeneration of a plethora of different body tissues, including skin, muscle, bone, nerves, blood vessel, tendon, ligament, cartilage and tooth. Any variation to the FGF protein could result in different genetic disorders at different levels (Yun et al., 2010).
The last one is the ectodysplasin A (EDA) protein, which plays an important role in the ectodermal development of cells in all animals. Research has concluded that EDA is crucial to the formation of hair follicles, scales, feather follicles in mammals, fish, lizards and birds (Yun et al., 2010).
Turing reactions-diffusions are chemical reactions where a molecule, classed as an activator, activates its own production while another molecule, classed as an inhibitor, represses the activator. Those molecules become heterogeneously distributed when the inhibitor diffusion is larger than that of the activator, forming periodic patterns. The activator can also initiate the production of the inhibitor, forming a self-regulating loop (Inaba et al., 2019).
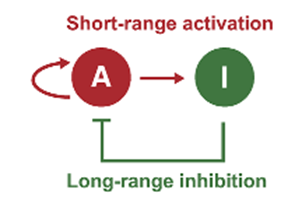
In birds, the feather follicle pattern is controlled by the feedback loop between two molecules: BMP which acts as the inhibitor and FGF which acts as the activator. FGF acts locally on the mesenchymal cells – initiating a condensation of cells – while BMP suppresses cell aggregation – working as a long-range inhibitor (Inaba et al., 2019). The feedback network of these two molecules presents the basic requirements for a Turing reaction-diffusion pattern. However, the patterns would be random and different from bird to bird, but a hexagonal lattice of feather buds can be observed consistently in all of them. Therefore, another molecule plays an important role in the patterning of feather follicles (Inaba et al., 2019).
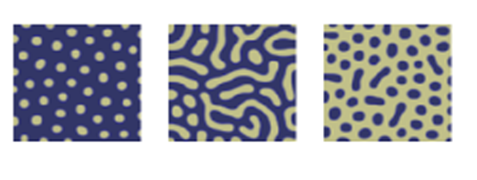
The formation of feather buds alongside the dorsal line of the bird is not random. Research has shown that a wave travelling bilaterally on the bird while interacting with the Turing reaction-diffusion patterns is what produces the highly ordered hexagonal feather bud array (Inaba et al., 2019). It is the expression and diffusion of EDA through the mesenchymal cells that form this wave. An initial threshold is required to induce the formation of feather buds.
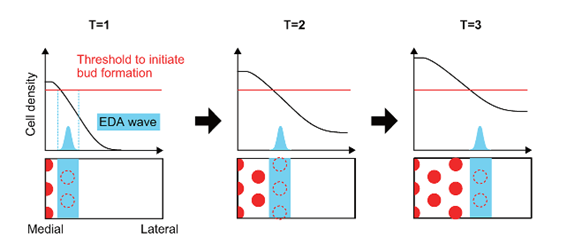
EDA first emerges in the dorsal line as a stripe covering the whole line. It then spreads bilaterally along the mesenchymal cells while inducing the expression of FGF proteins by interacting with EDA receptors found on the cells, which then initiates aggregation of cells and formation of feather buds. Beta-catenin molecules are expressed globally on the dorsal skin of the skin (Inaba et al., 2019). As the EDA progresses laterally, the expression of beta-catenin molecules changes spatially and becomes focused at precise locations, forming the observable hexagonal patterns. When EDA is upregulated, which means that its quantity is increased by cell stimulus, the area on which FGF expresses itself increases, forming larger feather buds. Inversely, it is smaller when EDA is downregulated (Inaba et al., 2019).
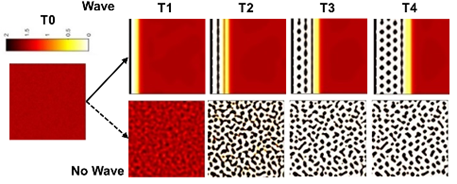
It is important to note that these EDA waves are not present in all birds. In fact, flightless birds like the emu or the ostrich lack these signalling waves. Voronoi tessellation analysis has shown that the feather bud patterns observed on flightless birds have a lower to no hexagonal distribution (Ho et al., 2019). Without the EDA wave to guide the feather primordium's locations, mesenchymal cells still aggregate, but do not follow a precise hexagonal distribution. This absence of hexagonal patterning for the flightless bird's feathers is due to the fact that a special arrangement of feathers required for flight is not needed (Ho et al., 2019).
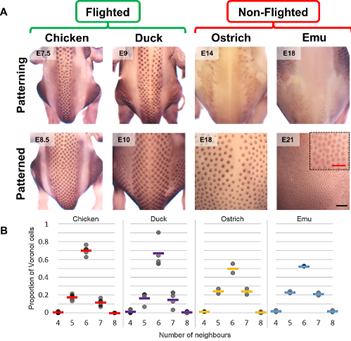
Feather Growth
Feather Bud Growth
A bird's skin is a composite tissue: like most animals, it consists of a protective layer of cells called the epidermis with a mesenchyme underneath, the dermis with a membrane layer in between. The epidermis – or more generally the epithelium, – which refers to the protective layer of cells lining internal or external surfaces of an animal, is a thin continuous sheet of cells (Ho et al., 2019). These are fixed together by their intercellular junctions, preventing an individual cell from moving. In contrast, the dermis forms a more elastic tissue. In the case of birds, during its early stage of development, a mesenchyme forms the dermis. The mesenchyme consists of mobile cells with unassigned functions. They form a liquid matrix and can move through it, under the epithelium (Ho et al., 2019).
The formation of feather buds is initiated by the grouping and condensation of dermal mesenchymal cells underneath a placode, a part of the epidermis which thickens. This forms a small bud on the skin layer, a feather primordium (Ho et al., 2019). These combined cells grow and form a feather follicle, from which a filament grows, and epithelium cells proliferate to form the rest of the feather, forming a down feather by the time the bird hatches: the small, almost fur-like, feathers that can be seen on a bird right after hatching (Ho et al., 2019).
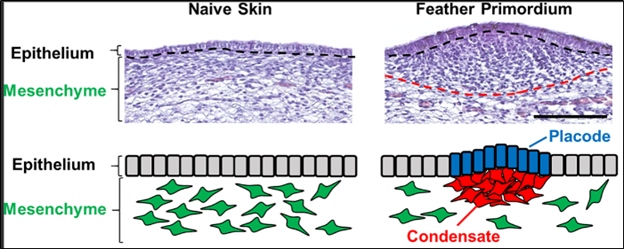
The exterior feathers, larger and more robust, follow the same principle to grow, but it starts at a later stage of the bird's life. These feathers do not last a lifetime. They follow a regenerative cycle that can be largely divided into a growth phase and a resting phase (Chen et al., 2015). During the growth phase, the feathers develop in the follicle similarly as the first feather formed by proliferation of epidermal cells. After varying amounts of time, depending on the bird's species, the bird enters a molting stage, where its feathers shed and cells in the follicle start preparing and proliferating to start a new cycle. This cycle specifically helps heal its feathers or it can unexpectedly bring new changes to it (Chen et al., 2015).
Branch Formation
Branching morphogenesis is a general mechanism that increases the surface area of an organ. Feather branching is an example of how periodic structures result from a reaction-diffusion mechanism during pattern formation. Cells accommodate the rapid formation of feather branches through the rearrangement of cell adhesion and changes in cell shape, and molecular signaling controls the patterning of the periodic feather branches (Cheng et al., 2018). Signaling molecules that regulate this process include BMP (Bone morphogenetic protein), Shh (Sonic hedgehog), Wnt which plays a central role in bone development and FGF (Fibroblast Growth Factor). For instance, interactions between Shh and BMP2 were suggested to be involved in feather branching morphogenesis (Cheng et al., 2018).
Particularly, the antagonistic balance between noggin and bone morphogenetic protein 4 (BMP4) has a critical role in feather branching – with BMP4 promoting rachis formation and barb fusion – and noggin enhancing rachis and barb branching. Furthermore, sonic hedgehog (Shh) is essential for inducing apoptosis of the marginal plate epithelia, which results in spaces between barbs (Yu et al., 2002).
In fact, the basal layer of the cylindrical epithelia of the feather filament forms periodic invaginations and segregates into alternating zones of cells destined for proliferation and death. Each valley becomes a marginal plate that will undergo programmed cell death, creating space between barbs. Each ridge becomes a barb ridge with bilaterally positioned barbule plates and centrally positioned axial plates (Cheng et al., 2018). Axial plate cells will eventually disappear to give space to opening barbules. The initial periodic patterning is triggered by activators and inhibitors that involve BMP signaling whereas the apoptosis of marginal plates involves Shh signaling as mentioned previously. At the follicular level, there is another round of apoptosis including pulp epithelia lying internal to the filament cylinder, feather sheath enclosing the filament cylinder, and barb generative zone located in the posterior follicle. Thus, feather branches open after the branching pattern is sculpted by programmed cell deaths (Cheng et al., 2018).
Feather branching morphogenesis produces different types of feathers: downy, bilaterally symmetric contour and bilateral asymmetric feathers. Downy feathers are radially symmetric, and the barb ridges lie parallel to one another (Cheng et al., 2018). These feathers are pliable and provide warmth to the bird. On the other hand, bilaterally symmetric contour feathers and bilaterally asymmetric flight feathers form a vane, producing a more rigid structure. The vane structure of these feathers allows for the movement of air, which is necessary for flight. The bilaterally asymmetric configuration of flight feathers provides improved aerodynamics (Chen et al., 2015).
The Role of Beta-Keratin
Beta-Keratin
Feathers are mostly composed of corneous beta-keratins, which are found exclusively in birds and reptiles. Keratin is an insoluble group of filament-forming proteins that are produced in particular epithelial cells of vertebrates. They protect the vertebrates from various external environments and provide mechanical support (Wang et al., “Keratin: Structure, mechanical properties, occurrence in biological organisms”). The alpha- and beta-keratin are the two types of keratins that are essential components in birds. In this paper, we will mainly focus on the characteristics and the structure of beta-keratin, which is only present in mature feathers.
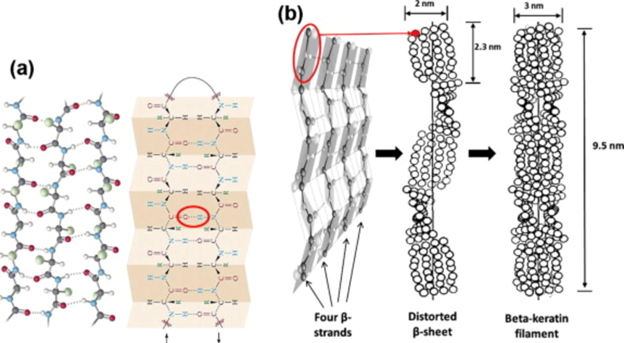
Different types of keratins can be classified based on their x-ray diffraction pattern (Fig. 11); alpha keratin will present an amorphous pattern whilst the beta-keratin will present a feather-pattern, also known as the beta-pattern. Furthermore, the internal supportive structures, such as the alpha-helices and the beta-sheets, can be used to classify keratins. One of the most notable characteristics of the beta-pattern is that it has an axial repeat of 0.31 nm reflection, while the amorphous pattern has a 0.515 nm spaced reflection (Schweitzer et al., 1999). The beta-keratin also has a 0.47 nm equatorial arc and 0.97 nm equatorial reflection. These are some common characteristics that are found in beta-keratins that makes them unique. Alpha- and beta-keratinous materials demonstrate a fine filament-matrix structure. The beta-keratin filament has a diameter of 3-4 nm and is what produces the feather pattern in beta-keratin. In feathers, a pile of filaments is oriented along the long axis of feather. Unlike the alpha-keratin, beta-keratin does not consist of several types of proteins and is only integrated into one single protein (Schweitzer et al., 1999). In a beta-keratin filament, one of the most significant features is the pleated sheet. The latter consist of laterally packed beta-strands that can be either parallel or antiparallel, and chains that are kept together by intermolecular hydrogen bonds. The hydrogen bonds between the strands along with the planarity of the peptide bond are what stabilizes the pleated sheet (Wang et al., “Keratin: Structure, mechanical properties, occurrence in biological organisms”).
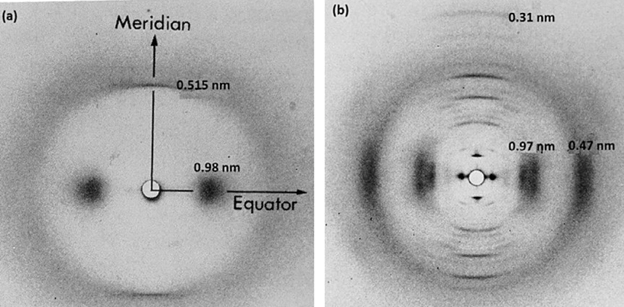
The intermolecular/intramolecular disulfide linkages and the interchain peptide linkages in keratin is what makes them insoluble. In general, beta-keratin is stiffer than the alpha-keratin and is the main component of avian claws and beaks, reptilian claws and scales, and feathers (Feroz et al., 2020).
Beta-Keratin in Feathers – Keratinization
As mentioned previously, the idea of keratinization and the two types of keratins is extremely important in describing the structure of the feather. Alpha-keratins are functionally diverse and are found in all vertebrates – being the primary component for numerous structures – whereas beta-keratins are only found in reptiles and birds and only found in mature feathers. In the feather development process, the relationship between morphogenesis and keratinization becomes very significant, since keratinization of each appendage depends on the earlier morphogenetic events (Haake et al., 1984). Alpha-keratins are displayed at the beginning of the developmental stage in the periderm and later in feather sheath. However, this presence starts to decrease significantly when the bird starts to hatch and when the down feather appears from the sheath. On the contrary, beta-keratins are demonstrated in the later stages of the developmental process (Greenwold et al., 2014). Initially, it is present only in the feather sheath. However, following barb ridge morphogenesis, beta-keratin will be found in the barb ridge, making up 90 % of the barbs and barbules. Overall, the variation of beta-keratin has an extremely important role in the formation of various types of feathers. This means that the beta-keratin displays an active and vital evolution of the avian lifestyle (Greenwold et al., 2014).
Beta-keratin can be grouped into four different subfamilies: claw, feather, scale, and keratinocyte beta-keratin whereas alpha-keratin can be divided into type I and type II. Comparative transcriptome analysis shows that 26 alpha-keratins and 102 beta-keratins are displayed in chicken scales and feathers during embryonic development (Greenwold et al., 2014). This proves that keratinization plays an essential role in the diversification of feathers and birds. Feather keratin – a type of beta-keratin – is especially crucial since research shows that it makes up to 85 % of the total number of beta-keratin in avian feathers (Greenwold et al., 2014). Beta-keratin, being the major protein building block of feathers, is what contributes to the strength of the epidermal appendages and to providing flexibility and elasticity to feathers.
When feathers start to form, the lower side of the follicle produces cells that will move upward and form the sheath that will grow outside the follicle. Later, beta-keratin filaments will be produced in the base of the feather, inside the follicle cell. Beta-keratinization happens gradually, meaning that it occurs in gradient, starting from the feather apex to the base and from the periphery of the barb ridge to the interior (Haake et al., 1984). As the feather beta-keratin polypeptides increase, the barb ridges will be paralleled. Alpha-keratins also exist during beta-keratinization. However, as the beta-keratinization proceeds and the feather differentiates, the alpha-keratin will lose its significance compared to the beta-keratin, like mentioned previously (Haake et al., 1984).
Color and Pigmentation in Bird Feathers
Colors and pigmentation in feathers have an important impact on camouflage, thermoregulation, mate choice, and communication. The colors we see in bird feathers come from two possible sources: nanostructures and pigments or a combination of both.
Beta-Keratin Nanostructure Formation Mechanisms
Many non-iridescent colors in feathers are the result of 3-dimensional, amorphous (quasi-ordered), spongy beta-keratin and air nanostructures found in the medullary cells of feather barbs. There are two predominant classes of nanostructures found in feather barbs: channel-type and sphere-type. The channel-type nanostructures consist of sinuous, interconnected, bicontinuous air channels and beta-keratin bars of similar widths and shapes (Fig. 12b). On the other hand, the sphere-type nanostructures are made up of amorphous, close-packing spheroidal air cavities, separated by beta-keratin walls and often interconnected by small air passages (Fig. 12d). There is also a recently discovered class of 2-dimensional medullary nanostructure that consists of amorphous bundles of parallel beta-keratin nanofibers in air, but we will not go into the details of this (Saranathan et al., 2012).
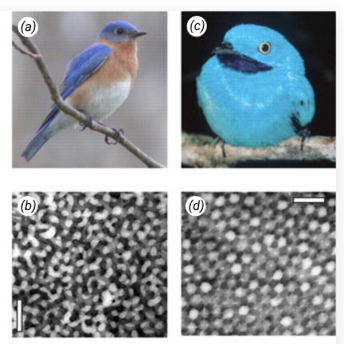
Prum et al. (2009) found that these amorphous medullary nanostructures form spontaneously without any underlying biological template or stencil of cytoskeletal fibers and membranes. They proposed that both channel and sphere nanostructures could be formed by the arrested phase separation of beta-keratin protein from the cellular cytoplasm. An essential process for the self-assembly of soft, condensed-matter systems, phase separation is where a mixture becomes unstable due to lower temperatures and intermediate volume fractions and unmixes via either spinodal decomposition (SD) or nucleation-and-growth (Prum et al., 2009). SD produces interconnected bicontinuous channels while nucleation-and-growth results in spherical droplets; these two processes are likely responsible for channel-type and sphere-type medullary nanostructures, respectively. However, this is not for certain. Additionally, Prum et al. (2009) studied the specific development of channel-type nanostructures in the feather barbs of Blue-and-Yellow Macaws. They found that during keratinization, drying of the feather cells creates capillary forces that seem to drive heavier molecules and materials to the cell's periphery. This results in peripheral aggregations of granular materials and a large volume of cytoplasm devoid of electron density in the center of the cell. The channel-type, beta-keratin nanostructures form spontaneously within these peripheral aggregations and coarsen and grow over time to completely fill the cell – likely through SD in the case of the blue-and-yellow macaw feather (Prum et al., 2009).
Nanostructure and Pigment Interactions
Many unique colors that we see in bird feathers are produced by a combination of both medullary nanostructures and pigments like melanin, carotenoids, and psittacofulvin. First, light passes through the pigmented outer cortex of a feather barb and a portion is absorbed by the pigment, creating a band-pass filter effect (Saranathan et al., 2012). Then, the remaining light travels to the underlying medullary nanostructure. Through evolution, these spongy keratin nanostructures have been tuned to constructively reinforce and scatter only a portion of the pigment-reflected light and to have longer wavelengths destructively interfere, reducing the broadband reflectance from the pigments (Saranathan et al., 2012). Finally, the light scattered by the nanostructures passes through the pigmented cortex again to leave the feather barb, resulting in the feather taking on a uniquely saturated, bright, and usually longer wavelength color. For example, this type of pigment-nanostructure interaction can produce saturated greens, yellows, and oranges that are unique from the spectrum of colors produced by pigments or nanostructures alone (Saranathan et al., 2012).
These nanostructures have applications in biomimicry. For example, there is ongoing research on producing colored films using naturally sourced biodegradable materials by imitating the nanostructures found in bird feathers (“Mimicking bird feathers to recreate nature's luminous colours” | Cordis EU Research Results, n.d.).
Pigment Patterning: Reaction-Diffusion Models
There are two types of pigments that contribute to the colors we see in bird feathers: melanins and carotenoids. Melanins produce a range of blacks, greys, browns, and oranges and are the predominant pigment found in most plumages. They are synthesized by special cells called melanocytes that work with feather follicles to finely control the pigmentation patterns within a feather (“How do birds get their colors?” | University of Chicago Press Journals, 2017). Meanwhile, carotenoids produce brighter color hues and are used in specialized feather structures. Birds cannot synthesize carotenoids and must consume the pigment, which then circulates through the bloodstream to the feather follicles. Afterwards, in a reaction unregulated by any cells, the specialized feather structures react with the carotenoids, altering them to produce different hues (“How do birds get their colors?” | University of Chicago Press Journals, 2017).
During the early development of a feather germ, melanocytes migrate into its tubular epidermis from the dermal pulp. The melanocytes' pseudopodia extend to reach the epidermal cells of the barb ridges and to transfer melanosomes (melanin-producing organelles) to developing feather keratinocytes, which take in the melanosomes through phagocytosis (Prum and Williamson, 2002). As the keratinocytes complete development and die, the melanosomes are incorporated into the cells' keratin, giving it colors. During these developmental stages, interactions between melanocyte pseudopodia and feather keratinocytes determine whether melanosomes are transferred into the keratinocytes (Fig. 13), further determining within-feather pigment patterns (Prum and Williamson, 2002).
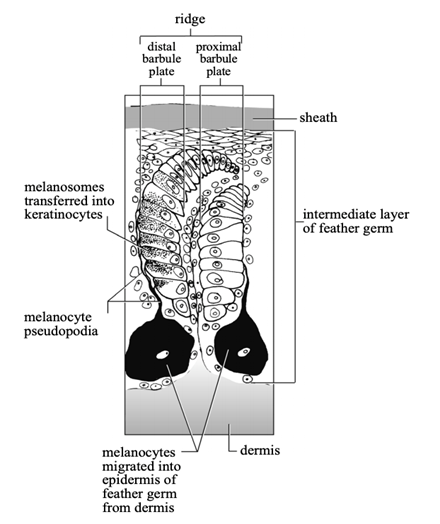
Prum and Williamson (2002) hypothesize that melanocytes and feather follicles can produce a large variety of ‘within' feather patterns through reaction-diffusion systems. As evidence, they simulated feather growth and within-feather pigment patterning using three reaction-diffusion models, which hypothesize a chemical morphogen – an activator – and inhibitors. Melanin transfer was determined by a critical threshold of stimulus from the activator at the same time and place of a keratinocyte in the follicle collar. The simplest model was an activator-inhibitor model, where the activator activates the production of both itself and the inhibitor, while the inhibitor inhibits production of the activator (Eq. 1a, Eq. 1b). This produced either spatial or temporal periodicity in the patterns, depending on the values of the parameters, as seen in Fig. 14a, b, c and d.
{∂a\over ∂t}=r_a ({a^2\over b}+b_a )-r_a a+D_a {(∂^2 a)\over (∂x^2 )}
(1a)
{∂b\over ∂t}=r_a a^2+b_b-r_b b+D_b {(∂^2 b)\over (∂{x^2}')}
(1b)
where a(x, t) and b(x, t) represent the activator and inhibitor concentrations, respectively. ba and bb are the independent production rates of the activator and inhibitor. ra and rb are the decay rates. Da and Db are the diffusion rates. These parameters remain constant during a simulation (Prum and Williamson, 2002).
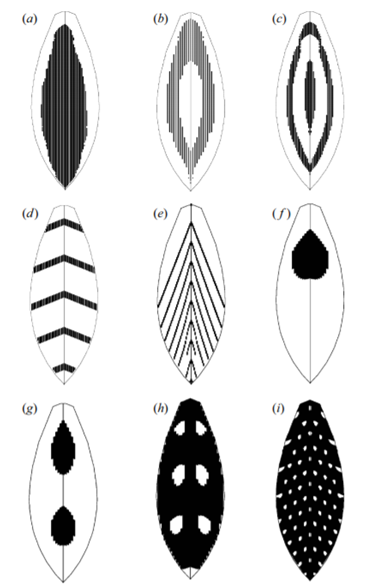
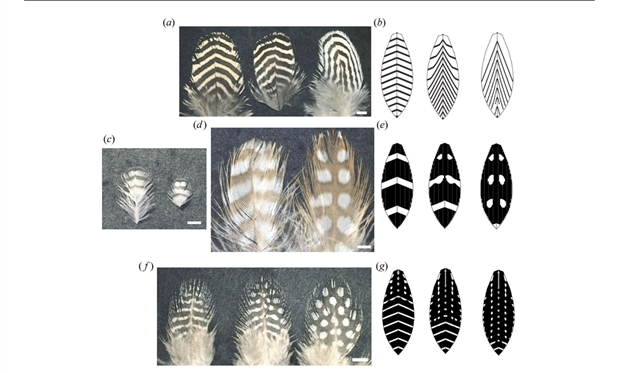
The second model had an activator deplete the substrate in an antagonistic interaction (Eq. 2a, Eq. 2b). This created the chevron patterns seen in Fig. 14e and Fig. 15b.
{∂a\over ∂t}=r_a b(a^2+b_a )-r_a a+D_a {(∂^2 a)\over (∂x^2 )}
(2a)
{∂b\over ∂t}=b_b-r_a b(a^2+b_a )-r_b b+D_b {(∂^2 b)\over (∂x^{2⋅} )}
(2b)
where all variables relating to a(x, t) are the same as in the first model. b(x, t) represents substrate concentration. bb represents the independent background production rate of the substrate. The rest of the variables for b(x, t) are the same except they are in reference to the substrate (Prum and Williamson, 2002).
The third model was an activator-two inhibitor model (Eq. 3a, Eq. 3b and Eq. 3c); one inhibitor causes stable patterns and the other causes periodic patterns. This produced more complex patterns of spatially and temporally periodic patterns overlaid together, as seen in Fig. 14f, g, h and i and Fig. 15c and e.
{∂a\over ∂t}={r_a\over c}*({a^2\over b}+b_a )-r_a a+D_a {(∂^2 a)\over (∂x^2 )}
(3a)
{∂b\over ∂t}={(r_b a^2)\over c}-r_b b+D_b {(∂^2 b)\over (∂x^2 )}
(3b)
{∂c\over ∂t}=r_c a-r_c c+D_c {(∂^2 c)\over (∂x^{2⋅} )}
(3c)
where all variables relating to a(x, t) and b(x, t) are the same as in the first model. c(x, t) represents the second inhibitor. rc and Dc are the decay rate and diffusion rate of the second inhibitor (Prum and Williamson, 2002).
These models were able to simulate common complex pattern transitions with simple changes in one or few parameters. They even had the ability to reproduce pattern anomalies seen in real birds where the anomalies are repeated identically in subsequent feathers grown from the same follicles. Overall, the models support the hypothesis that within-feather pigment patterning is a result of antagonistic interactions between chemical morphogens within the tubular follicle and feather germ.
Conclusion
As evidenced, feather formation is complex at all scales, involving many different cells, genes, molecules, and processes, from the formation of feather buds during the embryonic stage of the bird to the feather growth, branch formation, and pigmentation patterning that occurs every feather regenerative cycle.
One can note that Turing reaction-diffusion patterns are a common theme in multiple stages of feather development. During the bud formation, the initial feather buds appearing during the sixth day of the embryo follow a hexagonal distribution. In branch morphogenesis, reaction-diffusion patterns regulate the periodic placement of feather branches. Although it is not certain yet, the detailed pigment patterning of feathers is also likely a result of Turing reaction-diffusion systems. Beta-keratin is an outstanding protein fiber structure that is only found in reptiles and birds. In the case of birds, keratins are used to make the epidermal appendages strong and tough as well as making the feathers more flexible and elastic – as they are stabilized by hydrogen bonding between the beta-strands along the peptide-bonded backbone. Beta-keratins are demonstrated in the later stages of the developmental process, making up 90 % of the barbs and barbules. These processes and molecules come together to form a fully developed feather that can have different shapes, structures, and colors to suit the particular functions of that feather: from downy feathers that keep a baby chick warm, to the bilaterally symmetric contour feathers that are optimized for aerodynamic flight, to the colorful plumages used for interspecies communication.
References
(AAAS), A. A. f. t. A. o. S. (2020). PigeonBot's feather-level insights push flying bots closer to mimicking birds. Retrieved from https://www.eurekalert.org/pub_releases/2020-01/aaft-fi011320.php
Benton, M. J., Dhouailly, D., Jiang, B., & McNamara, M. (2019). The Early Origin of Feathers. Trends in Ecology & Evolution, 34(9), 856-869. doi:10.1016/j.tree.2019.04.018
Chen, K., Pittman, R. N., & Popel, A. S. (2008). Nitric oxide in the vasculature: where does it come from and where does it go? A quantitative perspective. Antioxidants and Redox Signaling, 10(7), 1185-1198. doi:10.1089/ars.2007.1959
Cheng, D., Yan, X., Qiu, G., Zhang, J., Wang, H., Feng, T., . . . Yue, Z. (2018). Contraction of basal filopodia controls periodic feather branching via Notch and FGF signaling. Nature Communications, 9(1), 1345. doi:10.1038/s41467-018-03801-z
Dieckmann, E., Dance, S., Sheldrick, L., & Cheeseman, C. (2018). Novel sound absorption materials produced from air laid non-woven feather fibres. Heliyon, 4(9). doi:10.1016/j.heliyon.2018.e00818
Feroz, S., Muhammad, N., Ratnayake, J., & Dias, G. (2020). Keratin – Based materials for biomedical applications. Bioactive Materials, 5(3), 496-509. doi:10.1016/j.bioactmat.2020.04.007
Greenwold, M. J., Bao, W., Jarvis, E. D., Hu, H., Li, C., Gilbert, M. T. P., . . . Sawyer, R. H. (2014). Dynamic evolution of the alpha (α) and beta (β) keratins has accompanied integument diversification and the adaptation of birds into novel lifestyles. BMC Evolutionary Biology, 14(1), 249. doi:10.1186/s12862-014-0249-1
Haake, A. R., König, G., & Sawyer, R. H. (1984). Avian feather development: Relationships between morphogenesis and keratinization. Developmental Biology, 106(2), 406-413. doi:10.1016/0012-1606(84)90240-9
Harris, A. K., Stopak, D., & Warner, P. (1984). Generation of spatially periodic patterns by a mechanical instability: a mechanical alternative to the Turing model. Journal of Embryology and Experimental Morphology, 80, 1-20.
Ho, W. K. W., Freem, L., Zhao, D., Painter, K. J., Woolley, T. E., Gaffney, E. A., . . . Headon, D. J. (2019). Feather arrays are patterned by interacting signalling and cell density waves. PLoS Biology, 17(2), e3000132. doi:10.1371/journal.pbio.3000132
Inaba, M., Harn, H. I. C., & Chuong, C.-M. (2019). Turing patterning with and without a global wave. PLoS Biology, 17(3), e3000195. doi:10.1371/journal.pbio.3000195
Journals, U. o. C. P. (2017). How do birds get their colors? The role of melanins in creating complex plumage patterns in 9,000 species. Retrieved from https://www.sciencedaily.com/releases/2017/08/170805142503.htm
Pittaway, R. (2000). Plumage and Molt Terminology. Ontario Birds, 18, 27-43. Retrieved from https://sora.unm.edu/sites/default/files/27-43%20OB%20Vol%2018%231%20Apr2000.pdf
Prum, R. O., Dufresne, E. R., Quinn, T., & Waters, K. (2009). Development of colour-producing beta-keratin nanostructures in avian feather barbs. Journal of the Royal Society, Interface, 6 Suppl 2(Suppl 2), S253-S265. doi:10.1098/rsif.2008.0466.focus
Prum, R. O., & Williamson, S. (2002). Reaction–diffusion models of within-feather pigmentation patterning. Proceedings of the Royal Society of London. Series B: Biological Sciences, 269(1493), 781-792. doi:doi:10.1098/rspb.2001.1896
Results, C. E. R. (n.d.). Mimicking bird feathers to recreate nature's luminous colours. Retrieved from https://cordis.europa.eu/article/id/413547-mimicking-bird-feathers-to-recreate-nature-s-luminous-colours
Saranathan, V., Forster, J. D., Noh, H., Liew, S.-F., Mochrie, S. G. J., Cao, H., . . . Prum, R. O. (2012). Structure and optical function of amorphous photonic nanostructures from avian feather barbs: a comparative small angle X-ray scattering (SAXS) analysis of 230 bird species. Journal of The Royal Society Interface, 9(75), 2563-2580. doi:doi:10.1098/rsif.2012.0191
Schweitzer, M. H., Watt, J. A., Avci, R., Knapp, L., Chiappe, L., Norell, M., & Marshall, M. (1999). Beta-keratin specific immunological reactivity in feather-like structures of the Cretaceous Alvarezsaurid, Shuvuuia deserti. Journal of Experimental Zoology, 285(2), 146-157. doi:https://doi.org/10.1002/(SICI)1097-010X(19990815)285:2<146::AID-JEZ7>3.0.CO;2-A
Shyer, A. E., Rodrigues, A. R., Schroeder, G. G., Kassianidou, E., Kumar, S., & Harland, R. M. (2017). Emergent cellular self-organization and mechanosensation initiate follicle pattern in the avian skin. Science, 357(6353), 811-815. Retrieved from https://science.sciencemag.org/content/sci/357/6353/811.full.pdf
Wang, B., Yang, W., McKittrick, J., & Meyers, M. A. (2016). Keratin: Structure, mechanical properties, occurrence in biological organisms, and efforts at bioinspiration. Progress in Materials Science, 76, 229-318. doi:10.1016/j.pmatsci.2015.06.001
Wang, R. N., Green, J., Wang, Z., Deng, Y., Qiao, M., Peabody, M., . . . Shi, L. L. (2014). Bone Morphogenetic Protein (BMP) signaling in development and human diseases. Genes Dis, 1(1), 87-105. doi:10.1016/j.gendis.2014.07.005
Yu, M., Wu, P., Widelitz, R., & Chuong, C.-M. (2002). The morphogenesis of feathers. Nature, 420, 308-312. doi:10.1038/nature01196
Yun, Y. R., Won, J. E., Jeon, E., Lee, S., Kang, W., Jo, H., . . . Kim, H. W. (2010). Fibroblast growth factors: biology, function, and application for tissue regeneration. J Tissue Eng, 2010, 218142. doi:10.4061/2010/218142