The Optical Properties of Camera Eyes
Tuna Gedik, Nassib Hassouna, Mohul Sharma, Michelle Sateen Yazbek
Introduction
Sight undeniably plays a crucial role in the lives of many organisms on Earth. Many species heavily rely on sight for their survival; vision provides to them the early warning stimuli signaling the presence of a threat. However, it also enriches our perception of our surroundings, giving it color, form, meaning, and depth. When looking at it in the simplest way, vision is achieved through the eye, followed by a long chain of neural connections spanning the space between the retinal receptors to the primary visual cortex (Koretz and Handelman, 1988). A highly sophisticated system, which will be elaborated upon in this paper, allows an image of the surrounding environment to be displayed on the internal layer of the eye, called the retina. In fact, the colors that we perceive are a direct result of the wavelengths of the electromagnetic photon wave-particles that hit our retinal receptors (Koretz and Handelman, 1988).
Many organisms have developed different ocular structures based on their respective needs for vision. Consequently, there exists many different types of eyes in the animal kingdom, some of which are schematically represented in Fig. 1.

This paper will focus on the camera-type eye, an ocular structure that is present in many species around the globe from widely different ecosystems. It is named as such due to its way of functioning, which resembles the mechanism of a camera, where a lens refracts light onto a retina full of photoreceptors (Koretz and Handelman, 1988). The latter capture photons and transform their energy into electrical signals. We, for example, possess this type of eye. However, cephalopods, such as squids and octopuses, have camera eyes as well (Sarah, 2016).
The topics that will be discussed in this paper include the evolution of the eye, the optics of lenses, the refractive nature of the camera eye lens, and focal length and accommodation. We will end with a presentation of relevant current and future biomedical applications of said knowledge.
The Evolution of the Eye
Two Big Questions
Two big questions remain only partially answered with various theories: When and how did eyes evolve? To many, eyes have an incredibly detailed mechanism that can, among other things, switch focus from a screen in front of us to a distant horizon in less than half a second (“Animal Eyes Inspire New Camera Technology” | Knut and Alice Wallenberg Foundation, n.d.). The structures required for these functions are so complex that some renowned scientists, such as Charles Darwin, admitted that the idea of the eyes having naturally evolved is absurd to a large degree (refer to Harvey, 2015 in Appendix). Nonetheless, many modern scientists agree that the complexity of the eye is, more than anything, a revelation of the immense engineering capabilities of nature. There is a widely accepted theory for how eyes may have evolved, the fundamentals of which will be explained in this section of the paper.
Complex multicellular life started becoming more prevalent during the Cambrian period. This is also when the first eyes appeared, around 541 million years ago, in a group of extinct animals called Trilobites (“Evolution of the Eye” | New Scientist, n.d.). The latter had eyes very similar to those of modern insects, which are known to have compound eyes (Fernald, 2007).
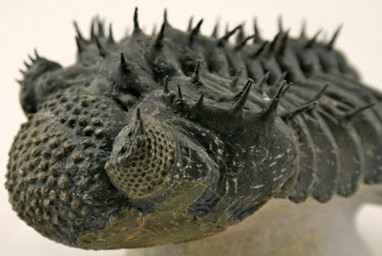
At the time, various single celled organisms had light spots, groups of light sensitive cells. An example of a currently living organism with such a light-sensing system is the euglena, a single-celled photosynthetic eukaryote. It possesses a light spot which allows it to sense where light is coming from. The reception of this information allows it to use its flagellum (a tail like structure) to propel itself towards light (refer to Harvey, 2015 in Appendix). However, in time, these light spots started to concentrate in certain areas and began to grow inwards, forming a pit. Compared to having a flat light spot, the curvature allowed the organism to better sense the direction of light (refer to Harvey, 2015 in Appendix).
As the pit got deeper in light-sensing organisms, they got better at analyzing changes in light of the surrounding environment, thus becoming capable of sensing movement. As can be viewed in Fig. 3, the opening of the pit also got narrower, letting less light in. This eye, called the pinhole eye, greatly increased resolution (refer to Harvey, 2015 in Appendix). For example, Planaria currently possess these kinds of eyes; this increases their chances of survival against predators (refer to Harvey, 2015 in Appendix). As transparent cells sealed off the entrance of the pit to protect the eye from the outside, the cornea formed. This allowed the transparent humor to form inside the cavity, increasing the sensitivity of the retina to light rays (“Evolution of the Eye” | New Scientist, n.d.).
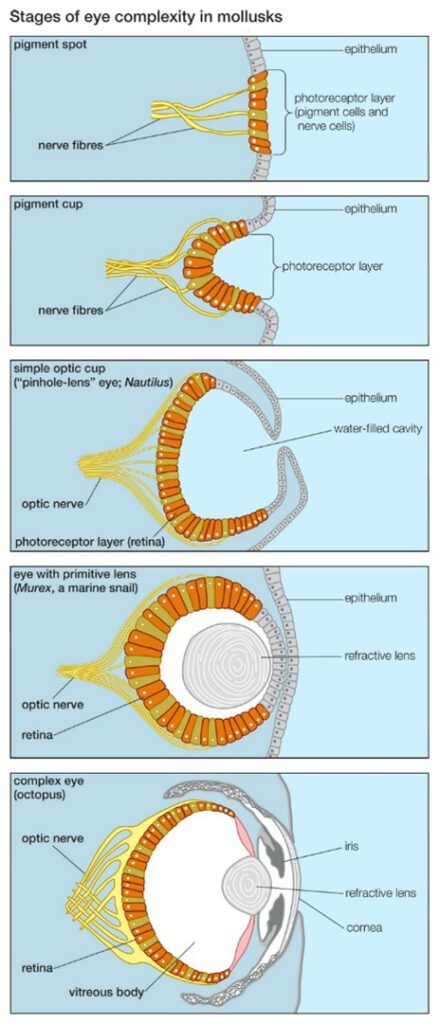
The next big step in the evolution of the eye was the formation of the lens. As the lens formed at the entrance of the eye, it allowed the eye to change the focal length to refract light so that it falls on the retina (refer to Fig. 3). This increased the effectiveness of the eye by a hundred times. Marine predators, called Cubozoans, have this kind of eye (“Evolution of the Eye” | New Scientist, n.d.). In time, more than ten different types of eyes evolved. At the same time, the brain evolved too, allowing more information to be processed at the visual cortex.
One commonly found type of eye is called the spherical lens eye, which is more commonly referred to as the camera eye. It has a single lens that refocuses light coming into the eye so that it falls on the retina correctly (Than, 2005). While the human eye works like this, differently developed camera eyes exist too. For instance, the Crampton's muscles (Fig. 4) in birds' eyes provide them the ability to adjust the shape of their corneas, increasing their range of accommodation (Ramel, n.d.).
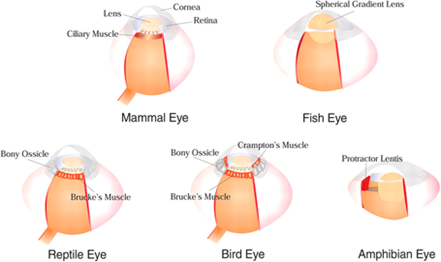
A fish species called the four eyed fish also has different camera eyes that evolved due to natural selection. Despite having two eyes, each of their eyes have two differently shaped pupils, divided by tissue (refer to Fig. 5). Since the four eyed fish spends its time floating on the top of water, the bottom cornea is adapted to see according to the refractive index of water, while the top cornea is adapted to the refractive index of air (Wambugu, 2019).

The traits of the camera eye can also be compared between different species as an example of convergent evolution (refer to Harvey, 2015 in Appendix). For example, despite evolving in different environments, vertebrate eyes and the eyes of cephalopods – molluscan marine animals like squids and octopuses – show traits of a camera eye. Both the vertebrate eye and the cephalopod eye contain the iris, circular lens, vitreous cavity, pigment cells, and photoreceptor cells. In both, the input from the light-sensitive retina follows the optic nerves to be processed in the occipital lobe (Serb, 2008).
However, the two have significant differences as well. The vertebrate eye is an outgrowth of the brain, while the eyes of cephalopods develop as invaginations of the surface of the body (Teboul, 2013). Thus, the cephalopod eye does not have the cornea. Another significant difference is in the way the cephalopod eye adjusts its focal length. While the vertebrate eye changes its focal length through the change in the curvature of the lens, the cephalopod eye changes its focus by moving the lens. The muscles surrounding the lens move the lens forwards, to the retina, and backwards, away from the retina (Teboul, 2013). This is similar to how cameras or telescopes focus.
Another difference between the structure of the vertebrate eye and cephalopod eye is the retina. The retina in the vertebrate eye is inverted. This means that all the photoreceptors face outwards from the eye (Fig. 6). As such, light must pass through layers of cells and neurons before arriving at the cones (refer to Harvey, 2015 in Appendix). Consequently, the optic nerves must cross the retina to connect to the back of the photoreceptors. Thus, photoreceptors cannot cover all the retina because there cannot be any photoreceptors at the point where all optic nerves enter the retina. This results in the formation of a blind spot (Fig. 6). Such a blind spot does not exist in the cephalopod eye because the retina is not inverted; photoreceptors face the eye opening, so the optic nerves can connect from the back without interrupting the retina (“18.5G: Convergent Evolution” | Biology LibreTexts, 2020).

Additionally, natural selection has allowed for the divergent evolution of the eye of species with significant degrees of ancestral commonality. For example, cats, who are night hunters, have evolved a reflective layer that can maximize the amount of light the eye can detect (“Animal Eyes Inspire New Camera Technology” | Knut and Alice Wallenberg Foundation, n.d.). Many other mammals do not possess this additional reflective layer, but it serves as a great adaptation to feline species as their survival partly depends on it. Interestingly enough, this reflective layer is what provides cats with the frequently observed glow in their eyes as well as excellent night vision.
The Optical Principles of the Eye
Passage of Light Through Convex and Concave Lenses
To study and grasp the concept of the camera eye, it is essential to have an understanding of the different types of lenses. In the concept of physics, a lens is described as a piece of convex or concave glass or plastic that is transparent (“OUR EYES WORK LIKE CAMERA'S!” | Discovery Eye Foundation, 2020). The latter has the property of focusing and refracting light in a certain manner.
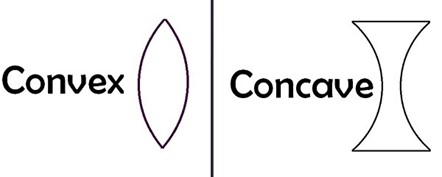
When light travels through a convex lens, it acts to merge the light rays at a specific point called the focal point. Physically, as it can be seen in Fig. 7, a convex lens is thicker in the center when compared to its edges. The image obtained from this type of lens is real and inverted (“OUR EYES WORK LIKE CAMERA'S!” | Discovery Eye Foundation, 2020).

On the other hand, concave lenses disperse the light rays going through them. They have an inward curve and are thinner at the center. The image obtained from the concave lenses is virtual and diminished (i.e., smaller) (Surbhi, 2018). As it will be explained later in the report, convex lenses are used to correct hyperopia while concave lenses correct myopia.
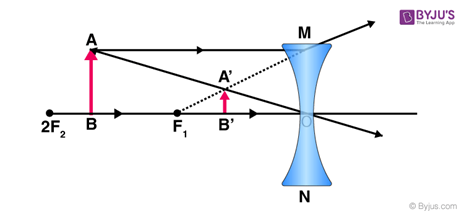
Path of Light Through an Eye
Digital cameras, similarly to camera eyes, require light to work. The latter travels in a straight line most of the time but it can also bounce off objects, which allows us to see them.
This whole process begins with the cornea which sits in front of the eye. It is a transparent and curved structure that works together with the lens to focus light onto the retina (Heiting, 2019). The aqueous humor is a liquid layer that is found right behind the cornea and its job is to maintain pressure levels in front of the eye as light is passing through. The light's path is then directed to the pupil, a round aperture that determines the amount of light that will pass through and reach the lens (Heiting, 2019).
When light passes through the cornea, the divergent rays are bent towards the lens where they are reflected for a second time before arriving at the retina. The curvature of the lens determines the distance between the eye and the object that the light is reflecting off as well as the distance to the retina on which the image is formed. Following this process, the lens stabilizes the focusing of the image with the help of specific muscles, called the ciliary muscles (refer to Harvey, 2015 in Appendix).
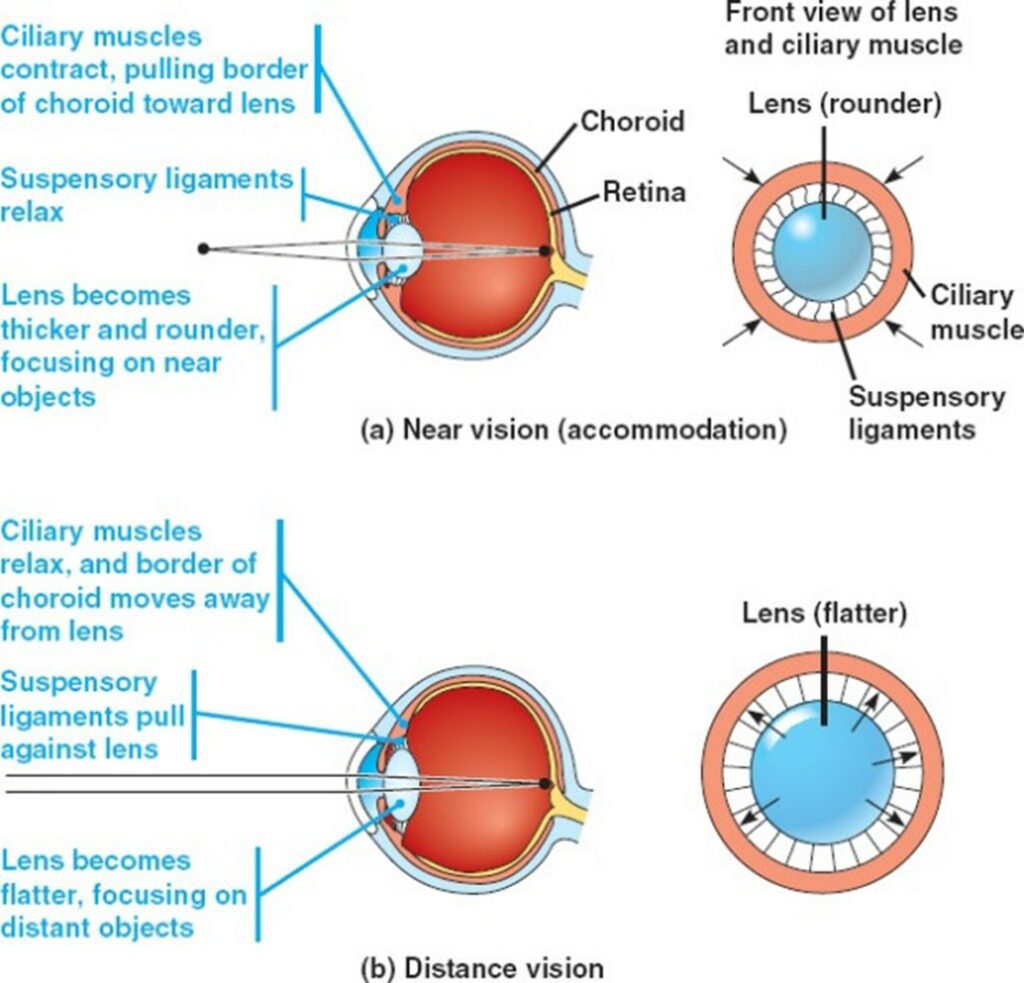
It is essential to note that the ocular lens has a unique ability: it can change its curvature. Therefore, the focal distance can be changed as well. This adjustment of the lens is also known as the process of accommodation. The lens is a flexible structure, and its curvature, via the zonules, is regulated by ciliary muscles. At a short focal distance, the ciliary muscle contracts, the zonule fibers relax, and the lens thickens, resulting in a rounder shape and thus high refractive strength (Sanderson, 2012).
Inversely, when changing focus to an object at greater distance, relaxation of the lens is required, which results in the increase of the focal distance. For both focusing and supplying almost the entire refractive power of the eye, marine animals must rely solely on their lens, as the water-corneal interface does not provide a substantial enough gap in refractive indices to have significant refractive power (Sanderson, 2012).
The light has yet to pass through another refractive medium, the vitreous humor, before reaching the retina. As explained above, since the lenses in camera-like eyes are convex, the image produced is inverted. If the process came to a stop at that moment, our whole world would appear upside down; but how do we turn this image right side up? The image must travel as impulses in the optic nerves, where it finally reaches a specific part of the brain, the visual cortex. When the image is formed in the brain, it interprets the image's orientation correctly and regains its proper perspective (“Physics of the Eye” | Lumen Learning, n.d.)
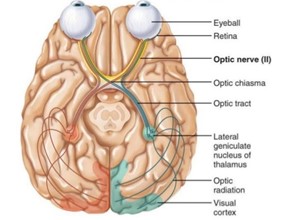
The Retina in Detail
To begin with, the central part of the retina is called the Macula. This structure is unique since it contains the largest number of photoreceptors compared to all the other parts of the eye. In fact, photoreceptors can be divided into two distinct categories based on their different functions: the cones and the rods (Turbert, 2019). The rods are the reason for the existence of our side vision and they also contribute to facilitating the process of seeing at night or when the lighting is dim. Moreover, they are located outside the macula and can even be found at the outer edge of the retina (Turbert, 2019). On the other hand, the cones are found inside the macula and their main function occurs when bright light comes through our eyes. At this moment, they provide detection of colors, sharp details, and a clear central vision (Turbert, 2019). The Retinal Pigment Epithelium (RPE) is also another component of the retina. The RPE has the function to absorb the extra light that is unneeded. It is a tissue layer located below the photoreceptors (“OUR EYES WORK LIKE CAMERA'S!” | Discovery Eye Foundation, 2020). Finally, the last part of the retina puzzle is the choroid, a dense capillary net located behind the retina. The only function of this component is to make sure that nutrients traveling from small blood cells reach the retina and the RPE (“OUR EYES WORK LIKE CAMERA'S!” | Discovery Eye Foundation, 2020).

The Refractive Nature of the Camera Eye Lens
The camera eye of many vertebrates and invertebrates is highly specialized to complete its primary function: refracting and focusing light onto the retina in such a way that the organism is endowed with a clear picture of its surroundings. Consequently, the camera eye must have a significant degree of optical power. From an optics perspective, the optical power of the eye, also called refractive power, is the degree to which it will converge light; it corresponds to the reciprocal of the focal length (Greivenkamp, 2004). In aquatic species, such as fish and squids, most of the eye's refractive power comes from the component known as the lens (Slingsby et al., 2013). In terrestrial animals, the refractive power of the lens is reduced, but it still plays a crucial role: combined with the ciliary body, it can adjust its optical power, and consequently its focal length, so as to keep focus on the image being viewed (Land, “The Optical Structures of Animal Eyes”). However, before we consider this process of adjustment, known as accommodation (Kaufman et al., 2003), we must understand the refractive nature of the lens, which constitutes the basis of vision as we know it.
The lens of the camera eye is of cellular nature; it is made up of many long, specialized cells, called fiber cells, that in the early evolution of the camera eye, got rid of their nuclei and mitochondria and packed themselves with highly specialized proteins known as crystallins (Goodsell, 2010). The sacrifice of removing these generally crucial cellular components provided a great pay-off to fiber cells: it increased lens transparency and suppressed light scattering, which would impair vision if it were to occur (Slingsby et al., 2013). Crystallin proteins consequently make up almost the entirety of fiber cells and are thus the primary reason for the lens' optical power.
Crystallin Proteins and Refractive Indices
The refractive index refers to the measure of the bending of light as it passes from one medium to another (Editors of Encyclopedia Britannica, “Refractive index”). As can be seen in Fig. 13, a certain material or substance will have its own corresponding refractive index and light will be bent following Snell's Law (Land, “The Optical Structures of Animal Eyes”):
n_1\sin(i_1) = n_2\sin(i_2)
(1)
It is thus clear that the greater is the difference in refractive indices of the mediums through which light is passing, the greater will be the degree of light refraction.
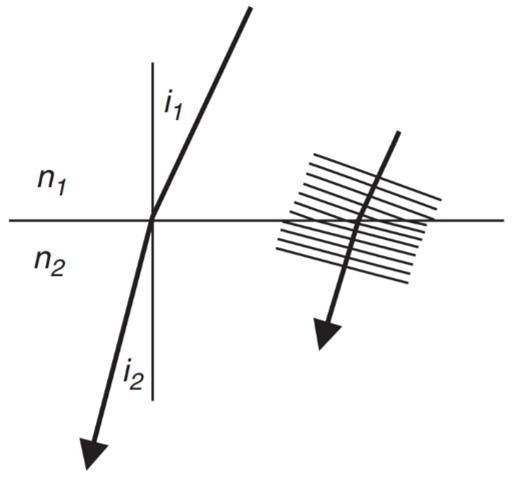
There are three primary types of crystallins, which are more-or-less ubiquitous in all species with camera eye lenses: α-crystallins, β-crystallins, and γ-crystallins (Slingsby et al., 2013). However, it is worth noting that the distribution of these crystallins varies from species to species, and many possess their own taxon-specific crystallin proteins (Zhao et al., 2012). For example, cephalopod eye lenses are primarily made up of S-crystallins (Tan et al., 2016). However, most crystallins share two common features. Firstly, as can be viewed in Fig. 14, they possess an unusually high molecular refractive index compared to the average protein (Zhao et al., 2012); this allows for a great degree of light refraction according to Snell's Law. Secondly, all crystallins have evolved from proteins with average refractive indices which initially had functions completely unrelated to light refraction in the eye. For example, α-crystallins are part of a family of heat shock proteins that play a role in cellular homeostasis (Slingsby et al., 2013). S-crystallins, on the other hand, are thought to have evolved from glutathione S-transferase, a phase II metabolic isozyme (Tan et al., 2016).

Crystallin proteins are present at very high concentrations in the camera eye lens; they are extremely soluble and thermodynamically stable so as to prevent crystallization and aggregation (Zhao et al., 2012). If such interactions between crystallin proteins were to occur, vision would be impaired due to light scattering in the lens and would result in the formation of cataracts. It is believed that crystallin proteins have evolved high refractive indices so as to avoid, as best as possible, such phenomena (Slingsby et al., 2013). By increasing the molecular indices of refraction of lens proteins, a proportionally smaller concentration of these proteins is required to provide a same amount of refraction (Zhao et al., 2012). By consequence to decreasing the concentration of proteins in the eye lens, the likelihood of aggregation and crystallization is decreased as well. This explains the highly unusual structure and amino acid composition of crystallin proteins: many possess a very small amount of alanine, threonine, valine, and leucine amino acids, while possessing a large amount of tryptophan and tyrosine (Slingsby et al., 2013). The formers possess a very low molecular refractive index increment compared to the aromatic amino acids, tryptophan and tyrosine (Slingsby et al., 2013). Through an increase in aromatic amino acids and a decrease in low refractive index residues, bodily proteins evolved to become highly sophisticated lens crystallins. Nature thus reengineered existing proteins to fulfill a role in the camera eye lens with more efficiency and less chance for error.
Spherical Aberration and the Graded Refractive Index
Most aquatic vertebrates and invertebrates possess spherical eye lenses, as these provide a compact design for an effective eye with a short focal length (Land, “The Optical Structures of Animal Eyes”). However, an eye of this shape has an inherent flaw known as spherical aberration. Spherical aberration, of which a diagram can be shown in Fig. 15, occurs when light rays passing through a spherical medium are not all bent to the same degree and consequently do not focus on the same point (Land, “The Optical Structures of Animal Eyes”).

Biological systems with spherical lenses have evolved a way of counteracting spherical aberration: a graded refractive index is created in the eye lens. Higher concentrations of crystallins with higher refractive indices are found in the center of the lens, called the nucleus, while lower concentrations are found near the periphery (the cortex) (Sweeny et al., 2007). Consequently, the refractive index of the spherical eye lens of aquatic species gradually decreases when moving outwards from the nucleus (Sweeny et al., 2007). Fish and cephalopods, which possess this type of eye, are thus able to view clear images underwater with spherical eye lenses possessing a very high optical power (Land, “The Optical Structures of Animal Eyes”).
Terrestrial vertebrates, including humans, also possess a graded refractive index in their camera eye lenses (Ji et al., 2012). However, as a significant percentage of the focusing power of these species is provided by the cornea, which is also made up of an arrangement of various crystallin proteins, they have also evolved a slightly hyperbolic-shaped cornea to further counteract spherical aberration (Land, “The Optical Structures of Animal Eyes”).
The Oddities of γ-Crystallins
Out of all forms of crystallin proteins, γ-crystallins are exceptionally unique with regards to their taxonomic distribution and subclass specializations. For starters, even amongst species having independently evolved these proteins, the refractive indices of almost all γ-crystallins are, on average, exceptionally higher than that of other types of crystallins (Slingsby et al., 2013) (refer to Fig. 14). Moreover, out of the three classes of ubiquitous proteins, α-, β-, and γ-crystallins, the distribution of γ-crystallins between the different phyla is the most inconsistent. Birds, for example, have replaced their γ-crystallins with a taxon-specific δ-crystallins (Chen et al., 2016). Moreover, many mammals, such as primates and guinea pigs, have lost genes coding for some γ-crystallin subclasses (Chen et al., 2016). Contrarily, fish lenses possess a large amount of a unique subclass of γ-crystallins, known as γM-crystallin, which not only has one of the highest known molecular refractive indices out of all known γ-crystallin proteins (Zhao et al., 2012), as can be seen in Fig. 14, but also has no orthologs in mammals (Chen et al., 2016). The following question naturally arises: “Why is there such an uneven distribution of γ-crystallins in the animal kingdom, and why is there such a large dichotomy between the degree of specialization of different γ-crystallin subclasses?”
Once more, nature has proven itself to be a formidable engineer. Recall from Snell's law (Eq. 1) that the degree of light refraction is larger when there is a greater difference between the refractive indices of the media through which light is passing. Consequently, due to the significantly larger refractive index of water compared to air, aquatic vertebrates require lenses with a very high refractive index, thus explaining the high prevalence of γM-crystallins in these species (Land, “The Optical Structures of Animal Eyes”). When vertebrates started to colonize the land, the high refractive indices of the lens were not only unneeded, but became detrimental to vision on land (Land, “The Optical Structures of Animal Eyes”). This kickstarted the transformation of the camera eye in terrestrial species: the cornea, which initially only served the purpose of protecting the fish eye, became an image-forming structure of its own (Land, “The Optical Structures of Animal Eyes”). Through natural selection began the progressive genetic suppression of various high refracting γ-crystallin genes in various phyla, as well as the production of many taxon-specific crystallins (Chen et al., 2016). The cornea thus became the primary light-refracting component of the terrestrial and avian vertebrate eye, while the lens became a secondary image-forming component with a softer structure to allow for the adaptive fine-tuning of vision, called the process of accommodation (Chen et al., 2016).
Focal Length and Accommodation
The focal length can serve as a measure for how strongly a given system will either converge or diverge light (Greivenkamp, 2004). As mentioned above, for an image to be formed in the brain, the light rays reflecting from that object must pass through certain parts of the eye and be projected on the retina. To have clear vision, light rays must converge on the fovea, the part of the retina with the most cones (Glasser, 2010).
Accommodation for Close Objects
Objects around us often do not remain static, nor do we. The eye must thus find ways of accommodating in such a way as to keep a clear picture of its surroundings. When an object gets closer, the light rays reflect on the eye at a greater angle from the object. Since the cornea refracts light rays to the same degree regardless of the distance, the rays do not diverge enough to fall on the fovea, which causes a blurred vision, as can be seen in Fig. 16 (“Eye Accommodation Made Easy” | Simple Science Answers | YouTube, 2013). To prevent this from happening, the focal length must be shortened. This job is done by the lens, which will further refract light rays to provide the eye with the correct focal length (Glasser, 2010). In a camera, the lens can be moved so that the image can be focused on the film. In the vertebrate eye, since the lens is unable to change its place, an evolutionary mechanism steps in (“Compare the Working of a Human Eye with a Camera Lens” | Knowledge Platform | YouTube, 2019).
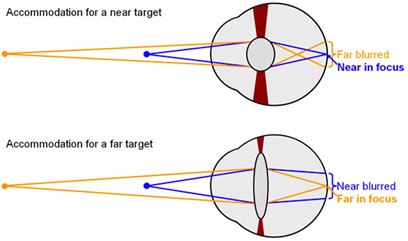
Around the lens lies a ring of muscles, called the ciliary muscles. The lens is attached to the ciliary muscles by the suspensory ligaments (Glasser, 2010). When the eye looks at a close object, the postganglionic fibers in the parasympathetic nervous system release a specific neurotransmitter known as acetylcholine (Ach) (Glasser, 2010). The M3 muscarinic receptors on the ciliary body respond to the released acetylcholine. As a response, the ciliary muscles contract, partially closing onto the lens (Glasser, 2010).
When the suspensory ligaments are tense, they pull the lens outwards, making the lens flat. The contraction causes the tense suspensory ligaments to loosen (Fig. 17). When the suspensory ligaments loosen, the lens loses its rigid shape, becoming rounder (“Eye Accommodation Made Easy” | Simple Science Answers | YouTube, 2013). This thick, round, unstretched form of the lens is much more convex compared to its previous stretched out form. This gives the lens a higher refractive power, bending light rays so that they fall on the fovea (Glasser, 2010).
Accommodation for Far Objects
When the object is far away, the light rays reflecting from the object come at a relatively small angle, almost parallel. As such, light does not have to be bent to a higher degree to fall on the fovea (Fig. 16). However, the round lens bends the light too much, blocking the light rays from landing on the fovea. To prevent this, the eye must increase its focal length (“Eye Accommodation Made Easy” | Simple Science Answers | YouTube, 2013).
To increase its focal length, the ciliary muscles relax. The relaxed ciliary muscles move outwards from the lens, causing the suspensory ligaments connecting the lens and the ciliary muscles to stretch out (Fig. 17). The tension in the suspensory ligaments result in the outwards pull of the lens. Consequently, the lens becomes flatter and thinner, losing its convexity (“Eye Accommodation Made Easy” | Simple Science Answers | YouTube, 2013). Due to the decrease in convexity, the refractive power decreases too. The initial refraction due to the cornea is enough for the light rays to fall on the fovea and produce a clear image when far away.
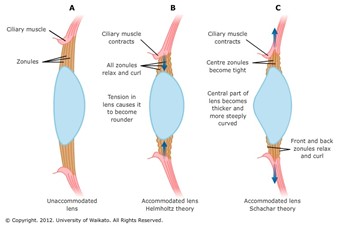
This process where the vertebrate eye changes its focal length to clearly see objects with varying distances is called the “accommodation of the eye” (Glasser, 2010). As described above, this occurs through adapting the curvature of the lens using the ciliary muscles. This theory, proposed by Hermann von Helmholtz in 1855, is the most widely believed theory explaining eye accommodation (Land, “Focusing by shape change in the lens of the eye”). In the following part of the paper, the failure to accommodate the eye and its consequences are discussed.
Biomedical Applications
Cataracts Disease
As mentioned previously, the lens of the camera eye is made up of fiber cells that have lost their nucleus and organelles to reduce light scattering. However, with this benefit also came a price: there is no protein turnover in fiber cells; proteins that have broken down and that are unable to complete their respective function(s) in the cell are not replaced and regenerated (Zhao et al., 2012). Crystallins therefore have to last the lifetime of an organism (Zhao et al., 2012). Unfortunately, many factors can cause the denaturation of crystallin proteins in the lens, the most common of which is age (Moreau et al., 2012). As an individual ages, crystallins are often the target of covalent modifications such as oxidation, deamidation, truncation, glycation and methylation (Sharma and Santhoshkumar, 2009). These changes can cause the partial unfolding of crystallins, and consequently the exposure of hydrophobic groups that usually lie in the inner, unexposed portions of the folded protein, due to the aqueous nature of the cytosol (Moreau et al., 2012). Hence, as more crystallins get affected by covalent modifications as an individual ages, these unfolded proteins will begin to aggregate towards one another, which clouds the lens and causes light scattering and vision impairment (Moreau et al., 2012). This phenomenon, of which a diagram can be viewed in Fig. 18, is known as cataract formation and is the major cause of blindness in the world (Sharma and Santhoshkumar, 2009). The leading solution to cataract-related vision impairment is through the form of cataract surgery, where the clouded lens is replaced by an artificial clear lens (“Cataract Surgery” | Fort Worth Eye Associates, n.d.). However, research is currently being done to find ways of preventing cataract formation. Notably, much research is being done to find ways of using phytochemical antioxidants derived from plants to prevent this age-related disease (Moreau et al., 2012), a field that bioengineers could undeniably greatly contribute to.

Myopia and Hypermetropia
There are two common eye conditions that result from an organism being unable to properly perform accommodation, which are respectively known as myopia and hypermetropia.
Myopia, also called nearsightedness, is a common condition that affects one's ability to see objects located far away from the eye. Those suffering from myopia will see far-away objects as blurred. Nearsightedness occurs when the shape of one's eye causes light rays to refract incorrectly; instead of focusing on the retina, the image forms in front of the retina. This is a result of the eyeball being longer than normal, or the cornea being curved too steeply (Fig. 19).
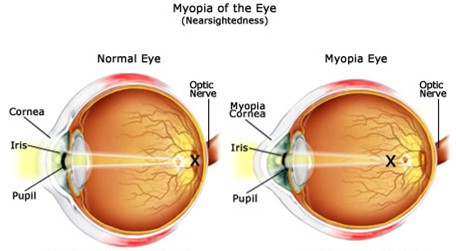
Hence, if the cornea or the lens is not evenly and smoothly curved, a refractive error appears, which manifests itself in the form of various symptoms, including blurry vision when looking at distant objects, the need to squint or partially close the eyelids to see clearly, headaches caused by eyestrain, and difficulty seeing while driving a vehicle, especially at night (“Nearsightedness” | Mayo Clinic, n.d.). This condition either develops gradually or rapidly, and often worsens during childhood and adolescence. Moreover, nearsightedness is theorized to be genetic. However, some studies also show that a lack of time spent outdoors could increase one's chances of developing myopia (Turbert, 2019).
Some people may also suffer from additional complications due to myopia, one of which is a reduced quality of life, as one may not be able to complete a task as efficiently and properly as one wishes. Also, uncorrected nearsightedness may cause you to squint your eyes to maintain focus, hence leading to eyestrain and headaches. Severe nearsightedness could put the patient at risk of retinal detachment, glaucoma, cataracts, and myopic maculopathy (Turbert, 2019).
Ophthalmologists call severe myopia high myopia. It is a more serious form of the condition, where the eyeball grows more than it is supposed to and becomes very long front to back. This extreme case of myopia usually stabilizes between the ages of 20 and 30 (Turbert, 2019).
Degenerative myopia, also called pathological or malignant myopia, is a rare type of nearsightedness. The eyeball gets longer very quickly, causing severe myopia. This type of myopia can get much worse far into adulthood, putting the patient at risk of having a detached retina, abnormal blood vessel growth in the eye, and glaucoma, which is a progressive eye condition that can lead to permanent blindness; it is caused by a buildup of fluids in the front portion of the eye, consequently increasing pressure in the eye and damaging the optic nerve (Seltman, “What is Myopia (Nearsightedness)?”).
Unlike myopia, hypermetropia is a common eye condition where nearby objects appear blurred, but your vision is clearer when looking at things from further away. Also called long-sightedness or hyperopia, it occurs when the axial length of the eye is too short, meaning that the eyeball itself is shorter than usual. One can also have hypermetropia if the cornea is flat, as it should normally be curved to properly direct light onto the retina. With this condition, the retina is closer to the pupil, causing the light to travel behind the retina (“Hypermetropia” | AIMU, 2017). Like myopia, hypermetropia is usually genetic. However, children with this condition tend to outgrow it when the eyeballs lengthen as they grow.

The degree of hypermetropia corrected by accommodative effort is known as facultative hypermetropia. Remaining uncorrected, hypermetropia is called absolute hypermetropia. Total hypermetropia after abolishing voluntary accommodation is known as manifest hypermetropia (the sum of facultative and absolute). With advancing age, accommodative efforts cannot be sustained, and hypermetropia becomes absolute till the effort of accommodation fails to correct any hypermetropia. Thus, facultative hypermetropia becomes abolished and there remains no difference between absolute and manifest hypermetropia (Bailey, 2020).
The symptoms of hypermetropia vary depending on the age of the patient and the severity of refractive error, as young patients are often cured by mild accommodative effort, without any symptoms. If not, the symptoms vary depending on the case. When hypermetropia is fully corrected, the patient suffers from asthenopia (eyestrain), frontal or fronto-temporal headache, watering, and mild aversion to light. In the case where hypermetropia is not fully corrected, the symptoms mainly are: asthenopia and defective vision. Another situation is when hypermetropia is high (more than 4D), where the affected only suffer from marked defective vision for both near and distant objects. Finally, if someone suffers from a spasm of accommodation, it may induce pseudo-myopia, which presents itself as an intermittent sudden blurring of vision. These symptoms could result from different causes, such as axial hypermetropia, curvature hypermetropia, index hypermetropia, positional hypermetropia, and the absence of a crystalline lens (Seltman, “Hyperopia (Farsightedness)”).
Medical and technological advances have developed various methods of helping a person suffering from either myopia or hypermetropia, the most common of which are wearable glasses and surgery. Also, there exists ways to slow or stop the progression of these conditions.
Eyeglasses are a simple, safe way to sharpen vision. Contact lenses are worn right on your eyes and have the same corrective effects as eyeglasses. Refractive surgery reduces the need for eyeglasses and contact lenses. This technique consists of using a laser beam to reshape the cornea, resulting in a decreased nearsighted prescription in the case of myopia. This method is usually used to treat nearsightedness but can also be used for mild to moderate farsightedness (“Farsightedness” | Mayo Clinic, n.d.). Also, this approach does not completely erase the need for glasses. The patient disposes of different surgeries: Laser-assisted in situ keratomileusis (LASIK), Laser-assisted subepithelial keratectomy (LASEK), and Photorefractive keratectomy (PRK). These procedures are not reversible, so one should be aware of the risks and consequences.
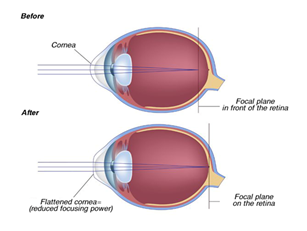
Researchers are currently continuing to search for less invasive and more effective approaches to stop nearsightedness from getting worse over time. For example, the topical medication, atropine, used to dilate the pupil of the eye, may help slow the progression of nearsightedness (“Nearsightedness” | Mayo Clinic, n.d.). Some scientists say that increased time outside may decrease the lifetime risk of nearsightedness. In addition, dual focus contact lenses have been shown to slow the progression of myopia in children between the ages of 8 and 12 (“Nearsightedness” | Mayo Clinic, n.d.). Lastly, orthokeratology is an approach in which the patient wears rigid, gas permeable contact lenses for several hours a day to even out the curvature of the eye (“Nearsightedness” | Mayo Clinic, n.d.). As can be seen, there remains much research to be done and technological advances to be made in the field of refractive error correction.
Conclusion
The camera eye has evolved in many vertebrates and invertebrates, piece-by-piece, to become the highly sophisticated structure it is today. Many terrestrial vertebrates, including mammalian and avian species, have evolved the ability to fine-tune their vision depending on the distance at which the object being viewed is located; this is known as the process of accommodation and is accomplish by a combination of muscles known as the ciliary body. The ciliary muscles connect to an ocular structure, called the lens, through ligaments known as zonules.
The lens is a structure made up of highly specialized fiber cells that are packed with crystallins. These proteins have a very high molecular refractive index, solubility, and thermodynamic stability, giving rise to a transparent ocular structure that allows for the effective passage and refraction of light. Consequently, the lens focuses light rays onto the retina: a thin, light-sensitive inner layer that is located at the rear of the eye.
In terrestrial vertebrates capable of accommodation, the lens is softer and capable of being stretched and compressed by the ciliary body, so as to adjust the refractive power and the focal length of the lens. Notably, when such an organism is focusing on an image from far away, the ciliary muscles relax, which stretches the zonule ligaments. This consequently stretches the lens, causing it to flatten and allow light rays to converge onto the retina. A similar, inverse process occurs when the organisms in question are viewing images from a close distance.
The eye remains, to this day, one of the most fascinating complex biological structures to have arisen as a result of natural selection. However, as are many other biological and man-made creations, it is not without its fault; refractive errors are very common in camera eyes, especially in those of humans. Through the understanding of the camera eye's structure and function, biomedical specialists have developed ways of counteracting cataract formation, myopia, and hypermetropia. However, much research remains to be done to develop more effective and easily implementable methods of reducing and preventing refractive errors.
Even amongst the large amount of research conducted 2020 in relation to the COVID-19 pandemic, biomedical researchers have done a great deal of progress to revolutionize our understanding of the eye and vision problems. Notably, at the Canadian 2020 Vision Summit was mentioned that “innovative approaches in gene therapy, stem cells, pharmaceuticals, and other fields are leading to major discoveries, and in some cases producing viable treatments for eye diseases” (“2020 Vision Summit” | Vision Research | Fighting Blindness Canada, 2020). Truly, bioengineers can have a formidable impact on future research and industrial advances in this field.
Appendix
“Compare the Working of a Human Eye with a Camera Lens.”, 19 February 2019, Knowledge Platform, YouTube, https://www.youtube.com/watch?v=xglvW0QPcVg.
“Eye Accommodation Made Easy.”, 20 February 2013, Simple Science Answers, YouTube, https://www.youtube.com/watch?v=JQNEc8H4oUE/.
Harvey, Joshua. “The evolution of the human eye.”, 8 January 2015, TED-Ed, YouTube, https://www.youtube.com/watch?v=qrKZBh8BL_U&t=1s.
References
(AIMU), A. I. M. U. (2017, 5 Dec 2017). Hypermetropia: Symptoms, Causes, Diagnosis, Management and Complications. Retrieved from https://www.aimu.us/2017/12/05/hypermetropia-symptoms-causes-diagnosis-management-and-complications/
Aquarium, V. (2014, 22 Jul 2014). The power of having four eyes. Retrieved from https://www.aquablog.ca/2014/07/featured-animal-largescale-foureyes/
Associates, F. W. E. (n.d.-a). Cataract Surgery. Retrieved from https://www.ranelle.com/cataract-surgery/
Associates, F. W. E. (n.d.-b). LASIK Eye Surgery. Retrieved from https://www.ranelle.com/lasik/
Bailey, G. (2020, Apr 2020). Hyperopia: What is farsightedness? Retrieved from https://www.allaboutvision.com/en-ca/conditions/hyperopia/
Biology, P. (2014, 31 May 2014). Understanding the Eye to Grade 9 at GCSE Biology (part 2) 2.91 2.92. Retrieved from https://pmgbiology.com/tag/ciliary-muscle/
Britannica, E. (n.d.). Eye. In: Encyclopedia Britannica. Retrieved from https://www.britannica.com/science/eye-anatomy#/media/1/199272/74661
Britannica, E. o. E. (2019, 23 Dec 2019). Refractive index. Retrieved from https://www.britannica.com/science/refractive-index
Chen, Y., Sagar, V., Len, H. S., Peterson, K., Fan, J., Mishra, S., . . . Wistow, G. (2016). γ-Crystallins of the chicken lens: remnants of an ancient vertebrate gene family in birds. FEBS Journal, 283(8), 1516-1530. doi:10.1111/febs.13689
Classes, B. S. (n.d.). Concave And Convex Lenses: Image Formation. In. byjus.com. Retrieved from https://byjus.com/physics/concave-convex-lenses/
Clinic, M. (n.d.-a). Farsightedness. Retrieved from https://www.mayoclinic.org/diseases-conditions/farsightedness/diagnosis-treatment/drc-20372499
Clinic, M. (n.d.-b). Nearsightedness. In. mayoclinic.org.
College, L.-B. C. (n.d.). Peripheral Nervous System In. cf.linnbenton.edu. Retrieved from http://cf.linnbenton.edu/mathsci/bio/jacobsr/upload/Nervous%20System%207%20-%20Cranial%20and%20Spinal%20Nerves.pdf
Fernald, R. (2007). Casting a genetic light on the evolution of eyes. American Journal of Ophthalmology, 143, 196-197. doi:10.1016/j.ajo.2006.11.010
Foundation, D. E. (2020, 13 Feb 2020). OUR EYES WORK LIKE CAMERA'S! Retrieved from https://discoveryeye.org/our-eyes-work-like-cameras/
Foundation, K. a. A. W. (n.d.). Animal Eyes Inspire New Camera Technology. Retrieved from https://kaw.wallenberg.org/en/research/animal-eyes-inspire-new-camera-technology
Glasser, A. (2010). Accommodation. In D. A. Dartt (Ed.), Encyclopedia of the Eye (pp. 8-17). Oxford: Academic Press. Retrieved from https://www.sciencedirect.com/science/article/pii/B9780123742032000361
Goodsell, D. (2010). Crystallins. Retrieved from https://pdb101.rcsb.org/motm/127
Greivenkamp, J. E. (2004). Field Guide to Geometrical Optics: Society of Photo Optical. Retrieved from https://books.google.ca/books?id=1YfZNWZAwCAC
Heiting, G. (2019). Refractive errors and refraction: How the eye sees. Retrieved from https://www.allaboutvision.com/en-ca/eye-exam/refraction/
Ji, S., Ponting, M., Lepkowicz, R. S., Rosenberg, A., Flynn, R., Beadie, G., & Baer, E. (2012). A bio-inspired polymeric gradient refractive index (GRIN) human eye lens. Optics Express, 20(24), 26746-26754. doi:10.1364/oe.20.026746
Kaufman, P. L., Alm, A., & Adler, F. H. (2003). Adler's Physiology of the Eye: Clinical Application: Mosby. Retrieved from https://books.google.ca/books?id=2YlsAAAAMAAJ
Koretz, J. F., & Handelman, G. H. (1988). How the human eye focuses. Scientific American, 259(1), 92-99. doi:10.1038/scientificamerican0788-92
Land, M. (2015). Focusing by shape change in the lens of the eye: a commentary on Young (1801) ‘On the mechanism of the eye'. Philosophical transactions of the Royal Society of London. Series B, Biological sciences, 370(1666), 20140308. doi:10.1098/rstb.2014.0308
Land, M. F. (2005). The optical structures of animal eyes. Current Biology, 15(9), R319-323. doi:10.1016/j.cub.2005.04.041
Learning, L. (n.d.). Physics of the Eye. Retrieved from https://courses.lumenlearning.com/physics/chapter/26-1-physics-of-the-eye/
Lee, L. P., & Szema, R. (2005). Inspirations from Biological Optics for Advanced Photonic Systems. Science, 310(5751), 1148-1150. doi:10.1126/science.1115248
LibreTexts, B. (2020, 15 Aug 2020). 18.5G: Convergent Evolution Retrieved from https://bio.libretexts.org/Bookshelves/Introductory_and_General_Biology/Book:_General_Biology_(Boundless)/18:_Evolution_and_the_Origin_of_Species/18.5:_Evidence_of_Evolution/18.5G:_Convergent_Evolution
Moreau, K. L., & King, J. A. (2012). Protein misfolding and aggregation in cataract disease and prospects for prevention. Trends in Molecular Medicine, 18(5), 273-282. doi:10.1016/j.molmed.2012.03.005
Ramel, G. (n.d. ). Bird Eyes: Exactly How Does A Bird's Vision Work? Retrieved from https://www.earthlife.net/birds/vision.html
Research, V. (2020). 2020 Vision Summit. Retrieved from fightingblindness.ca: https://www.fightingblindness.ca/vision-research-2020-vision-summit/
Sanderson, G. (2012). How the eye focuses light. Retrieved from https://www.sciencelearn.org.nz/resources/50-how-the-eye-focuses-light
Sarah, Maneet, Chelsea, Brennan, & Adrienne. (2016, 3 Apr 2016). How Is an Octopus Smarter than a Fifth Grader? Retrieved from https://biol1210.trubox.ca/2016/376
Schachar, R. (2012). Theories of eye accommodation. In. sciencelearn.org.nz: University of Waikato. Retrieved from https://www.sciencelearn.org.nz/images/54-theories-of-eye-accommodation
Scientist, N. (n.d.). Evolution of the eye. Retrieved from https://www.newscientist.com/definition/evolution-of-the-eye/
Seltman, W. (2020a, 23 Apr 2020). Hyperopia (Farsightedness). Retrieved from https://www.webmd.com/eye-health/farsightedness
Seltman, W. (2020b, 7 Feb 2020). What Is Myopia (Nearsightedness)? Retrieved from https://www.webmd.com/eye-health/nearsightedness-myopia
Serb, J. (2008). Toward Developing Models to Study the Disease, Ecology, and Evolution of the Eye in Mollusca. American Malacological Bulletin, 26, 3-18. doi:10.4003/006.026.0202
Sharma, K. K., & Santhoshkumar, P. (2009). Lens aging: effects of crystallins. Biochim Biophys Acta, 1790(10), 1095-1108. doi:10.1016/j.bbagen.2009.05.008
Sight, E. (n.d.). Myopia (nearsighted). Retrieved from https://www.everydaysight.com/myopia/
Slingsby, C., Wistow, G. J., & Clark, A. R. (2013). Evolution of crystallins for a role in the vertebrate eye lens. Protein Science, 22(4), 367-380. doi:10.1002/pro.2229
Surbhi, S. (2018, 8 Dec 2018). Difference Between Convex and Concave Lens. Retrieved from https://keydifferences.com/difference-between-convex-and-concave-lens.html
Surgicenter, T. E. C. (2015, 21 Sep 2016). Eye Surgery for Farsightedness. Retrieved from https://theeyeclinicsurgicenter.com/eye-surgery-for-farsightedness/
Sweeney, A. M., Des Marais, D. L., Ban, Y.-E. A., & Johnsen, S. (2007). Evolution of graded refractive index in squid lenses. Journal of the Royal Society, Interface, 4(15), 685-698. doi:10.1098/rsif.2006.0210
System, S. N. (2013, 21 May 2013). Vision. Retrieved from https://sensoryandnervous.wordpress.com/sensory/physiology/vision/
Tan, W.-H., Cheng, S.-C., Liu, Y.-T., Wu, C.-G., Lin, M.-H., Chen, C.-C., . . . Chou, C.-Y. (2016). Structure of a Highly Active Cephalopod S-crystallin Mutant: New Molecular Evidence for Evolution from an Active Enzyme into Lens-Refractive Protein. Scientific Reports, 6(1), 31176. doi:10.1038/srep31176
Teboul, D. (2013). Evolution of the Eye. Retrieved from https://danniteboul.wordpress.com/2013/11/12/evolution-of-the-eye/
Than, K. (2005). Nature Inspires Design of New Eyes. In. livescience.com. Retrieved from https://www.livescience.com/3914-nature-inspires-design-eyes.html
Turbert, D. (2019, 9 Apr 2021). Nearsightedness: What Is Myopia? Retrieved from https://www.aao.org/eye-health/diseases/myopia-nearsightedness
Wambugu, D. M. (2019, 9 Jan 2019). Four-eyed Fish Facts: Unique Animals of the World. Retrieved from https://www.worldatlas.com/articles/four-eyed-fish-facts-unique-animals-of-the-world.html
World, F. (n.d.). What is a Trilobite Retrieved from http://www.fossilworld.com/K1-tri/About.html
Zhao, H., Brown, P. H., Magone, M. T., & Schuck, P. (2011). The molecular refractive function of lens γ-Crystallins. Journal of Molecular Biology, 411(3), 680-699. doi:10.1016/j.jmb.2011.06.007