Taste in Birds
Karina Carlson, Rylee McDonald, Palmyra Mendoza Cabrer, Michael Weldon
Introduction
For a long time, it was agreed that birds did not have a sense of taste, or that if they did, it was minimal (Rowland et al., 2015). In fact, research into whether they even had taste buds only began 50 years after taste organs were described for fish, amphibia, mammals and reptiles (excluding crocodilia as they came later) (Berkhoudt, “Avian Taste Buds: Topography, Structure and Function”). Until then, it was perfectly intuitive that birds do not taste – a seemingly reasonable stance when considering that birds do not chew, but instead cram food down their throats (Reilly et al., 2001), in a motion similar to lapping, whilst chewing to release chemicals is so crucial to taste for other beings. Further, once explored, it was found that they have far fewer taste buds on their tongues than other vertebrates, and very limited expressivity while eating. They show some response to unpleasant taste, by shaking their heads or wiping their beaks for instance but indicate no behavioral distinction between neutral and delicious caloric intake (Rowland et al., 2015). It may be that there is just no advantage to communicating this sort of pleasure as other birds might come and consume the same resources if the first get too excited. Yet the answer could also be that a food's flavor may simply not matter as much to them – birds will prioritize quantity of intake over specific taste qualities (Rowland et al., 2015). So it indeed seems like taste may not be well – developed in birds. Yet, somehow, there is enough information to write a bioengineering report on the topic, so how can this be?
Well, it appears that birds' beak chemistries are incredibly diversified, just as their shapes are. Their diets cover a wide range, and so do the combinations of taste receptors different species have developed. Some birds are really picky, while others think appearances matter most. And the information they are receiving to make their decisions is not the same.
This paper will explore taste in birds through the general structure and connectivity of a bill and through some examples of gustation abilities in specific species. It will start with an analysis of each of the components of the bill, followed by an investigation into the gustation, chemesthesis and olfaction processes most common across bird species. Finally, it will explore taste preference and variance in the avian class for the key flavors of salty, bitter, sweet, sour, umami, calcium and fatty tastes, with a miscellaneous category for those that do not fit anywhere else.
Bill Structure and Connectivity
Birds have well-developed taste systems within their beaks. Only 5 % of birds' taste buds are found on their tongues (Rowland et al., 2015). In contrast, humans hold approximately 80 % to 95 % of their mouth's taste buds on the tongue. Bird species use different systems, with species having unique combinations of receptors and their distributions. Some are quite elaborate. Even chickens care what they eat, and they have more taste buds for the volume of their oral cavity than most mammals (Roura et al., 2013).
Bill
Before diving into the different taste systems, the basic structure of a beak should be made clear. Or rather, the bill – beak refers to the hard external surface (“Bird Beak Anatomy” | Chewy Editorial | BeChewy, 2011).
The beak acts like a fingernail does, serving as armor while also widening the scope of roles the protected element can perform – increasing precision, grip and rigidity. It is binded to the upper (maxillary) and lower (mandibular) jaw bones by a layer of connective tissues (“Bird Beak Anatomy” | Chewy Editorial | BeChewy, 2011). Both jaws are connected to the skull by kinetic joints that can move independently (Macwhirter, 2009). That makes up most of the bill's volume. The bones are fed blood through a vascular layer, which contains nerve endings, veins and arteries (“Bird Beak Anatomy” | Chewy Editorial | BeChewy, 2011).
Beak
The structure of the beak is made of keratin – the same material that makes up hooves and horns (“Bird Beak Anatomy” | Chewy Editorial | BeChewy, 2011). It can be quite tough – tough enough for the woodpecker's smashing and the macaw's crushing habits (Fig. 1). It is also constantly growing and being worn down (“Bird Beak Anatomy” | Chewy Editorial | BeChewy, 2011), similar to rodent teeth. The rates of wear vary from species to species. For instance, a hummingbird does not need to regenerate its beak as fast as a parrot, whose beaks grow between 2 to 8 cm a year (“Bird Beak Anatomy” | Chewy Editorial | BeChewy, 2011).
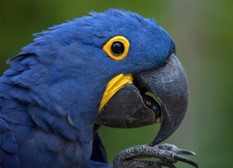
Palate
Moving to the back of the mouth, birds do not have a soft palate. Instead, the opening to the nasal passageway is a fringed V-shaped slit called the choana that closes when swallowing (Fig. 2). The choana can be seen in Fig. 2, papillae are abundant in this region (Macwhirter, 2009) and 70 % of birds' taste buds are found on the palate (Rowland et al., 2015).
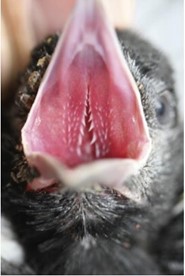
Nostrils
For most birds, the nostrils are located close to the head, on the sides of the beak (“Bird Beak Aanatomy” | Chewy Editorial | BeChewy, 2011). However, for foraging birds like ibises and kiwis they are found near the tip of the bill (Martin, 2017).
Tongue
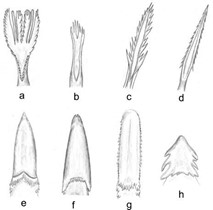
The lower beak — or mandible — often has a trench-like space where the tongue sits. This depression follows the bill shape, so birds have different tongue shapes (Erdoğan and Iwasaki, 2014). This feature is more of a constraint than it is a mold. To briefly generalize tongues, consider Fig. 3. These are all bird tongues, designed for flexibility and grip. For specialists in extraction (3a, 3b, 3c, 3d, 3f), such as woodpeckers (3d) who get insects from bark and hummingbirds who collect nectar from flowers, a long, narrow, protruding tongue with lateral barbs and spinous papillae at the tip is best (Erdoğan and Iwasaki , 2014). The narrowness means the tongue can get deeper while the texture increases surface area and thus extraction potential. Hummingbirds have an additional tube-shape feature to their tongues while honeyeaters and sugarbirds (Fig. 4) – who also collect nectar – opt for a brush-like feature (Fig. 3a and c) (Erdoğan and Iwasaki, 2014), scraping it up rather than sucking.
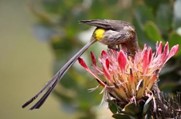
The rainbow lorikeet tongue, shown in Fig. 5, also works this way (“Lorikeet tongue: the strangest tool in the parrot world” | psittacology, 2020). Birds that are specialized for manipulating food (Fig. 3e, f and g) – food that might escape or that requires releasing from a shell – have tongues no longer than their beaks, covered in rigid, sharp papillae for grip. Ducks, chickens (Fig. 3e), penguins and vultures all have these tongues (Erdoğan and Iwasaki, 2014).
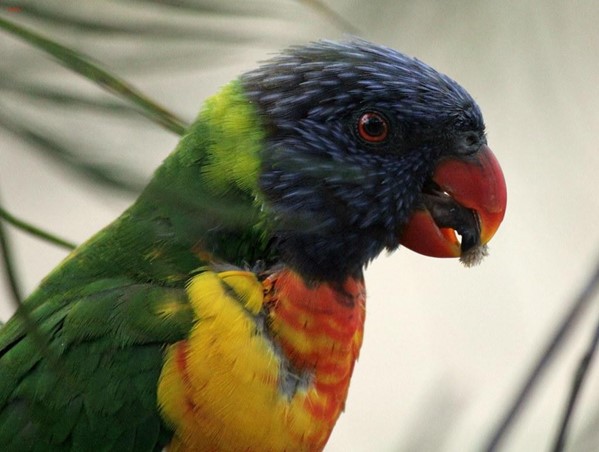
Ducks have hairs and spikes on theirs, which act as a sieve to filter food particles from the water. Meanwhile, penguins (Fig. 6), need so much grip to deal with their fish diet that their tongues are partially keratinized (“Let's talk about bird tongues” | Tough Little Birds, 2014), making their spikes extra unrelenting. The last category applies to ratite birds – the flightless ones, such as emus (h) and ostriches. Their tongues are small, relative to their bills – and they do not really use them (Erdoğan and Iwasaki, 2014). They eat through a “catch-and-throw” technique (“Emu's eating in slow motion” | CONTENTbible | YouTube, 2019), where the tongue presses against the bottom of the mouth, which widens the esophagus so the thrown food lands in the bill and can be swallowed directly (Erdoğan and Iwasaki, 2014).
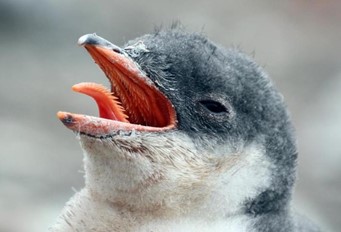
There is a further layer of complexity to bird tongues worth noting: their make-up. The human tongue is made up entirely of muscle, but bird tongues also contain a series of bones known as the hyoid apparatus which allows for added rigidity and flexibility. These bones are surrounded by fleshy, muscular padding. Close to the tip of the tongue, one bone branches into a Y-shape, giving a slight indentation that allows the bird to keep objects in place against their tongue while they manipulate them. All of this results in impressive tongue dexterity. To this effect, parrots — who use their tongue to explore their environment — can manipulate nuts with the dexterity of at least three human fingers ( “What's Inside Your Bird's Mouth” | Bird Tricks, 2013). It is important to acknowledge the diversity in tongues to understand the differences in taste between birds. How a bird gets food affects where – and whether – it needs to taste it. Each bill is its own system, and some are quite different. To close on tongues, birds' typically, but not always, come in grey, pink or black (“What's Inside Your Bird's Mouth” | Bird Tricks, 2013).
Taste Buds
Several receptor cells and ion channels, which all serve to diagnose compounds, are spread out across the oral and nasal cavities of the bill and extend into the throat. Depending on their type, they are connected to separate nerves and stimulate different areas of the birds' brains. This report will go more into detail about the function of these receptors shortly, but first comes a description of the anatomy of the most common – taste buds.
Mammal and bird taste buds have some significant differences. But while they are not exactly alike, the chemistry behind taste is similar. This section will briefly describe the taste buds of humans, to better understand those of birds, for whom the relevant research is scarcer. In humans, taste buds lie in the walls and groves of wart-like bumps called papillae, as seen in Fig. 7.
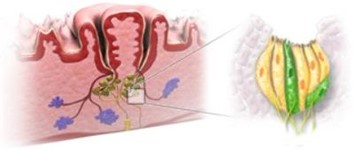
There are three types of papillae. Fungiform papillae are the most common, and contain sensory cells for taste, touch and temperature. Each fungiform papilla contains 3-5 taste buds. Circumvallate papillae are large, round, raised and visible to the naked eye. A person only has 7-12 circumvallate papillae, which contain several thousand taste buds. Follatile papillae can also be seen by the naked eye, the human tongue contains about 20. Each taste bud is made up of 10-50 sensory cells, and looks a bit like an orange, with the sensory cells as the wedges (“How does our sense of taste work?” | InformedHealth.org, 2016) (Fig. 8). Right above the taste bud is a small indentation filled with fluid. The purpose of this feature is to ensure that chemical substances can be detected and analyzed by as many sensory cells as possible, before being swallowed (“How does our sense of taste work?” | InformedHealth.org, 2016). At the opening of this indentation, sensory cells have finger-shaped sensory extensions called taste hairs, which can be seen in Fig. 9. For taste to be perceived, proteins in the surface of these hairs bind to specific chemical substrates. The sensory cell transforms this interaction with the taste-causing chemical substances into a nerve signal (“How does our sense of taste work?” | InformedHealth.org, 2016). As for all animals, this type of information is then carried along the cranial nerves to part of the lower section of the brainstem and travels to the part of the brain that is connected with sensory perception. Here, taste signals are connected to smell signals, so the brain has a full picture of what is going on (“How does our sense of taste work?” | InformedHealth.org, 2016). The other types of stimuli, such as smell, have their own receptors but the mechanisms and pathways are quite similar.
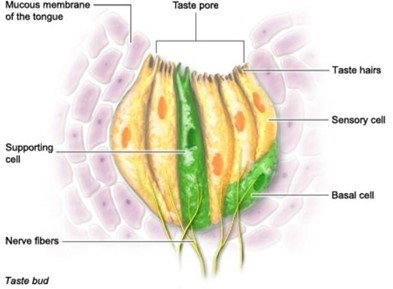
While humans have one structure of taste bud and three types of papillae, descriptions of bird taste do not discuss papillae, and research focuses instead on distinguishing between bud variations. Through shape and morphology, taste buds in birds can be separated into three distinct types (Rowland et al., 2015), but not all three appear in all bird species. The purpose of these variations has yet to be investigated, but it is agreed that they have different structures. Type I buds are egg-shaped structures that are made up of a central core of sensory cells and sustentacular cells, the latter provide support, while the former are responsible for detecting taste. This core is surrounded by follicular cells. Type I taste buds are mainly observed in songbirds, chickens, and pigeons (Rowland et al., 2015) Type II buds are narrower and more elongated than type I buds but have the same supporting and follicular cell types. Type II buds are observed in ducks and waders (like the Heron in Fig. 9). Type III buds resemble the buds of mammals, like humans, but they lack follicular cells. There is a taste canal that has been observed in chicken taste buds and does not appear in mammal taste buds. This taste canal acts as a tube, allowing communication of the sensory cells with the oral cavity (Rowland et al., 2015).

In mammals, taste buds appear predominantly on the tongue, in the taste papillae. This is because mammals use their tongue to position food in their mouth for grinding by their teeth. Birds, however, do not use their tongues as mammals do. The result of this is that less than 5 % of a bird's taste buds are found there, and these few are usually at the back of the tongue, because that is where the food makes contact with the tongue. In fact, 70 % of bird's taste buds lie on the palate. The rest appear somewhere close to the opening of the esophagus (Rowland et al., 2015).
Taste Reactions: Chemesthesis, Olfaction and Gustation
The general composition of the bill has now been established, along with an understanding of structural diversity between bird species. Moving on, it is time to explore how exactly birds can detect taste. This sense – gustation – is closely related to two other chemical senses: chemesthesis – stimulus caused by pain or irritation – and olfaction –smell.
Though these other two are not strictly part of taste, they certainly play a role in the feeding experience, and thus diet choice, so all three will be covered.
Chemesthesis
Chemesthesis – as it is known in the context of birds – is the sensation of pain during consumption (Barakate and Elwin, 2015). It translates to the subjective experience of “eww/yum; that's spicy.” The feeling can be induced by several receptors: TRPV1, TRPA1, TRPM8, TRPV3 and TRPV4 (TRPs are transient receptor potential channels, and the following letters and numbers refer to the subfamilies and the members of these respective subfamilies (Slack, 2016)). These may or may not be present in taste buds (scientists have yet to sort this out) and do not send signals to the same region of the brain as gustation experiences do (Roper, 2014). That is why they are different. Otherwise, they act similarly. When a specific compound is detected, the receptor receives/detects this information, and sends a signal to the brain, which induces the appropriate sensational response (Clark et al., 2014). This signal makes its way to the brain through different nerves depending on where the compound is detected in the mouth: the trigeminal nerve is for the front of the tongue, the palate, the tonsils, and the nasopharyngeal mucosa; the glossopharyngeal and vagal nerves are for the back of the bill. The pain caused by these irritants is beneficial to birds as it causes them to avoid consuming potentially harmful compounds (Slack, 2016).
Several arthropods have taken advantage of chemesthesis in birds. Ants of the genus Camponotus release the compound methyl-anthranilate as self-defense, which, in application, serves more to protect their kin. It triggers the trigeminal nerve in birds and makes them lose interest quite effectively (Conner et al., 2007). Chemesthesis is also relevant to birds in the form of capsaicin in chillies (Fig. 10).
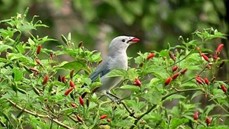
This time it is to their advantage. These plants produce visually appealing fruits but are very particular about who gets to eat them. Mammals do not; the flavor is aggressive and painful, but local birds – the superior seed spreaders – do, as they are insensitive to capsaicin (Tewksbury and Nabhan, 2001). Thus, the arrangement increases the distance seeds will travel, and reduces competition between snackers; it is a win-win for the non-furries. Chemesthesis overlaps with olfaction too, and creates uncomfortable sensations around evolutionarily – determined bad air. In one study, scientists exposed European starlings to anthranilate, a non-toxic compound they find aversive. The birds avoided it. The scientists then transected their olfactory nerves (so the starlings can no longer smell), and they still avoided the compound. After the chemesthesis-related nerve branches were transected, the birds did not react to the compound (Clark et al., 2014).
Olfaction
Olfaction is more effective at sensing odors though – and is really important to certain bird species. It informs the animal about the food in question without requiring direct contact, or even close proximity. Many species use smell to locate food. For example, turkey vultures detect a compound associated with decomposed carcasses, and black-footed albatrosses (Fig. 11) can respond to bacon grease over 31 km away (Clark et al., 2014) – all from the tiny odor compounds lingering in the air, far away from their source and passing through the bill's nasal cavity. There, receptors linked to the olfactory nerve dendrites can identify their nature and the bird can react (Clark et al., 2014). Smell is also actively involved while eating, and olfactory receptors are firing at the same time as those in taste buds are. At this time, olfaction complements gustation and serves no further purpose. At a different time – a time before the bird eats, per se, olfaction is influencing its development as a chick. Near the end of a bird's incubation period, exposure to certain odors influences its preference for these odors in its diet (Bertin et al., 2012). A chick will most likely show a preference for the smell of the food that its parents consumed while it was still in incubation.
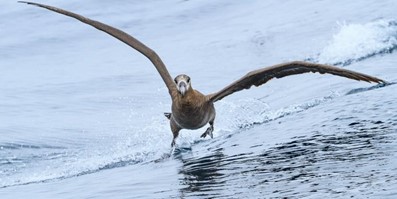
Gustation
The taste buds in birds are located mainly around the salivary glands of the palate, at the back of the tongue, and near the oropharynx (Clark et al., 2014). The compounds from the birds' food dissolve in their saliva and come into contact with the taste receptors found within the taste buds. Without saliva, these compounds would not make it to the taste receptors. Interestingly, birds' saliva has a higher flow rate than some mammals' saliva. This may act as compensation for the fewer taste buds found in birds' oral cavities (Rowland et al., 2015) as fewer receptors means that less signals being sent, but more saliva means more compounds are contacting the receptors, and thus more signals are being sent that way. For salty and sour tastes, the taste receptors are channel proteins in the cell membrane that are selective for sodium and hydrogen ions respectively. As for other tastes, the receptors are membrane proteins that interact with these compounds (Fig. 12). In both cases, the interaction of the compounds with the proteins causes electrical changes that lead to chemical signals being sent to the brain (Smith and Margolskee, 2001). These signals make their way through the facial nerve, the chorda tympani, the palatine, and the glossopharyngeal nerve (Rowland et al., 2015).
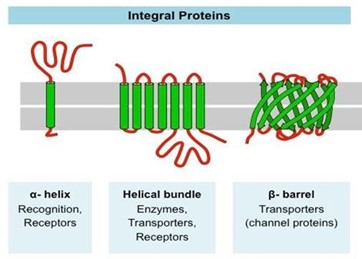
Taste and Taste Across Species (Which Can Detect What, and Why)
Though bird species are utilizing the same chemistry principles when consuming food, they also technically use the same physics principles for acquiring it, which (Carlson et al., 2020) – it is the same in this case. The specific compounds a bird is sensitive to depend on its diet. Allocating resources towards receptors that will not be activated is not evolutionarily clever, so for taste in birds, less is more – to an extent. Often, it is an advantage to taste a particular flavor so that the bird can consume more of a necessary nutrient, or so it can avoid a harmful one. Sometimes, it is more helpful to be impervious to a compound as to not be distracted or deterred – like in the case of capsaicin. In the section Taste Reactions: Chemestesis, Olfaction and Gustation of this paper, the effects of perceived spice through chemesthesis were explored. Now, the report will cover some of the relationships between birds and other classic flavors.
Sweet
As it turns out, humans are not the only ones with a sweet tooth. In fact, most vertebrates possess Tas1r2 and Tas1r3 genes which combine to form receptors that bind to sugars and other sweeteners (Treesukosol et al., 2011). Given that the diets of many avian species consist of nectars, fruits and grains, which are high in starch, these feeding behaviors were studied extensively. The components that make up most of their diets contain high concentrations of sucrose, glucose and fructose. Thus, it was believed that many birds have a preference towards a sweet taste and similar gustatory response to humans. However, data collected on the genome sequences of chickens, turkeys and zebra finches shows that all three species lack the Tas1r2 sequence that encodes the sweetness receptor, or one that is homologous to it. Homologous, meaning a gene sequence that will result in a protein, which fulfills the same function as the one encoded by the Tas1r2 and Tas1r3 in humans. For many researchers, this did not come as a shock, given that studies have observed that many birds lack behavioral patterns in terms of dietary preferences (Rowland et al., 2015). However, they continued their investigations by examining species who survive primarily off nectar, a highly sweet substance containing large amounts of disaccharide sucrose, as well as hexose monosaccharides, and pentoses such as glucose, fructose and xylose – all of which are chemical names for sugars.
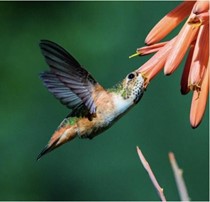
Naturally, hummingbirds (Fig. 13), were the ideal test subject for many avian taste perception studies. Some revealed that hummingbirds are not an exception; they too lack the Tas1r2 gene sequence that is fundamental for sweet taste detection. Yet, they do have the Tas1r1 and Tas1r3 sequences, which are generally responsible for savory taste. It was thus theorized that, throughout evolution, their Tas1r1 and Tas1r3 gene sequences resulted in a protein that adapted to distinguish sweet. A study was developed – in an attempt to confirm this hypothesis – in which researchers examined the response of hummingbirds, chickens and swifts to sugars and amino acids by assessing the response of the birds' Tas1r1 receptors in heterozygous cells. This was done by using calcium-sensitive photoprotein reporters, put more simply fluorescent dyes, to detect amino acid concentrations within the cells (Toda et al., 2011). Several carbohydrates such as sucrose, fructose and glucose were detected in the cells of hummingbirds, along with sucralose and sugar alcohols: sorbitol and erythritol, but only when Tas1r1 and Tas1r3 worked together as a heterodimer (a protein composed of two proteins). On the other hand, Tas1r1-Tas1r3 heterodimers in chickens and swifts failed to detect any concentrations of carbohydrates or sugar alcohols, instead recognizing only alanine and serine sweet amino acids. Therefore, the researchers concluded that the hummingbirds' ability to detect such a large array of sweeteners, without the Tas1r2 gene sequence must be the result of small changes over millions of years in their Tas1r1- Tas1r3 heterodimers (Baldwin et al., 2014).
It is intuitive to think that birds who dine on delicious syrupy fruits do so knowingly. Yet, hypotheses need confirmation to become facts. Species of tanagers (Fig. 14) and manakins (Fig. 15) were examined, as their diets are made up of approximately 8 % to 12 % sugar, primarily from tropical tree fruits. Birds from northeastern Costa Rica were kept in captivity over 5 months and offered two identical meals at the same time, but with different sugar contents. Each was equally accessible, and the contents eaten from each of the dishes was measured after each feeding.
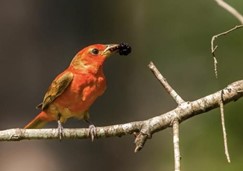
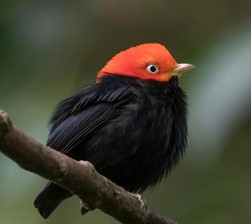
Throughout the trials, it was determined that tanagers preferred the higher sugar content diet, while manakins were, surprisingly, more or less indifferent to sugar level variance. This is likely due to the fact that tanagers crush their fruits, releasing the juices to coat their tongues while manakins swallow fruits whole and so they will not experience the sweet taste to the same extent (Levey, 1987). Although research indicates that some birds can taste sweetness, most cannot.
Salty
Something not particularly well known is that goats love salt and are known to lick natural salt deposits as a tasty snack. Birds, like goats, enjoy low concentrations of sodium in their diets, but are repelled when it is in excess. Their enjoyment of salt is likely an adaptation, as salt has many beneficial qualities to a bird's health and survival. Salt can aid in water retention, which can prevent dehydration. It also plays a surprisingly crucial role in nerve and muscle function, in balancing fluids, and in regulating blood pressure. Generally, birds will seek out salt when they are running low; signals in the nervous system will then cause them to crave it. In comparison, humans crave salt regularly, not only when their body is in need of it (Leshem, 2009).
In terms of dietary behavioral patterns and taste studies among birds, chickens have been the popular subjects of choice given the potential impacts on the poultry industry. Thus, much of this section will reference studies done on chickens.
The first of many examined, was one conducted on a group of chickens displaying a sodium deficiency and aimed to test the similarity between bird and human salt cravings. The number of taste buds in chickens is only 25 times smaller than humans, even though their oral cavity is 200 times smaller. This lessens the difference between chickens' and human's taste abilities, given that cavity size should be proportional to the number of taste buds. Consequently, the results of the study suggested that they are similar, as the chickens unanimously opted for solutions with medial concentration of NaCl when provided with options. They were confined in a pen with multiple solutions of high and low sodium concentrations for drinking, and their following behaviors were observed. However, upon further examination of the anatomy of the chickens, the researchers concluded that chickens' taste abilities differ from humans, given the presence of nasal salt glands (like that of the seagull in Fig. 16).
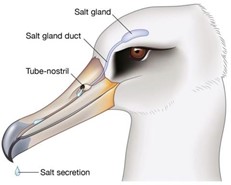
Further, to birds without these glands, extremely high concentrations of salt are toxic (Roura et al., 2013). The nasal salt gland secretes a saline solution that is reflective of the sodium content in the bird's blood, given that its function is to secrete excess salt to compensate for their less efficient kidneys. On its course out of the bird's body, the solution is directed through the taste buds, and the bird's cravings adjust accordingly (Braun, 2015). It is excreted similarly to drool, so any excess salt lingers in the mouth. The receptors allowing birds to taste salt are thought to be epithelial cell sodium ion proteins, meaning salt detection will result in an increase or decrease of activity of sodium ion channels. These channels work in contrast to nasal salt glands (as in Fig. 18), by regulating salt reabsorption into the body (Roura et al., 2013).
Sour
Sour taste is dependent on acidity, usually caused by bacterial fermentation. More often than not, sourness – an aggressive flavor – results in an initial rejection or dislike for the taste due to sensitivity. Sensitivity, however, generally decreases with age among avian species. Young birds are often frightened or immediately startled by the taste. To examine the avian response to sourness, chickens were chosen once again as test subjects. This time though, they were presented with acidic and alkaline solutions of a range of moderate alkalinity and acidity to some more extreme concentrations. The chickens were tolerant of the more moderate concentrations but avoided those of extreme pH values. Sourness is one of the few tastes where there is too little information to determine whether sour taste in avian species is more based on preference or necessity (Roura et al., 2013). Birds detect sour tastes thanks to transmembrane channel receptors which are selective for hydrogen ions, several of which have also been identified in mammals. This may support the idea that sour taste among birds is based on flavor, but there is not yet enough evidence to confirm, nor disprove this hypothesis (Roura et al., 2013).
Bitter
Like for sour lemons, birds have a similar reaction to humans for bitter medication. That is, quite an unpleasant one – they will move their tongue and beak and will shake their head in response to it (Macwhirter, 2009). The bitter taste is extremely important in birds because many toxins commonly used as a prey defense mechanism taste bitter. Birds have evolved to recognize that taste as harmful, which is why prey and even medicine that is bitter tasting is avoided or rejected by birds (Skelhorn and Rowe, 2010). Even more impressive is that birds can learn to associate bitter taste with different levels of toxin to make sure they keep their consumption of toxins low and to optimize their nutrient intake (Skelhorn and Rowe, 2010).
Many animals possess defensive toxins, which they advertise with color, sounds and odors (Skelhorn and Rowe, 2010). The idea of this strategy is that predators will remember a bad experience when they see, hear or smell the warning signs and will therefore avoid eating that organism. An example of warning coloration is the poison frog, which can be seen in Fig. 17. But these types of prey are not entirely off limits. Completely rejecting bitter-tasting prey would reduce a bird's opportunities for gaining nutrients. When consuming prey with toxins, there is always a tradeoff. It is profitable for birds to be able to tell the toxin concentration so they can consume the nutrients of these prey whenever possible; the distastefulness of bitter- tasting toxins often increases as toxin concentration increases (Skelhorn and Rowe, 2010).
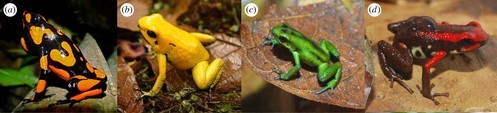
The European starling, pictured in Fig. 18 has a strategy to get the most nutrients, by weighing the pros and cons of eating certain prey, i.e., nutrients versus toxins. In an experiment, mealworms were injected with different concentrations of quinine solution. Quinine is a distasteful toxin that causes vomiting in humans and birds. Starlings can detect its effects after ingestion and learn to associate variation of bitter taste with different levels of toxin and choose to eat in a way that they get lots of nutrients and a small portion of toxins. So starlings can actually learn the relative toxicity of distasteful prey (Skelhorn and Rowe, 2010). If prey falsely advertises their level of toxicity – like by having the same color as other highly toxic prey – and the bird that wants to consume it possesses developed bitter taste receptors, the bird will be able to tell the level of toxicity, and the false advertising will not be very effective. Therefore, the actual chemical defense of toxins is better for survival and is more prevalent in evolution. More specifically, bitter-tasting toxins that predators will remember the taste of are more successful than those that cannot be detected by birds, as that detection is what allows prey to be avoided and survive (Skelhorn and Rowe, 2010).
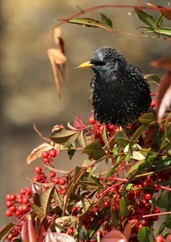
Accordingly, it is beneficial for birds to have well that well-developed bitter taste, so they can eat more while not being poisoned.
Bitter taste comes from the interaction of chemicals with a group of G protein-coupled receptors named Tas2rs that are encoded by taste receptor genes called Tas2rs (Confusing, right? The difference is that the genes are italicized). The total number of these Tas2rs genes varies greatly. In general, birds possess a smaller number of Tas2rs. So far, they have been examined in 16 birds and the number ranges from 1 in the dove to 18 in the white-throated sparrow (Wang and Zhao, 2015). Carnivorous birds have few Tas2rs, while birds with more plants and insects in their diets have more. This is because the number of functional Tas2rs genes is positively correlated to the amount of potential toxins in the organism's diet (Wang and Zhao, 2015).
Umami
What carnivorous birds lack in bitter detection, they make up for in umami receptors. Many birds, especially carnivorous species, have diets rich in proteins, glutamate and amino acids, erring on the side of savory. Thus, one might suspect taste preference towards umami flavor. The umami flavor is likely the least commonly known of the five human tastes. So for clarification, ‘umami' is the Japanese word for savory, and its flavor is often associated with meat or bone broth and is very prevalent in Asian cuisine. The Tas1r1 and Tas1r3 receptor genes are responsible for umami taste recognition in humans. Genome sequences of many birds indicate the presence of these genes in select species, such as chickens, turkeys, loons, zebra finches, egerts, and tubenose seabirds. Each of these likely has the ability to recognize the taste of umami, and may even enjoy it. Many bird species adjust their diets depending on nutrient deficiencies, while some feed based on fondness of flavors. For instance, chickens are intuitive feeders who feed based on need rather than desire. Unsurprisingly, when studied, they seemed to adjust their food choices to compensate for nutrient shortages. Given the little data in this area of study, what is known is generally applied to all birds. Thus, it is said that for umami, and most flavors, birds generally do not have dietary preferences stemming from taste – while this could be a characteristic unique to chickens. To this effect – and providing some counter examples, some researchers figured that since starlings (Fig. 18) consume large amounts of insects, they must have developed a stronger preference for amino- acids than red-winged blackbirds (Fig. 19), who seem to have more variety in their diet.
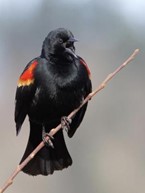
The research revealed that both blackbirds and starlings increased their concentration of specific amino acids per gram of mass of their body, using the supplementary sources in the provided meals. Still, starlings consumed the amino acids at a higher rate than blackbirds overall. This would indicate that starlings indeed have a stronger affinity – not just need – for umami-flavored insects. However, a possible flaw in the experiment was that the supplements were offered via solution-soaked rice, but the rice soaked in tannic acid was far less popular among both species. This likely interfered with the study, given birds' aversion to sour or bitter sensations (Rowland et al., 2015). Something rather unique about the umami receptors is that they are thought to be involved in adjustments of post-ingestive and metabolic events, and are located in the hypothalamus, liver and fat tissues, not just near the bill. This gives the bird a more informed cravings system, as it gets regular feedback about how amino acid levels are doing throughout its system (Martin, 2017).
Calcium
Parents often nag their young children to drink their milk so that they can “grow big and strong”. In the case of birds, this is excellent advice as calcium is one of the most essential mineral requirements in their diet. Particularly important for egg laying and bone strength, large amounts of calcium are needed to construct the shells. Given that chickens lay so frequently, they require calcium-rich diets and, over time, have likely developed a liking to the flavor. When a group of calcium deprived chickens were offered supplementary calcium carbonate, their consumption of this supplement was inversely related to the concentration of calcium in their typical diet (Roura et al., 2013). They have fine-tuned regulation systems and do not go overboard. In another study, broiler chickens were offered diets containing different amounts of calcium, and the results proved the chickens were capable of distinguishing between concentrations. Further, that they may target a concentration that best suits their needs and appetite. What was rather intriguing about the case, however, was that each individual broiler chicken was not equally attracted to calcium, implying allelic variation across the calcium sensors of the broiler chicken population (Roura et al., 2013). For all laying birds, calcium is likely the most limiting micronutrient when it comes to reproduction. The shell acts as protection to the embryo and encases all integral nutrients for embryonic development (Hughes and Wood-Gush, 1971). The survival of the embryo is heavily reliant on the crystalline form of calcium carbonate of the eggshell. Throughout its incubation period, the embryo will absorb calcium from the shell and, once born, the chicks require calcium-rich foods in feeding from their mothers (Reynolds and Perrins, 2010). Fig. 20 shows a strong crystalline calcium carbonate structure of an avian egg. This is a healthy structure which would be able to house and nourish an embryo as there is no breakage and the structure appears to be thick.
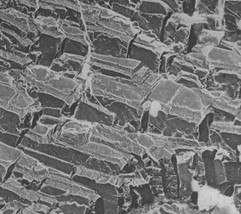
Calcium to birds is not about growing big and strong – they have modest ambitions and will hold off when those are met. It is simply about not being feeble and requiring the minimum intake to form solid-enough structures that the bird is healthy, and its eggs are not puddles.
Fat
Although the likelihood of birds' ability to taste fats has been widely discredited, many studies continue in hopes that chickens in particular will take a liking to it. Of course, this would increase their weight and overall desirability to the consumer market. Many poultry farms will regulate the fat content in the diet of the birds to fatten them up and instill a liking towards oil types (Roura et al., 2013).
A promising lead centers around the G-protein-coupled receptor 120 (GPR120), a known fatty acid receptor present in mammals' mouths, as the cGPR120 gene which codes for GPR120 was detected in the chicken palate. To test its fatty acid-sensing capabilities in chickens, a study cloned the gene to obtain GPR120, which was then subjected to oleic acid and linoleic acid. Both fatty acids were shown to be agonists of cGPR120, meaning that oleic acid and linoleic acid bound to the receptor, and this stimulus produced a chemical response, much like those that send sensory taste information. The authors thus predict that cGPR120 would be expressed in the chickens' tastes buds (Sawamura et al., 2015), and this could further be extended to support the existence of fat-tasting abilities in chickens.
Given these results, the study then fed chickens food rich in corn oil, which contains a lot of oleic acid and linoleic acid, as well as food rich in mineral oil that does not contain these fatty acids. The chickens demonstrated a preference for the corn oil (Sawamura et al., 2015). Further studies are needed to support these findings and to explore them in depth, but these results could be very useful to the poultry industry when seeking to increase feed intake.
Miscellaneous
There are also some taste adaptations that are not as analogous to human sensations, so those will be touched upon in this section.
For instance, sandpipers (Fig. 21) locate food underground through taste. These are a type of probing bird, like kiwis and red knots, so they have long beaks with which they poke holes in wet sand to unearth worms, mollusks or crustaceans (Cunningham et al., 2013).
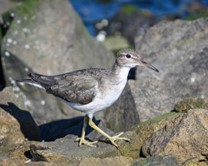
Studies were conducted to determine how exactly this technique works – as the birds have higher retrieval success than models based on randomness would predict. The results were that it depends on the species. Red knots cannot taste at all, and detect purely through pressure and touch (Piersma et al., 1998). Sandpipers, however, do not have that ability, but still have high rates of success. When presented with buckets of wet sand that had had worms and mollusks in it (and was now empty, but flavored) and clean, wet sand, sandpipers spent more time searching in the sand that had had worms (Martin, 2017), while red knots made no distinction and moved on to buckets of rocks and mollusks (Piersma et al., 1998). In this case, taste serves sandpipers similarly to smell; it is a location strategy that allows them to narrow in on a target based on the intensity of the nearby compounds it emits.
Another interesting case is the famous bald eagle. Eagles do not have a strong sense of smell, unlike vultures; they rely mostly on sight to locate delicious targets. These include live animals, but also sickly or wounded animals, and even dead prey they come across. They also steal from other predators. And sight alone cannot always distinguish between what is, and what very much is not edible. Luckily, taste can. After a few nibbles, the bird can determine whether the carcass is spoiled and will go eat something else if necessary (“Can birds taste things?' | Canadian Wildlife Federation, n.d.).
Ducks and mallards (Fig. 22) also distinguish between edible and inedible food, but they do so through their bill tip organ (Berkhoudt, “The Epidermal Structure of the Bill Tip Organ in Ducks”). This organ contains two types of mechanoreceptors: Herbst corpuscles and Grandry corpuscles. The first type allows the bird to detect vibrations, while the second type allows them to detect changes in pressure or velocity against their bill (Avilova, 2018). The precision of this sensory organ is so impressive that mallards can tell submerged, hard, edible peas from similarly shaped and sized plasticine peas (Berkhoudt, “The Epidermal Structure of the Bill Tip Organ in Ducks”). That result is mainly an indicator of how they proceed in the wild to differentiate their diet from other food, or plastics and such, based on touch. Given the pollution currently found in nature, that scenario may be more likely than one would think! Another study showed that ducks can differentiate between healthy peas and unpleasant-tasting ones, from that same initial tip of the bill, which houses – along with the other specialized cells – a collection of taste buds (Rowland et al., 2015).
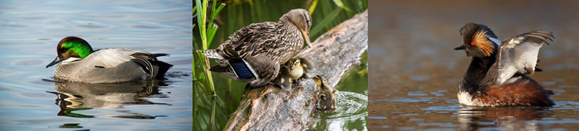
Fig. 22 (b) (middle) Adult female and downy young, mallard. Photographed by Owen Deutsch. [Adapted from “Mallard” | National Audubon Society, n.d.]
Fig. 22 (c) (right) Breeding adult, eared grebe. Photographed by Peter Knoot. [Adapted from “Eared Grebe” | National Audubon Society, n.d.]
Similarly, grebes can identify infected fish by holding it in the back region of their bills, where the taste buds are chilling (Rowland et al., 2015). What does an infection taste like? It remains to be elaborated. The research confirmed that the birds reject infected fish based on taste though (Rowland et al., 2015), so there is an answer out there.
Conclusion
To put it plainly, birds can taste. Some birds can taste quite well, others not so much. A prevalent theme of this report has been ambiguity – it is difficult to generalize as there are species that make the exception to every rule relating to gustation. In fact, a list of birds employing the same set of taste mechanisms would likely be very small. There is a lot of diversity in nature, and much research to be done on taste in birds; chickens are by far the most documented while few people would claim they are the most interesting. But already, the knowledge collected about bird gustation has several impactful applications in the food industry – both for catering to, and for working against the avian palate.
Firstly – and this is why chickens are so paparazzi-ed – humanity consumes a lot of chicken, and a lot of eggs. Unfortunately, the industry tends not to be very conscious of animal well-being, with the birds experiencing cramped cages and clipped beaks. Their diets are also optimized for output by choosing tastes for which the chickens increase their feed intake (Niknafs and Roura, 2018). Copious amounts of money have been invested in trying to determine the best nutrient and fat ratios in the farms of the industry titans. Luckily, small farms still want their free-range chickens to have well-balanced diets.
Secondly, farmers looking to keep birds away from their crops can also look to nature to find solutions and utilize observations on preferences for developing non-harmful avian repellents. One compound they can take advantage of is found in the skin of grapes. That is, methyl-anthranilate – the same one ants use to not get eaten (Conner et al., 2007). But researchers are actively developing new ones. Generally, the compounds to which birds are averse are more basic (pH-wise) and rigid (planar) molecules than those that affect humans more strongly (Clark et al., 2014). When selecting an appropriate repellent, there are two strategies to choose between. The first, primary repellents, directly impact the bird through a taste or an odor that the bird rejects, or from an irritant that makes the bird uncomfortable, and make it discontinue its feeding. The second is a little more subtle – secondary repellents cause undesirable post-ingestive effects that the bird then associates with a particular taste or odor (or appearance in some cases). These effects can include illness or pain as a result of consuming a certain item. The bird naturally wishes to avoid repeatedly going through these experiences and does so by staying away from the stimulus that preceded the undesirable effect. Secondary repellents are generally more effective, as primary repellents can cause an initial adverse reaction, but the bird may tolerate it as time goes by and it gets used to the effect (Werner and Clark, 2003). In either case, these are deterrents – not poisons – so it takes an understanding of taste to innovate new ones.
It remains to be seen what new applications further discoveries will inspire. They could stem from discovering receptors and new ways of detecting compounds, or from isolating odors aversive to pigeons and seagulls but unperceivable to humans (and diffusing these smells in bird-plagued cities). Who knows? All that can be said for now is that beaks are fascinating, and their biochemistry makes no exception.
Appendix
“Emu's eating in slow motion.”, 6 July 2019, CONTENTbible, YouTube, https://www.youtube.com/watch?v=OTMAKJ3xioM.
References
Accommodation, S. A. (n.d.). Cape Sugarbird. Retrieved from https://www.sa-venues.com/wildlife/birds_cape_sugarbird.php
Al, A. (2013). Gentoo Chick tongue. In. Flickr. Retrieved from https://www.flickr.com/photos/arctic_al/8453462457/in/photolist-6yxwjp-dT1cSH
Avilova, K. V. (2018). Spatial Organization of the Epithelial Structures in the Bill Tip Organ of Waterfowl (Anseriformes, Aves). Biology Bulletin Reviews, 8(3), 234-244. doi:10.1134/S2079086418030027
Baldwin, M. W., Toda, Y., Nakagita, T., O'Connell, M. J., Klasing, K. C., Misaka, T., . . . Liberles, S. D. (2014). Evolution of sweet taste perception in hummingbirds by transformation of the ancestral umami receptor. Science, 345(6199), 929-933. Retrieved from https://science.sciencemag.org/content/sci/345/6199/929.full.pdf
Barakate, M., & Elwin, D. B. (2015). Can you retrain your taste buds? Retrieved from https://ent-surgery.com.au/can-you-retrain-your-taste-buds/
Berkhoudt, H. (1975). The Epidermal Structure of the Bill Tip Organ in Ducks. Netherlands Journal of Zoology, 26(4), 561-566. doi:https://doi.org/10.1163/002829676X00226
Berkhoudt, H. (1992). Avian Taste Buds: Topography, Structure and Function. In R. L. Doty & D. Müller-Schwarze (Eds.), Chemical Signals in Vertebrates 6 (pp. 15-20). Boston, MA: Springer US.
Bertin, A., Calandreau, L., Arnould, C., & Lévy, F. (2012). The developmental stage of chicken embryos modulates the impact of in ovo olfactory stimulation on food preferences. Chemical Senses, 37(3), 253-261. doi:10.1093/chemse/bjr101
Birds, T. L. (2014). Let's talk about bird tongues. Retrieved from https://toughlittlebirds.com/2014/11/20/lets-talk-about-bird-tongues/
BirdTricks. (2013). What's Inside Your Bird's Mouth? Retrieved from https://birdtricksstore.com/blogs/birdtricks-blog/what-s-inside-your-bird-s-mouth
Blumin, L. (2012). Falcated Duck In. Flickr. Retrieved from https://www.flickr.com/photos/lenblumin/8268585850/in/photolist-dAQm4G-dAJTiF-dAEEv1-aYkfUB-dAQm6u-aYkfWT
Braun, E. J. (2015). Chapter 12 – Osmoregulatory Systems of Birds. In C. G. Scanes (Ed.), Sturkie's Avian Physiology (Sixth Edition) (pp. 285-300). San Diego: Academic Press.
Broch, L. (n.d.). Allen's Hummingbird. In. Audubon Photography Awards: National Auudbon Society. Retrieved from https://www.audubon.org/field-guide/bird/allens-hummingbird#photo2
Carlson, K., McDonald, R., Mendoza Cabrer, P., & Weldon, M. (2020). Physics of Avian Beak Design. Bioengineering. McGill University Montreal, Canada. https://bioengineering.hyperbook.mcgill.ca/physics-of-avian-beak-design-2/
Clark, L., Hagelin, J., & Werner, S. (2014). The Chemical Senses in Birds. In Sturkie's Avian Physiology: Sixth Edition.
Conner, W. E., Alley, K. M., Barry, J. R., & Harper, A. E. (2007). Has vertebrate chemesthesis been a selective agent in the evolution of arthropod chemical defenses? Biol Bull, 213(3), 267-273. doi:10.2307/25066644
Cunningham, S. J., Corfield, J. R., Iwaniuk, A. N., Castro, I., Alley, M. R., Birkhead, T. R., & Parsons, S. (2013). The Anatomy of the bill Tip of Kiwi and Associated Somatosensory Regions of the Brain: Comparisons with Shorebirds. PloS One, 8(11), e80036. doi:10.1371/journal.pone.0080036
Deutsch, O. (n.d.). Mallard. In. Audubon Photography Awards: National Audubon Society. Retrieved from https://www.audubon.org/field-guide/bird/mallard#photo2
Editorial, C. (2011, 21 Jan 2021). Bird Beak Anatomy Retrieved from https://be.chewy.com/bird-beak-anatomy/
Erdoğan, S., & Iwasaki, S. (2014). Function-related morphological characteristics and specialized structures of the avian tongue. Ann Anat, 196(2-3), 75-87. doi:10.1016/j.aanat.2013.09.005
Federation, C. W. (n.d.). Can birds taste things? Retrieved from https://cwf-fcf.org/en/about-cwf/faq/faqs/can-birds-taste-things.html
Heyn, A. N. J. (1963). The crystalline structure of calcium carbonate in the avian egg shell: An electron microscope study. Journal of Ultrastructure Research, 8(1), 176-188. doi:10.1016/S0022-5320(63)80029-5
Hughes, B. O., & Wood-Gush, D. G. M. (1971). A specific appetite for calcium in domestic chickens. Animal Behaviour, 19(3), 490-499. doi:10.1016/S0003-3472(71)80103-3
InformedHealth.org. (2016). How does our sense of taste work? In InformedHealth.org. Retrieved from https://www.ncbi.nlm.nih.gov/books/NBK279408/
Knoot, P. (n.d.). Eared Grebe. In. Audubon Photography Awards: National Audubon Society. Retrieved from https://www.audubon.org/field-guide/bird/eared-grebe
Knott, B. (2008). Heron Tongue. In. Flickr. Retrieved from https://www.flickr.com/photos/forgetmeknottphotography/2441898418/
Kuhn, T. (n.d.). Red-winged Blackbird. In. Audubon Photography Awards: National Audubon Society. Retrieved from https://www.audubon.org/field-guide/bird/red-winged-blackbird#photo6
Leshem, M. (2009). Biobehavior of the human love of salt. Neuroscience & Biobehavioral Reviews, 33(1), 1-17. doi:10.1016/j.neubiorev.2008.07.007
Levey, D. (1987). Sugar-Tasting Ability and Fruit Selection in Tropical Fruit-Eating Birds. The Auk, 104. doi:10.1093/auk/104.2.173
Litzinger, K. (n.d.). European Starling. In. Great Backyard Bird Count: National Audubon Society. Retrieved from https://www.audubon.org/field-guide/bird/european-starling#photo5
Macwhirter, P. (2009). 2 – Basic anatomy, physiology and nutrition. In T. N. Tully, G. M. Dorrestein, A. K. Jones, & J. E. Cooper (Eds.), Handbook of Avian Medicine (Second Edition) (pp. 25-55). Edinburgh: W.B. Saunders.
Matsubara, B. (2018). Spotted Sandpiper. In. Flickr. Retrieved from https://www.flickr.com/photos/beckymatsubara/40285030192/in/photolist-2jGCkBM-S6ik7G-24nRiY5-SCDwFj
NatureLoc. (2020). Can birds eye chili help in weight loss? What's the benefit of taking birds eye chilies in your diet? Retrieved from https://healthyliving.natureloc.com/can-birds-eye-chili-help-in-weight-loss-whats-the-benefit-of-taking-birds-eye-chilies-in-your-diet/
Niknafs, S., & Roura, E. (2018). Nutrient sensing, taste and feed intake in avian species. Nutrition Research Reviews, 31(2), 256-266. doi:10.1017/s0954422418000100
Optland, W. (2014). Rainbow lorikeet tongue. In (Vol. 3635 × 2770). Flickr. Retrieved from https://www.flickr.com/photos/62718813@N03/12057626964/sizes/l/
Ornithology, C. L. o. (2017, 6 June 2017). Why Can Some Birds Drink Salty Seawater? Living Bird(Summer 2017). Retrieved from https://www.allaboutbirds.org/news/why-can-some-birds-drink-salty-seawater/
Piersma, T., Aelst, R. v., Kurk, K., Berkhoudt, H., & Maas, L. R. M. (1998). A new pressure sensory mechanism for prey detection in birds: the use of principles of seabed dynamics? Proceedings of the Royal Society of London. Series B: Biological Sciences, 265(1404), 1377-1383. doi:doi:10.1098/rspb.1998.0445
Plants, S. D. Z. A. (n.d.). Macaw. Retrieved from https://animals.sandiegozoo.org/animals/macaw
psittacology. (2020, 30 Nov 2020). Lorikeet tongue: the strangest tool in the parrot world. Retrieved from https://www.psittacology.com/lorikeet-tongue-diet/
Raj, N., & Mahalekshmi, T. (2018). Multilabel Classification of Membrane Protein in Human by Decision Tree (DT) Approach. Biomedical and Pharmacology Journal, 11, 113-121.
Reilly, S. M., McBrayer, L. D., & White, T. D. (2001). Prey processing in amniotes: biomechanical and behavioral patterns of food reduction. Comparative Biochemistry and Physiology. Part A: Molecular and Integrative Physiology, 128(3), 397-415. doi:10.1016/s1095-6433(00)00326-3
Reynolds, S. J., & Perrins, C. M. (2010). Dietary Calcium Availability and Reproduction in Birds. In C. F. Thompson (Ed.), Current Ornithology Volume 17 (pp. 31-74). New York, NY: Springer New York.
Rivera-Brown, A., & Frontera, W. (2012). Principles of Exercise Physiology: Responses to Acute Exercise and Long-term Adaptations to Training. PM & R : the journal of injury, function, and rehabilitation, 4, 797-804. doi:10.1016/j.pmrj.2012.10.007
Roper, S. D. (2014). TRPs in Taste and Chemesthesis. In B. Nilius & V. Flockerzi (Eds.), Mammalian Transient Receptor Potential (TRP) Cation Channels: Volume II (pp. 827-871). Cham: Springer International Publishing.
Roura, E., Baldwin, M. W., & Klasing, K. C. (2013). The avian taste system: Potential implications in poultry nutrition. Animal Feed Science and Technology, 180(1), 1-9. doi:10.1016/j.anifeedsci.2012.11.001
Rowland, H. M., Rockwell Parker, M., Jiang, P., Reed, D. R., & Beauchamp, G. K. (2015). Comparative Taste Biology with Special Focus on Birds and Reptiles. In Handbook of Olfaction and Gustation (pp. 957-982).
Sawamura, R., Kawabata, Y., Kawabata, F., Nishimura, S., & Tabata, S. (2015). The role of G-protein-coupled receptor 120 in fatty acids sensing in chicken oral tissues. Biochem Biophys Res Commun, 458(2), 387-391. doi:10.1016/j.bbrc.2015.01.125
Sequeria, F. B. (n.d.). Red-capped Manakin. In. eBird. Retrieved from https://ebird.org/species/recman1
Skelhorn, J., & Rowe, C. (2010). Birds learn to use distastefulness as a signal of toxicity. Proceedings of the Royal Society B: Biological Sciences, 277(1688), 1729-1734. doi:doi:10.1098/rspb.2009.2092
Slack, J. (2016). Molecular Pharmacology of Chemesthesis. In (pp. 375-391).
Smith, D. V., & Margolskee, R. F. (2001). Making Sense of Taste. Scientific American, 284(3), 32-39. Retrieved from http://www.jstor.org/stable/26059127
Stevens, M., & Ruxton, G. D. (2012). Linking the evolution and form of warning coloration in nature. Proceedings of the Royal Society B: Biological Sciences, 279(1728), 417-426. doi:doi:10.1098/rspb.2011.1932
Tewksbury, J. J., & Nabhan, G. P. (2001). Directed deterrence by capsaicin in chillies. Nature, 412(6845), 403-404. doi:10.1038/35086653
Toda, Y., Okada, S., & Misaka, T. (2011). Establishment of a new cell-based assay to measure the activity of sweeteners in fluorescent food extracts. J Agric Food Chem, 59(22), 12131-12138. doi:10.1021/jf2029835
Treesukosol, Y., Smith, K. R., & Spector, A. C. (2011). The functional role of the T1R family of receptors in sweet taste and feeding. Physiol Behav, 105(1), 14-26. doi:10.1016/j.physbeh.2011.02.030
Wang, K., & Zhao, H. (2015). Birds Generally Carry a Small Repertoire of Bitter Taste Receptor Genes. Genome Biology and Evolution, 7(9), 2705-2715. doi:10.1093/gbe/evv180
Werner, S., & Clark, L. (2003). Understanding blackbird sensory systems and how repellent applications work.
Wuori, J. (n.d.). Summer Tanager. In. Audubon Photography Awards: National Audubon Society. Retrieved from https://www.audubon.org/field-guide/bird/summer-tanager#photo5
Zaremba, K. (n.d.). Black-footed Albatross. In. Audubon Photography Awards: National Audubon Society. Retrieved from https://www.audubon.org/field-guide/bird/black-footed-albatross#photo3