The Nose: An Examination and Comparison of the Turbinate Structures of Aquatic Animals, Terrestrial Caniformia, and Humans
Ingi El Shahid, Curtis Ehlert, Liv Toft, Mary Wan
Abstract
In recent years, researchers have studied biological systems with an engineering perspective, and have found that structures in nature are designed to respond to a specific function. The nose is one example of how evolution has formed a diversity of shapes for the same structure to accommodate the species' needs as a result of climate, latitude, environment, survival from predators, as well as numerous other selective pressures. More specifically, we will examine how these factors have influenced the structure, and subsequent function, of the nasal turbinates of three groups of mammals: aquatic animals, terrestrial Caniformia, and humans.
The Nasal Turbinate: A General Overview
The nasal turbinate is a structure made of thin spongy layers of bone, situated on the walls of the nasal cavity of vertebrate animals. Its main purpose is to increase the surface area of the nasal cavity to allow the air passing through the nose to be heated up, humidified, and filtered before entering the lungs. By expanding the contact surface of the nasal cavity, the inspired air spends more time in the nasal airway. This allows for the air molecules to take more time to be heated up to the body's temperature (“Anatomy and Physiology of the Nasal Cavity (Inner Nose) and Mucosa” | My VMC, 2015).
The functions of the turbinates are enabled by the presence of the mucosal surface in its structure. Its main components are epithelial cells, endothelial cells, mucus glands, cilia, and blood vessels. The epithelial cells act as a physical barrier that prevent the invasion of pathogens in the nasal cavity (“Anatomy and Physiology of the Nasal Cavity (Inner Nose) and Mucosa” | My VMC, 2015). They also regulate immune responses when the physical barrier fails. The epithelial cells have antigen-binding proteins that lead pathogens to T-lymphocyte cells to get destroyed. The endothelial cells act following an allergic reaction in the nose by attracting white blood cells to the inflammation site. The mucus glands are responsible for producing a sticky substance called mucus that attracts and traps pathogens as well as moistens the nasal cavity. Cilia are little hairs in the nose that move the mucus from the nasal cavity to the throat to be digested by stomach acids. Finally, blood vessels regulate the temperature of the nasal cavity to warm the air entering the nose (“Anatomy and Physiology of the Nasal Cavity (Inner Nose) and Mucosa” | My VMC, 2015).
Furthermore, most turbinates can be separated into three structures: the inferior, middle and superior conchae. The inferior concha is the largest out of the three bones and contains most of the structures described above. Its main role is to regulate the immune response and filtrate incoming air. The middle concha's role is to protect the sinuses. The superior concha protects the olfactory bulbs, which is the area in the brain responsible for processing the olfactory stimuli into information that can be analyzed by the brain (“Nasal Conchae (Nasal Turbinates)” | The Respiratory System, 2015).
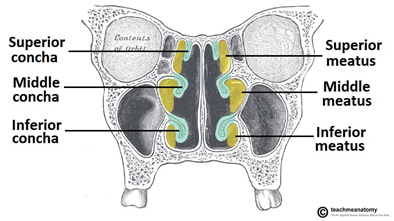
While most turbinates found in vertebrae have the same basic structures described above, each species' nasal conchae have unique characteristics that result from adaptation to their specific environment. The following paragraphs compare the turbinates of a range of species to better understand the relation between their unique structures and their functions.
Turbinal Trends and Comparisons in Aquatic Species
The features of nasal turbinates in aquatic animals have been shaped by the activities the animal performs on land versus in water. A variety of these aquatic animals will be examined, including the river otter, northern elephant seal, and Caribbean monk seal. Though these animals spend some time on land, they spend much of their time in aquatic environments. Due to this, their turbinates reflect the unique function they must perform to inhabit these environments. The river otter will be discussed in this part to provide relevant comparison as well, but this animal is classified to be more terrestrial than the preceding species. There are three main sections of mammal turbinates: the maxilloturbinals (connected to the maxillary bone), the nasoturbinals (connected to the nasal bones), and the ethmoturbinals (connected to the ethmoid) (Yee et al., 2016). To describe and compare the turbinates, the surface area (SA) of the maxilloturbinates, ethmoturbinates, and nasal turbinates will be examined first. Then, the respiratory nasal turbinates (maxilloturbinates) of various seals will be explored.
Often, aquatic animals have adapted to conserve body heat and water: body heat is lost faster in water than in air and respiratory evaporation is one of the most common ways water exits the body (Ortiz et al., 1978). The latter problem is especially magnified in aquatic species that lack access to fresh water. These species have evolved to solve these problems by enlarging their respiratory turbinates (maxilloturbinates) by increasing the number of folds in the turbinates (Niven and Laughlin, 2008). This allows more opportunity for the exhaled air to be cooled below body temperature and thereby condense onto turbinate mucosa, saving water. Thus, aquatic species generally have relatively greater maxilloturbinate SA when compared to terrestrial species (Niven and Laughlin, 2008). Additionally, to compensate for the extra energy expended to retain heat and water, aquatic species tend to have reduced olfactory turbinate SA (combined ethmoturbinates and nasal turbinates SA) when compared to terrestrial species since olfaction does not significantly help with detecting food or predators underwater. In addition to this, it takes a lot of energy to maintain and use the olfaction system, so it makes more sense for the animal to allocate that energy to complete a more useful job (Niven and Laughlin, 2008).

Fig. 2 illustrates that the sea otter, an aquatic species, has a higher proportion of all turbinates to be maxilloturbinates — respiratory turbinates — compared to the wolverine, a terrestrial species.
Northern Elephant Seal
An interesting example that illustrates the above characteristics is the northern elephant seal which spends about nine months in the salty ocean water per year. These seals have expanded maxilloturbinates to conserve water when they fast during breeding, with respiratory turbinates occupying 90 % of total turbinate SA to retain up to 92 % of the water in each breath (Lester and Costa, 2006). The additional surface area has allowed these seals to effectively take advantage of nasal countercurrent heat exchange, which further reduces water loss (Huntley et al., 1984). In fact, the ratio of olfactory to respiratory SA for these species was found to be 1:9 (Van Valkenburgh et al., 2011). For these seals in particular, the conservation of water is likely equally or more important than the conservation of heat.
Caribbean Monk Seal
In contrast to the northern elephant seal, the now extinct Caribbean monk seal (LeBoeuf et al., 1986) had a surprisingly low respiratory SA despite being an aquatic species. Because the Caribbean monk seal used to inhabit warm Caribbean waters, there was little drive for the expansion of respiratory SA to conserve heat and, to some extent, water as these species had an olfactory to respiratory SA ratio of 1:1 (Van Valkenburgh et al., 2011). These species support the idea that the climate affects the development of turbinates to adapt accordingly to the animal's habitat.
The scans in Fig. 2 highlight the lack of enlarged of maxilloturbinates in the monk seal compared to another aquatic species, the Antarctic leopard seal which will be discussed further on.
River Otters
Though more terrestrial than the two species discussed above, the river otter possesses aquatic species characteristics, having a large respiratory SA relative to the whole chamber volume compared to terrestrial species and only having 38 % olfactory SA (Van Valkenburgh et al., 2011). This is surprising as these animals tend to spend a sizable amount of time on land and scent mark regularly (Kruuk, 2006). However, this finding can be understood because, unlike northern elephant seals, river otters live in an environment where freshwater is abundant; thus, evolving expanded respiratory SA to mainly conserve heat rather than water. River otters illustrate how turbinates of semi-terrestrial species have evolved for these species to take advantage of both life on land and in water.
Phocid Seals
Phocids are a group of marine animals mostly inhabiting colder waters including the polar regions. In particular, polar seals, grey seals, and monk seals of the Phocidae family have adapted their respiratory turbinate structures based on the environmental temperature. These seals — and seals in general — are known to have a poor sense of smell due to small olfactory turbinates, but have high surface area maxilloturbinates that are branched (Folkow et al., 1988; Mills and Christmas, 1990; Skog and Folkow, 1994).
Placed before the discussion of the phocid seals are the following three figures (i.e., Fig. 3, 4, and 5) whose results will be mentioned and compared.
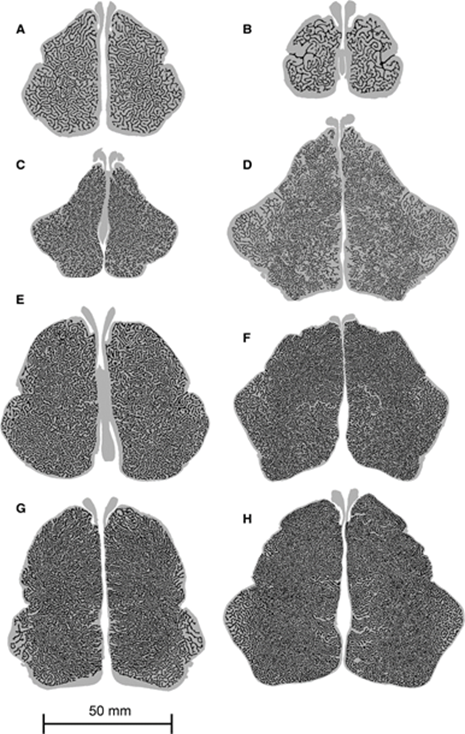

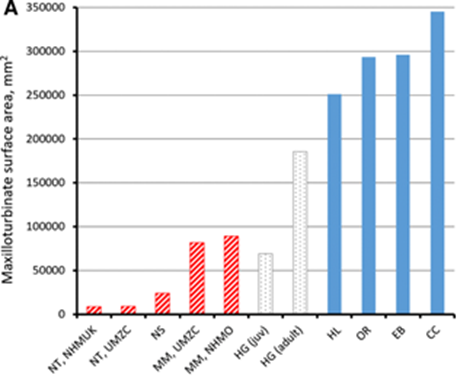
The polar seals that will be analyzed are two Antarctic seals, the Ross and Leopard seal, and two Arctic seals, the Hooded and Bearded seal. These seals live in very cold water all year long, making heat conservation of the utmost importance. Polar seals have grown densely packed maxilloturbinates that appear similar to dendrites. For all four seals, this feature is evident in the middle part of both nasal cavities; however, towards the edges, the branching becomes more dispersed. Specifically, the design of those of the leopard seal looks similar to that of a fingerprint as the turbinates curve around each other towards the outer edges (Mason et al., 2020).
Further, polar seals have the highest maxilloturbinate section perimeter compared to the grey and monk seals when analyzing the perimeters of the maxilloturbinates taken at different points along the nasal cavities (Mason et al., 2020). Polar seals also exhibit the largest length of the snout covered by maxilloturbinates. By taking the area under each curve respectively, the total SA of the maxilloturbinates were determined and that of the lowest polar seal is approximately three times higher than that of the highest monk seal (Mason et al., 2020). This substantial discrepancy can be explained by polar seals displaying an increase in cross-sectional area of the nasal cavity, a more complex and densely packed turbinates and a longer region of nasal cavity covered by maxilloturbinates (Mason et al., 2020). Having an expanded respiratory SA ensures that the total SA exposed to exhaled air is greater, improving heat exchange; possessing a complex, tightly packed web of maxilloturbinates allows for a shorter distance between the center of an airstream and a surface of a maxilloturbinate, enhancing heat exchange (Schmidt-Nielsen et al., 1970); having a bigger cross-sectional area slows down airflow which in turn relieves some of the resistance induced by the densely packed maxilloturbinates, further aiding heat exchange (Mason et al., 2020). It makes sense that polar seals have these features since these seals are constantly surrounded by cold air, making heat conservation extremely important.
As for the conservation of water, since all these seals are marine animals, they do not have access to fresh water aside from water obtained from their food or through their metabolism (Nordøy et al., 1992). Several studies have shown that the need to conserve water using turbinates may not be that significant specifically for species in colder environments (Folkow et al., 1988; How and Nordøy, 2007; Nordøy et al., 1992). For these species, conservation of heat is a stronger selective pressure.
The maxilloturbinates of the monk seals — specifically the Hawaiian and Mediterranean monk seals — are also long and curved such that they look like fingerprints, but they are relatively spread out compared to those of polar seals. As well, those of the Hawaiian monk seal branch less than the Mediterranean monk seal (Mason et al., 2020). Compared to the polar seals, the monk seals live in warm environments, so the need to conserve heat does not substantially drive the expansion of maxilloturbinates. This is reflected by the monk seals having the lowest maxilloturbinate section perimeter of all three groups and, thus, the smallest total SA (Mason et al., 2020). The need to conserve water may be more important as suggested in the discussion of the Caribbean monk seal.
In between the monk and polar seals is the grey seal that lives in intermediate temperatures. The design of the maxilloturbinates of these seals are not curvy nor branch out as long as those of the other two groups. Instead, they are more straight and shorter, especially in a juvenile grey seal (Folkow and Blix, 1987). They have a maxilloturbinate section perimeter between the polar and monk seals, with the adult grey seal slightly below the lowest polar seal, indicating that the total SA is closer to that of a polar seal. This makes sense since the grey seal is also a cold-water species that needs to conserve heat. However, interestingly, grey seals can recover as much as 80 % of the respiratory water, suggesting that their respiratory turbinates also can help extensively with retaining water (Folkow and Blix, 1987).
Altogether, examples of the northern elephant seal, river otter, and phocid seals along with the figures demonstrate that aquatic species generally have shrunken olfactory turbinates and enlarged respiratory turbinates to conserve heat and water. However, the extent to which each of these selective pressures affect the species depends on the species habitat and intrinsic behaviors. Regardless, these species among many other aquatic species have greatly benefited from the modifications to their nasal turbinates, in particular the maxilloturbinates.
Turbinal Trends and Comparisons in Terrestrial Caniformia
Animals in the Caniformia family are a subgroup of carnivorans–terrestrial. Caniformia are dog-like animals that come in a variety of sizes and inhabit different climates. By intuition, Caniformia should use their nasal faculties often. They need their olfactory — smelling — faculties to hunt prey, and respiratory faculties to breathe in cold climates non-laboriously: arctic animals especially. There is only one such structure located in the nose that serves both olfactory and respiratory functions: the turbinates (or nasal conchae). The respiratory turbinals are defined by the maxilloturbinals, while the olfactory turbinals are defined by the naso and ethmoturbinals (Green et al., 2012).
Not all turbinate structures look the same, however. In fact, there are no two turbinate structures between two different species that look exactly alike. Knowing this, we can address the differences in turbinates between different Caniformia species and address how factors such as climate, environment, and latitude contribute to their structure (Green et al., 2012).
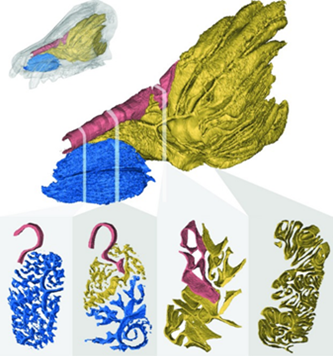
To answer this, three different families of Caniformia will be examined in detail: Ursidae (bears), with a concentration in polar bears because of their higher average latitude habitation; Canidae (canines or related to canines); and Mustelidae (a family including weasels, badgers, otters, ferrets, wolverines, etc.). They have been chosen as a notable sample due to the diversity of climates they inhabit, their size diversity, and because they are all terrestrial. Their likeness in body proportion and living predominantly on land should reduce outlying factors and focus differences in turbinate size and function on the animals' size, climate, and diet.
Bears
Inspiring cold air has unpleasant effects on many animals because the air they breathe is significantly less moist than the membrane that covers the nose and throat, and because it is so much colder than the temperature in the lungs (Bechshoft, 2019). The polar bear has no such problems; simply put, their long muzzle has turbinate structures extending from front to back that gives inspired air more time to be filtered. Indeed, the fact that the respiratory turbinals — maxilloturbinals — of polar bears are located at the front of the muzzle makes sense since the air requires time to be moisturized and heated — this is not unique to polar bears and is observable in all mammals (Bechshoft, 2019). However, arctic species tend to have greater relative respiratory turbinal surface area (RTSA) than temperate species due to their increased need for heat and water retention and tend to have enlarged olfactory turbinal surface areas (OTSA) — relative to temperate species — as well; the former can be credited to the cold and dry environments they find themselves in, and the latter can be credited to the wide home ranges of polar bears (Green et al., 2012). Essentially, both the olfactory and respiratory faculties must be heightened in polar bears due to their environment. Even though polar bears will spend a lot of their time in water, particularly in the Arctic Ocean hunting for seals, fish, etc. — and although they are considered the most aquatic of ursids, — they remain a terrestrial species that forage mostly on land, so their turbinate structures are not dependent on their aquatic activity (Valkenburgh et al., 2011).
When it comes to the grizzly, brown, and black bear species, there is not much variance between groups. These species tend to have similar, if not smaller respiratory and olfactory turbinal surface area proportions to polar bears, who are the outliers in Ursidae concerning environment since polar bears diverged from brown bears approximately 500 0000 to 800 000 years ago (Valkenburgh et al., 2011). It is observed that the enlargement of OTSAs and RTSAs is not a result of area and climate, at least measured by latitude, as much as ecological parameters. And despite spending a lot of time in the ocean, unique polar bear features are not credited to adapting to the water, since they still mostly forage for seals on land, and are still terrestrial, rather than aquatic or semi-aquatic creatures. Rather, their skull morphology – teeth and skull shape – has evolved dramatically due to their specialized seal blubber diet (Valkenburgh et al., 2011).
Canids
One might hypothesize that the OTSA of canids is relatively larger to most other animals, including Ursidae, because of dogs' exceptional smelling ability. Indeed, canids possess greater positive allometry than other mammals, including ursids (Green et al., 2012). This means that, as canids grow, their OTSA increases in size at a rate greater than the rest of their body. This discovery was accomplished through using the skulls of wild-caught animals with well-preserved turbinals and running them through a high-resolution computed tomography machine (HRCT), digitally cutting their skulls into 500 – 2000 slices and comparing the results. Interestingly, they found that the turbinate structures are composed of such paper-thin bones that they were difficult to separate from the background noise – so they used applied contrast limited adaptive histogram equalization to improve the contrast, which gave them a clearer image (Green et al., 2012).
But rather than scaling their OTSAs with a positive allometry, among Arctoidea, an infraorder of mostly carnivorous mammals that includes Ursidae, olfactory surface area is scaled isometrically. This means that morphological proportional relationships (e.g., turbinal to skull ratio, turbinal surface area to nasal volume ratio) stay constant as the size of species grows or shrinks in evolutionary time (Green et al., 2012).
Mustelids and Diet
Mustelidae make up the largest family in the Caniformia suborder and Carnivora order. Like ursids, they are a member of arctoid carnivorans, and share many of the same turbinate features with them. For example, their olfactory surface area scales isometrically as the species grows.
Although being called ‘carnivorans', which one might assume as meaning, directly, ‘carnivore', there are many animals — even some in the Caniformia family — that are omnivorous. Plenty of canids are included in this category, such as the raccoon dog and bat-eared fox (Green et al., 2012). Diet would seem like an obvious criterion to influence the relative sizes of OTSAs and RTSAs, since it influences animals to discriminate among different numbers of prey, as well as the ‘home range' of the animal (how far it must go to find food).
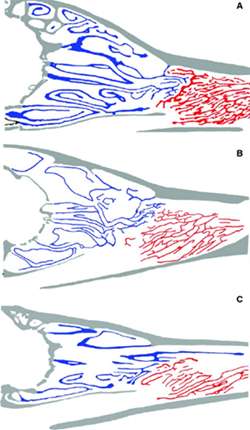
While the arctic fox is carnivorous, the raccoon dog and the bat-eared fox are omnivorous and insectivorous, respectively. The difference in the turbinals is striking — it is obvious that the arctic fox has greater surface area for both olfactory and respiratory turbinals than both of its canid cousins. — Indeed, the role of carnivorous diet cannot be downplayed: species in the vertebrate, vertebrate/non vertebrate dietary groups tend to have larger olfactory turbinals than species that non-vertebrate dietary group species (Green et al., 2012). When plotting a regression residual for olfactory turbinal surface area against chamber volume, Green et al. (2012) found that not only do vertebrate dietary groups have more positive residuals than the other groups (meaning their observed y-value is greater than their predicted y-value, in this case, olfactory surface area), but that the effect of diet is best expressed in the largest species of both canid and arctoid families.
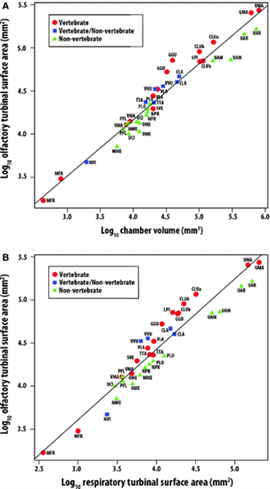
This can be shown in these regression residual plotlines. This is a contributing factor to why polar bears are shown to have a larger OTSA: they are the most carnivorous of the three sampled bears and have the most positive residuals. This is like the gray wolves for Canidae and the wolverine for Mustelidae (Green et al., 2012).
In summary, although climate as a measure of latitude does not predict a change in turbinal surface area in carnivora (neither olfactory nor respiratory), ecology and environment do. Changes of this sort can vary depending on the family, though. For example, because Canidae exhibit positive allometry, the largest dogs will have much more OTSA in relation to smaller dogs than large ursids and mustelids will (like the polar bear and the black bear, and the wolverine and the weasel) (Green et al., 2012). Furthermore, diet plays a crucial role amongst the Caniformia in that the more carnivorous an animal is, the more likely it will have relatively enlarged olfactory turbinal surface areas. Typically, the largest animals of a family are the most carnivorous, observed in the polar bear (Ursidae) gray wolf (Canidae) and wolverine (Mustelidae) (Green et al., 2012).
Human Turbinate
Anatomy
The human nasal anatomy is most characterized by what it is lacking rather than what it includes. Humans possess one pair of maxillo turbinates, also known as the inferior turbinates, and two to three pairs of ethmoturbinates: the middle, superior, and, if present, supreme turbinates (Marks et al., 2019). Humans, as well as primates, possess the simplest maxillo-turbinate, consisting of a single scroll structure (Mills and Christmas, 1989). In addition, unlike most other animals (although similar to the aquatic mammals discussed earlier), the olfactory area of the nose in humans is only found at the superior turbinate which is covered with olfactory mucosa (Mills and Christmas, 1989; Marks et al., 2019). The other turbinates — middle and inferior — are structurally similar to the ethmo-turbinates of animals; however, they are only used in air conditioning and are covered only with respiratory mucosa (Mills and Christmas, 1989; Marks et al., 2019). Moreover, humans lack a transverse septum which directs air to the olfactory region and the supreme turbinate is degenerated and entirely lacking in 80 % of humans (Mills and Christmas, 1989).
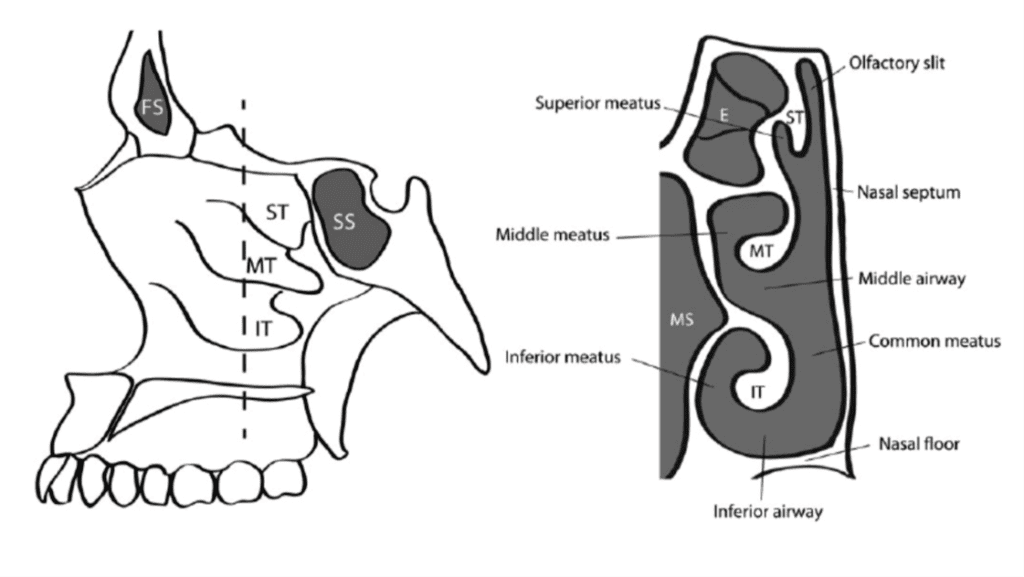
Function
The lack of structural design can be explained by the fact that humans do not rely on their sense of smell unlike many other mammals. Despite this, humans breathe primarily through their nose even though it requires more energy than mouth respiration (Naftali et al., 2005). One explanation comes from the fact that the nasal cavity guards against large particles, with mucous and small hairs acting as a line of defense against possible pathogens. However, more significantly, studies find that the nose can “condition inspired air to 90 % of alveolar conditions before reaching the nasopharynx” (Naftali et al., 2005). This air conditioning is important as it protects the lungs from thermal damage, infection, and dryness (Marks et al., 2019). The respiratory mucosa which line the maxillo turbinates produce mucus which helps to moisten the inspired air (Marks et al., 2019). In addition, the many capillaries which run along the turbinates evaporate water into the nasal airway, further increasing moisture, as well as transfer heat to the inspired air (Marks et al., 2019).
The mechanical structure of the maxillo turbinates also contributes to their air conditioning function. To understand how the mechanical structure of the human nose achieves this, one study created a nose-like model. During “quiet” breathing at 20 – 25 ºC, it was found that the inspired air was heated to around 34 ºC and the humidity was increased to 80 % after passing through the nose (Naftali et al., 2005). In addition, in cold temperatures of around -18 ºC, the inspired air was heated to 30 ºC (Naftali et al., 2005). It was also found that most of this heating and moisture addition had occurred by the time the air had left the turbinates region (Naftali et al., 2005). The role of the turbinates in this air conditioning process was studied by removing the inferior and middle turbinates. The efficacy of nasal conditioning decreased by 16 % and 12 % respectively (Naftali et al., 2005). It is speculated that the larger contribution to air conditioning from the inferior turbinate is due to the fact that it is longer (Naftali et al., 2005). Moreover, when the middle turbinate is removed, air is instead directed to the middle meatus. However, in the absence of the inferior turbinate, air is directed into a wide passageway where air-conditioning is ineffective (Naftali et al., 2005). It was found that the turbinates, along with the septal and lateral walls, account for 60 to 70 % of air conditioning (Naftali et al., 2005).
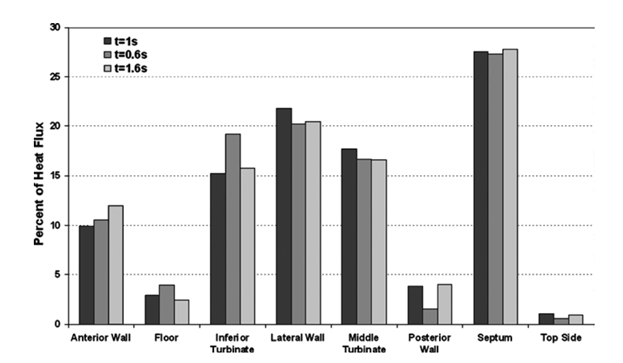
Human Turbinates as a Result of Climate Adaptation
Further insight into the function of human turbinates as a means for air conditioning can be seen in the structural differences of the turbinates of groups of individuals indigenous to equatorial Africa versus the arctic circle (Marks et al., 2019). When comparing the turbinates of these two populations, it was found that the inferior turbinates of people from the arctic circle were significantly superoinferiorly and mediolaterally larger (Marks et al., 2019). In the colder, drier climate of the arctic circle there is greater need for inspired air to be warmed and humidified. A larger maxillo turbinate is therefore desirable as there is an increase in contact between the respired air and the nasal mucosa which performs these functions (Marks et al., 2019). Conversely, in the hot, humid climate of equatorial Africa individuals do not need to warm nor humidify inspired air. Rather, minimized air contact with the maxillo turbinates is preferred as this minimizes airflow resistance and increases airflow efficiency (Marks et al., 2019). Turbinates function to condition inspired air, however, exactly how this air must be conditioned differs with environment and climate. Therefore, climate acts as a selective evolutionary pressure which leads to the morphological differences in maxillo turbinates observed in populations of unique environments. In addition, the structural differences in the maxillo turbinates of individuals of different climates further supports the notion that these turbinates function as thermo and humidity regulators in humans. If turbinates and air conditioning were not essential, these morphological differences would not be observed.
Conclusion
Nasal turbinates are an incredibly interesting structure to analyze to better understand structure-function relation. While most vertebrates have the same basic nasal conchae composition and structures, the size and proportion of each is evidently different. The features of nasal turbinates vary extensively depending on the environment in which the species lives. If that environment is predominantly water-based, the animal tends to have expanded respiratory turbinals, especially if temperatures are cold, to conserve water and heat. Although turbinate surface area scales differently with different species and families, mammals who are more carnivorous typically have larger olfactory turbinal surface areas. Finally, humans possess some of the simplest turbinates of any other mammal. Because humans do not rely heavily on olfaction, their turbinates reflect a function that focuses primarily on air conditioning. While there are observed differences between the turbinates of individuals living in colder, dryer climates versus warm and humid ones, the extent of turbinate evolution towards climate adaptation is not nearly as pervasive as that seen in polar bears and other arctic species. These groups of species, among many others, clearly show that changes in turbinate characteristics vary in degree, but nevertheless are crucial for the animals' survival in their unique habitats.
References
Bechshoft, T. (2019, 12 Mar 2019). Polar Bear Questions: Breathing in Cold Air. Retrieved from https://polarbearsinternational.org/news/article-polar-bears/polar-bear-questions-breathing-in-cold-air/
Folkow, L. P., & Blix, A. S. (1987). Nasal heat and water exchange in gray seals. American Journal of Physiology, 253(6 Pt 2), R883-889. doi:10.1152/ajpregu.1987.253.6.R883
FOLKOW, L. P., BLIX, A. S., & EIDE, T. J. (1988). Anatomical and functional aspects of the nasal mucosal and ophthalmic retia of phocid seals. Journal of Zoology, 216(3), 417-436. doi:https://doi.org/10.1111/j.1469-7998.1988.tb02439.x
Green, P. A., Van Valkenburgh, B., Pang, B., Bird, D., Rowe, T., & Curtis, A. (2012). Respiratory and olfactory turbinal size in canid and arctoid carnivorans. Journal of Anatomy, 221(6), 609-621. doi:https://doi.org/10.1111/j.1469-7580.2012.01570.x
How, O. J., & Nordøy, E. S. (2007). Seawater drinking restores water balance in dehydrated harp seals. Journal of Comparative Physiology. B: Biochemical, Systemic, and Environmental Physiology, 177(5), 535-542. doi:10.1007/s00360-007-0152-9
Huntley, A. C., Costa, D. P., & Rubin, R. D. (1984). The contribution of nasal countercurrent heat exchange to water balance in the northern elephant seal, Mirounga angustirostris. Journal of Experimental Biology, 113, 447-454.
Jones, O. (2019, 29 Sept 2019). The Nasal Cavity. Retrieved from https://teachmeanatomy.info/head/organs/the-nose/nasal-cavity/
Kruuk, H. (2006). Otters: ecology, behaviour and conservation: OUP Oxford. Retrieved from https://books.google.ca/books?id=i3uNfecE-LcC
LeBoeuf, B. J., Kenyon, K. W., & Villa-Ramirez, B. (1986). THE CARIBBEAN MONK SEAL IS EXTINCT. Marine Mammal Science, 2(1), 70-72. doi:https://doi.org/10.1111/j.1748-7692.1986.tb00028.x
Lester, C. W., & Costa, D. P. (2006). Water conservation in fasting northern elephant seals (Mirounga angustirostris). Journal of Experimental Biology, 209(Pt 21), 4283-4294. doi:10.1242/jeb.02503
Marks, T. N., Maddux, S. D., Butaric, L. N., & Franciscus, R. G. (2019). Climatic adaptation in human inferior nasal turbinate morphology: Evidence from Arctic and equatorial populations. American Journal of Physical Anthropology, 169(3), 498-512. doi:https://doi.org/10.1002/ajpa.23840
Mason, M. J., Wenger, L. M. D., Hammer, Ø., & Blix, A. S. (2020). Structure and function of respiratory turbinates in phocid seals. Polar Biology, 43(2), 157-173. doi:10.1007/s00300-019-02618-w
Mills, R. P., & Christmas, H. E. (1990). Applied comparative anatomy of the nasal turbinates. Clinical Otolaryngology and Allied Sciences, 15(6), 553-558. doi:10.1111/j.1365-2273.1990.tb00798.x
Naftali, S., Rosenfeld, M., Wolf, M., & Elad, D. (2005). The air-conditioning capacity of the human nose. Annals of Biomedical Engineering, 33(4), 545-553. doi:10.1007/s10439-005-2513-4
Niven, J. E., & Laughlin, S. B. (2008). Energy limitation as a selective pressure on the evolution of sensory systems. Journal of Experimental Biology, 211(Pt 11), 1792-1804. doi:10.1242/jeb.017574
Nordøy, E. S., Stijfhoorn, D. E., Råheim, A., & Blix, A. S. (1992). Water flux and early signs of entrance into phase III of fasting in grey seal pups. Acta Physiologica Scandinavica, 144(4), 477-482. doi:10.1111/j.1748-1716.1992.tb09324.x
Ortiz, C. L., Costa, D., & Le Boeuf, B. J. (1978). Water and Energy Flux in Elephant Seal Pups Fasting under Natural Conditions. Physiological Zoology, 51(2), 166-178. doi:10.1086/physzool.51.2.30157864
Schmidt-Nielsen, K., Hainsworth, F. R., & Murrish, D. E. (1970). Counter-current heat exchange in the respiratory passages: effect on water and heat balance. Respiration Physiology, 9(2), 263-276. doi:10.1016/0034-5687(70)90075-7
Skog, E. B., & Folkow, L. P. (1994). Nasal heat and water exchange is not an effector mechanism for water balance regulation in grey seals. Acta Physiologica Scandinavica, 151(2), 233-240. doi:10.1111/j.1748-1716.1994.tb09742.x
therespiratorysystem.com. (2015). Nasal Conchae (Nasal Turbinates). In. therespiratorysystem.com. Retrieved from https://www.therespiratorysystem.com/nasal-conchae-nasal-turbinates/
Van Valkenburgh, B., Curtis, A., Samuels, J. X., Bird, D., Fulkerson, B., Meachen-Samuels, J., & Slater, G. J. (2011). Aquatic adaptations in the nose of carnivorans: evidence from the turbinates. Journal of Anatomy, 218(3), 298-310. doi:https://doi.org/10.1111/j.1469-7580.2010.01329.x
VMC, M. (2015). Anatomy and Physiology of the Nasal Cavity (Inner Nose) and Mucosa. Retrieved from https://www.myvmc.com/medical-centres/lungs-breathing/anatomy-and-physiology-of-the-nasal-cavity-inner-nose-and-mucosa/
Yee, K. K., Craven, B. A., Wysocki, C. J., & Van Valkenburgh, B. (2016). Comparative Morphology and Histology of the Nasal Fossa in Four Mammals: Gray Squirrel, Bobcat, Coyote, and White-Tailed Deer. Anatomical record (Hoboken, N.J. : 2007), 299(7), 840-852. doi:10.1002/ar.23352