Review of the Mechanical, Structural, and Physical Properties of Pincers: An Evolutionary Wonder
Emilia Bridgeman, Claire Levasseur, Maxim Petkun, Alain Peng Zhang
Abstract
Pincers (alternatively known as chelae) are an integral and signature part of many arthropods. From arachnids to crustaceans, pincers exhibit critical functional roles in survival. The pincers of scorpions are notably multifunctional with their importance in seizing and capturing prey while inflicting pain and deterring predators. In crustaceans, chelae exhibit great functional and morphological diversity ranging from their use in coconut crabs to break coconuts to the reinforced pincers of hermit crabs, serving as a protective gate to the shell it inhabits. These notable behavioral features come as the result of the structural and mechanical properties of arthropod pincers which produce a highly reinforced structure. Primarily, the pincer structure is a hierarchical layered construction based on the twisted design of Bouligand architecture. This structural design produces a tool capable of withstanding the immense forces generated during the adduction of the chela as well as resisting damage from the environment. The following paper explores the details of the pincer's mechanical, structural, and physical properties and how these features produce a diverse system of practical tools for hunting and defense.
Introduction
In nature, a finite number of resources provokes competition between species. Darwin's mechanism of natural selection favors the randomly developed advantageous features which increase the chances of survival and reproduction. If such a feature significantly enhances a species' survival, it is passed on from generation to generation, eventually leading to evolutionary changes. So long as the trait is advantageous, or at the very least not unfavorable (remains as a vestigial trait), it will remain in the gene pool until a more appropriate feature supersedes it. One such example is the pincer, developed in pincer-wielding crustaceans from primitive trilobites, resulting in an entropically favorable adaptation and providing them with an advantage over competing species (Szwast et al., 2002).
Pincers have evolved to become highly versatile and are used by organisms to perform various tasks (Simone & Van Der Meijden, 2021). For starters, pincers can serve as effective weapons, capable of inflicting damage, immobilizing, and manipulating an opponent or prey. Pincer-possessing species use them as weapons primarily for predation, defence and sexual contests (Lane, 2018).
Notable pincer-wielding animals
Scorpions are predatory arachnids that frequently use their pincers as weapons. In predation, scorpions use their chelae to grab prey, inflict damage and establish a firm grip on the prey's limbs to restrain its movements and (Simone & Van Der Meijden, 2021).
Birgus latro, or the coconut crab, is the largest terrestrial crustacean (Oka et al., 2016). Their chelae are adapted for use as a weapon against other terrestrial organisms and as a tool for accessing food in plants with rugged exteriors. These massive crustaceans are notable due to their unique use of their pincers. A predominant source of food for this species is coconuts, which have a tough exterior making it challenging to access the nutrient-full core. To access this resource, the coconut crab uses its chelae to crush and open coconuts (Oka et al., 2016). Their exceptional ability to crush and break rigid exteriors, using their chelae, allows them to access a wide variety of food, including fruit, nuts, and seeds.
The American lobster, Homarus americanus, also has pincers similar to crabs, as they both have a hard-shelled exterior and are used in underwater environments. Despite this, lobster pincers are utilized differently. A single lobster has two distinct pincers–one for capturing prey and the other for cutting. However, their pincers are particularly extraordinary as they allow them to dig effectively. This allows lobsters to live along the seafloor in burrows and crevices they have dug with their chelae (Wu & Zhou, 2011). They can also dig themselves into the ground for protection if they feel threatened with no other nearby available shelter. Furthermore, lobsters in freshwater are known to dig large tunnels in sand and mud (Berrill & Stewart, 1973).
While pincers are commonly recognized as tools of predation, some animals prioritize using pincers as biological shields. A prominent example of such is the hermit crab. Hermit crabs are infamous for occupying vacated mollusk shells as habitats and armour, offering protection to the lower soft abdomen. For further protection against environmental threats and predators, hermit crabs of the species coenobita brevimanus exhibit the unusual behaviour of using their disproportionately large left chela as a protective barrier when receding into the inner confines of the shell, which can be observed in Figure 1b. As the hermit crab shifts into the defensive position, the left pincer covers the entrance of the shell, fully enclosing the crab within a protective barrier (Hsu et al., 2018).
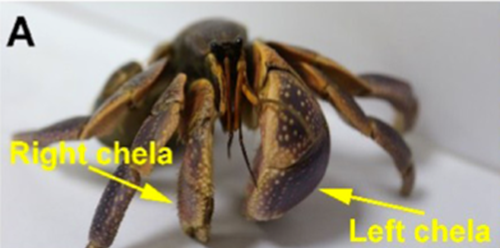
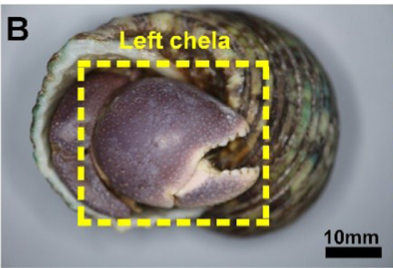
Fig. 1 Photographs of hermit crab outside of a gastropod shell and retracted inside. Note the disproportionately large left chela in (A) and its retracted position in (B) covering the shell entrance (Lin et al., 2021).
Chelae can also be used as a means of communication, specifically for mating signals and when competing with other individuals of the same sex. For instance, Uca pugnax, or the fiddler crab, uses its major (larger) pincer for fighting and mating signals (Levinton & Allen, 2005). In Figure 2, it can be observed that the generous size and vibrant colours of this large pincer are vital features not only for attracting potential mates but also for signalling the crabs' ability to fight and intimidate opponents. These pincers are also capable of regenerating. In fact, if the large chela of a fiddler crab is lost in battle, the smaller pincer will grow to the size of a large pincer for signalling and deterring opponents. However, it will never regain the same strength or fighting ability as an original pincer. Thanks to this pincer regeneration capability, the fiddler crab also uses its pincer to deceive opponents into believing it is capable of fighting and mating (Backwell et al., 2000).
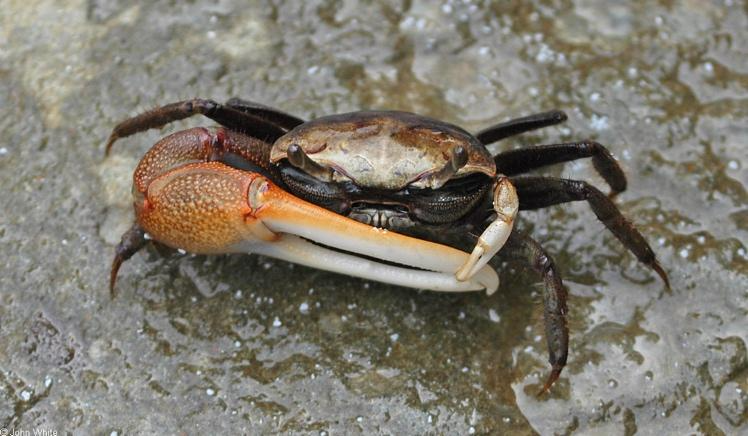
Fig. 2 Fiddler crab, Uca pugnax (White, 2005).
Pincers for predation
The ability to seize, restrain or incapacitate prey to prevent it from escaping is crucial to guarantee a successful hunt. Some species resort to chemical secretions to reduce the mobility of prey, such as venoms and glue-like secretions (Betz & Kölsch, 2004; Casewell et al., 2013), while other species use mechanical means to restrain their prey. The pincers of a scorpion are an example of such a weapon. When a scorpion detects prey, it uses its chelae to grab it. Once the target has been grasped, the scorpions may potentially use the stinger to inject venom to subdue it (Casper, 1985; Rein, 2003). As a matter of fact, it has been shown that whether or not the scorpion will prefer to use their stinger or chelae more in a confrontation depends on many factors, such as the size and toughness of the prey or threat (Casper, 1985; Edmunds & Sibly, 2010; Rein, 2003), the ontogenetic state of the scorpion (Casper, 1985; Cushing & Matherne, 1980), as well as the condition and morphology of their chela (Casper, 1985). Once the prey is successfully incapacitated, the scorpion will then use its chelae to manipulate it and bring it toward its chelicerae to consume it (Alexander, 1967; Bub, 1979; Polis, 1979).
The pincer also serves an essential role in sensing the environment. Scorpions are nocturnal and thus have limited vision (Polis, 1990). They rely on vibrational and chemical sensing to detect their prey. Therefore, their pincers are covered in chemo- and mechano-sensorial hair-like structures: the trichobothria and the sensilla. The sensilla are located at the extremity of the manus and form a constellation array used to detect chemical cues (Fet et al., 2006; Nisani et al., 2018). The trichobothria, located on the whole surface of the pincer detect airborne stimuli (Murayama & Willemart, 2019) while additional mechanoreceptors on their legs called slit sensilla, detect vibrations from the ground (Barth & Wadepuhl, 1975; Brownell & Farley, 1979).
Pincers for defense
Chelae are not only useful tools for hunting and attacking but are also highly effective weapons employed in active defence. The scorpions' primary strategy for defence is to inflict pain on the predator/attacker to either deter them from causing them any harm or to distract them and create the opportunity to escape (Simone & Van Der Meijden, 2021). Research on scorpions' defensive behaviour indicates that pincers are often used in combination with the stinger in such confrontations. Still, despite slight differences between different species of scorpions, the stinger remains the preferred weapon in these scenarios. In fact, not all species of scorpions use their chelae for defence and sometimes limit their defensive response to stinging only (Van Der Meijden et al., 2013). Nevertheless, some scorpion species also opt to use their pincers as shields, placing them over their head (prosoma) to protect themselves from frontal attacks, much like the hermit crab using its left pincer to cover up its shell opening (Newlands, 1969).
Anatomy of the scorpion pincers and the mechanism of movement
Figure 3 below illustrates the overall anatomy of the scorpion. In scorpions, the pincers consist of two segments, the manus (tibia) and the movable finger (tarsus). These segments are located at the extremity of the pedipalp, the second appendage (pair) from the front of the scorpion and are attached to the prosoma, informally known as the head of the arachnid. The tarsus is attached to the manus–the larger of the two segments–and behaves like a lever, pivoting on two joints located at the anteroventral side of the manus.

Fig. 3 Overview of the anatomy of a scorpion (Parabuthus transvaalicus, Buthidae): (A) dorsal view; (B) ventral view (Simone & Van Der Meijden, 2021).
The closing of the pincer is mainly controlled by the closing muscles contained in the manus (Figure 4). It is recognized that up to eight muscle bundles are involved in the closing of the chela. These muscles are connected to the tarsus by a ligament (Gilai & Parnas, 1970). Contrary to crustaceans (Behrens Yamada & G. Boulding, 1998), scorpions do not have muscles that open the pincer (Durale & Vyas, 1968; Gilai & Parnas, 1970). The opening of the pincer is the result of three mechanisms; the recoil of the elastic resilin in the joint, the hemocoel pressure buildup in the manus, and the elastic recoil of the sclerotized plates (arthrodial sternites) of the dorso-posterior interface of the movable finger and the manus (Alexander, 1967). The adduction and abduction of the chelae are controlled by the muscles in the third segment of the pedipalp, called the patella (Bowerman & Larimer, 1973).
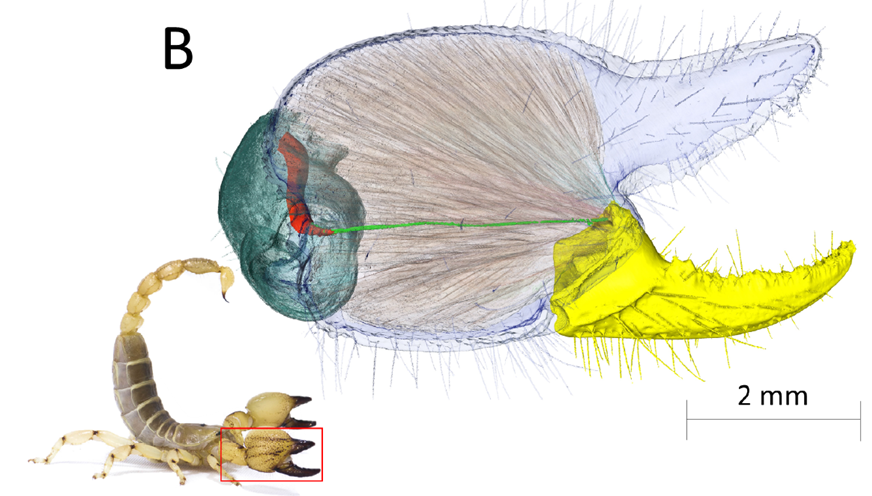
Fig. 4 Lateral view of the pincer of Scorpio maurus (Scorpionidae). This rendering created from synchrotron scan data shows the closing muscles and the ligament connecting the closing muscle of the patella to the movable finger. The components are indicated as follows: movable finger (yellow), cuticle of the manus (transparent blue), manus closing muscles (transparent orange striations), closing muscle in the patella (red), ligament connecting the closing muscle in the patella to the movable finger (light green), and cuticle of the patella (dark green) (Apodemes connecting the closing muscle in the manus to the movable finger not shown) (Simone & Van Der Meijden, 2021).
Anatomy of crab pincers and mechanism of movement
Like scorpions, crab pincers also comprise two components–the propodus and the dactylus. The fixed finger of the crab, known as the propodus, is connected to the articulating dactylus by an articular membrane. This forms a biarticular hinged joint that hinges about the lateral and medial fulcra of the pincer (Fujiwara & Kawai, 2016). Attached to the dactylus, are the opener and closer muscles of the pincer. Unlike scorpion pincers, the crab pincer has two muscles attached for opening and closing the dactyl independently. These opener and closer muscles are attached to the dactylus via the opener and closer apodemes respectively. Figure 5 illustrates the biarticular hinging mechanism of baptozius vinosus with labels for the apodemes, dactylus, and propodus and how they form a closed kinematic chain (CKC). A closed kinematic chain can simply be defined as a series of connected links and pivots forming a closed loop (Olsen, 2019). In the case of crab pincers, the dactylus, propodus, and opener and closer muscles form a permanent closed kinematic chain which provides the double benefit of allowing mobility of the dactylus as it pivots on the propodus while providing stability to the mechanism during adduction and abduction (Glaeser & Nachtigall, 2019; Olsen, 2019). This rather simple biarticular construction is shared with a wide variety of crustaceans and has been greatly altered to satisfy the needs of varying crab species (Fujiwara & Kawai, 2016).

Fig. 5 Illustration of the chela of Baptozius vinosus. Diagrams C and D illustrate the retraction and closing of the dactylus. Point (X) indicates the position of the propodus-dactylus joint consisting of the lateral and medial fulcra. Points (d) and (e) locate the positions of insertion of the closer apodeme (ca) and the opener apodeme (oa) to the ventral and dorsal margins of the proximal dactylus, respectively. The arrows in diagrams C and D indicate the direction of the opener and closer muscles, with contraction of the opener muscle in the opener apodeme causing the dactylus to actuate away from the propodus and contraction of the closer muscle forcing the dactylus to pinch against the propodus (Fujiwara & Kawai, 2016).
Pincer morphology
The variation of pincer morphology among different living organisms is significant, from the small pincers of a shrimp to the massive pincers of a lobster. The proposed mechanism to explain this variation is the evolution of the diversification of crustacean species resulting in a plethora of pincer morphologies. It is believed that the evolution of pincers is largely based on their use for predation and mating (Wolfe et al., 2021). Depending on the relative importance of either function, the morphology of the pincer is observed to display unique traits such as strong durable chelae for crushing prey in contrast to large lightweight pincers for ease of signalling to potential mates (Swanson et al., 2013).
In fact, for decapods (Crustacea), diet is believed to be the primary factor determining differences in chela morphology and size (Behrens Yamada & G. Boulding, 1998; Seed & Hughes, 1995). Research on intertidal crabs has shown that prey size selection in crabs depends on the pincer's gape, dentition pattern, and closing force (Behrens Yamada & G. Boulding, 1998). Crabs that possess larger and more powerful pincers have the option to feed on more heavily armoured organisms and, thus, are less selective of their prey. In contrast, crabs with weaker pincers are restricted to smaller and weaker prey and therefore consume less biomass (Behrens Yamada & G. Boulding, 1998). According to the same study, having a pincer with a larger gape does not seem to be related to the consumption of larger prey.
On the contrary, crabs with a smaller gape but stronger pincers attacked snails significantly larger than those attacked by crabs with wider but weaker chelae (Behrens Yamada & G. Boulding, 1998). Shell-breaking efficiency has also been shown to be related to pincer morphology. Specialized shell-breaking pincers tend to have large blunt molars and sharp shearing tips, enabling them to concentrate all of the force generated by the muscles onto one region of the prey's shell (Brown et al., 1979). As a result of this specialization for opening shells, crabs with pincer morphologies that are better adapted for opening hard-shelled prey can hunt on tougher prey when “easier” food becomes scarce, which creates selective pressure for thicker and larger predator-resistant shell morphologies in the prey, which in turn further selects for crabs with even more specialized shell opening pincers (Vermeij, 1976).
In scorpions, while there is no clear evidence that diet drives scorpion chela evolution, there exists evidence suggesting that pincer morphology is highly correlated with differences in performance and prey selection (Simone & Van Der Meijden, 2021). Between different scorpion families, and sometimes even between species belonging to the same genus, chela shape can range from having a stout and robust manus and short fingers to an especially slender manus and elongated fingers (Figure 6). In scorpion species with stouter chelae, pinch force is consistently higher than that in species with slender chelae (van der Meijden et al., 2010). These more robust species also tend to have a thicker cuticle (Kellersztein et al., 2019), which is believed to allow them to withstand the higher stress generated during maximum bite force (van der Meijden et al., 2012). More crushing power may be necessary when hunting for more formidable prey with a thicker exoskeleton, a feat that may be impossible for organisms with more slender chelae without the risk of breaking their fingers (van der Meijden et al., 2012).
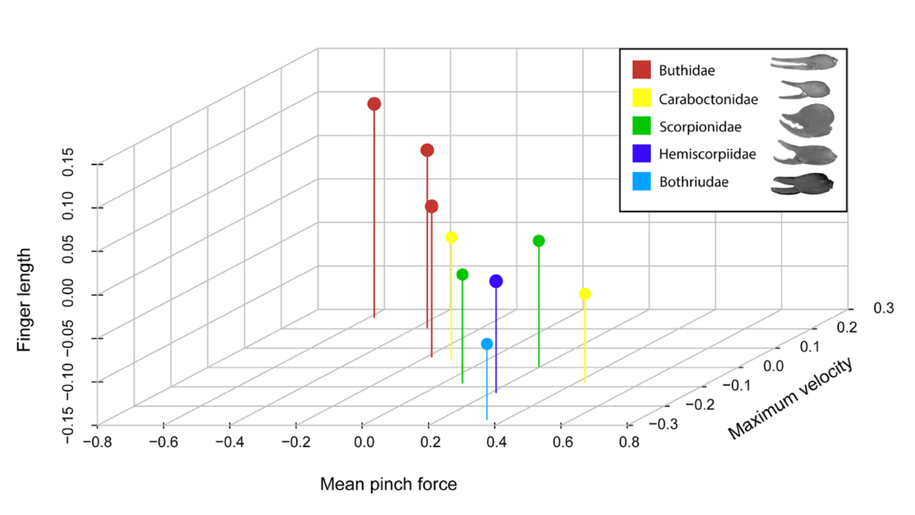
Fig. 6 Graph of the relationship between pincer finger length (size-corrected)(y-axis), pinch force (corrected for body size) (x-axis) and size-corrected maximum closing speed (z-axis). A member of each scorpion family is shown in the legend: Hottentotta gentili (Buthidae), Caraboctonus keyserlingi (Caraboctonidae), Pandinoides cavimanus (Scorpionidae), Hadogenes paucidens (Hemiscorpiidae), Bothriurus chilensis (Bothriuridae). Pattern: the scorpions with longer fingers appear to be faster but weaker. (Simone & Van Der Meijden, 2021).
For example, the exceptionally stout-pincered scorpion, Opistophthalmus carinatus (Scorpionidae), is known for feeding on hard-shelled terrestrial crustaceans when alternative food sources become scarce (Lamoral, 1971). On the other hand, Centruroides insulanus (Buthidae), a scorpion with smaller and weaker pincers, avoids hard-shelled prey and feeds on scarab beetles only if they are deprived of a hard elytra (Baerg, 1954).
While in scorpions, chelae variation only occurs between species or sexes, in some crustaceans, an individual can have both a robust crushing and slender cutting chela (Govind, 1989; Schenk & Wainwright, 2001).
In nature there is a pervading trade-off between speed and power. Cost-benefit theory is a consideration of the advantages and disadvantages that can be used to analyze the trade-off between speed and power in many species. This has been used to explain the consistent compromise observed in maximum speed and endurance across nature: in athletes, animals, and plants. For example, across many plant species, there is a trade-off between maximum photosynthetic rate and the ability to maintain photosynthesis under adverse conditions during unfavourable seasons, (Zhang et al., 2017). Cost-benefit theory can also be used to explain the reasons behind this trade-off in specific species, which can then be analyzed experimentally.
For certain species, pincers wielding immense power are not ideal for their functions within their environment. While some crustaceans aim to crush or bite with as much force as possible, for other species there is a trade-off between speed and power to fulfill multiple responsibilities. Specifically, a compromise between speed and power can be seen in the fiddler crab. The Uca Pugnax, or fiddler crab, is known for having two differently sized chela: a larger major chela used for fighting and courtship, and a smaller minor chela used for feeding. The focus of this trade-off is the major chelae. This trade-off is evident as the size of this large pincer is used for presentation rather than function (Schmitt, 2015). The fiddler crab's major chelae is a sexually-selected trait in males, meaning that the large size of the chelae is mainly used to attract female fiddler crabs. Additionally, the large size of this pincer is used during confrontation with other male crabs to intimidate opponents but is not advantageous while fighting (Schmitt, 2015).
The evolution of the fiddler crab pincer involved a trade-off of closing force for closing speed. This relative weakening with increasing body size may be due to the adaptive need for an increased ability to grasp an opponent. In fiddler crab combat, a quick grasp is a crucial advantage (Levinton & Allen, 2005). Since larger pincers are not advantageous in combat, this trade-off may also be a form of runaway selection, where a secondary sexual trait expressed in one sex becomes linked to a preference for the trait in the other sex. The trait and preference for the trait then continue in a loop of coevolution (Travers, 2017).
Chelae structure
Although the arthropods discussed–such as the scorpion, crab, and lobster–use their pincers for different purposes, their pincer structures are alike. The pincer or chela is part of the arthropod exoskeleton and is made of a chitin-protein matrix and calcium carbonate minerals arranged in rigid hierarchical layers (Zhou et al., 2010).
Some of the hierarchy begins to differ between species in the distinct layers of the pincer cuticle. The pincer cuticle is typically composed of 3 or 4 layers depending on the species. From the exterior of the pincer inwards, the layers are the epicuticle, the exocuticle, the extra-layer stacked lamella, and the endocuticle. Figure 7 shows an example of the hierarchical structure of two scorpion species, the Scorpio Maurus Palmatus (SP) and Buthus Occitanus (BO), where these 4 layers of the pincer cuticle can be identified. To properly understand the contrasting adaptations of the pincer, we must observe each layer's structure and mechanical properties.

Fig. 7 Diagram of pincer cuticle hierarchical structure of two scorpion species, Scorpio Maurus Palmatus and Buthus Occitanus (Kellersztein et al., 2019).
Microstructure of the pincer
Epicuticle
The first outermost layer of the pincer cuticle is called the epicuticle. This layer is characterized as thin, being only a few hundred nanometers, waxy, and lacking chitin fibers (Kellersztein et al., 2019). Due to its thickness and lack of chitin, this layer does not serve any significant mechanical reinforcement to the cuticle and instead acts as a waterproof barrier to protect the arthropod from the environment (Meyers et al., 2008). Without the epicuticle, the pincer would be degraded by water in its surroundings. In a study where two scorpion pincers were examined, it was found that the epicuticle of the Scorpio Maurus Palmatus (SP) species was divided into an inner and outer layer, whereas the Buthus Occitanus (BO) chela cuticle showed no signs of separation (Kellersztein et al., 2019). The BO endocuticle can be seen as having a spiky exterior in Figure 8c. This indentation is due to the ring-like pattern of the outer cuticle shown in the diagram above (Fig 7).
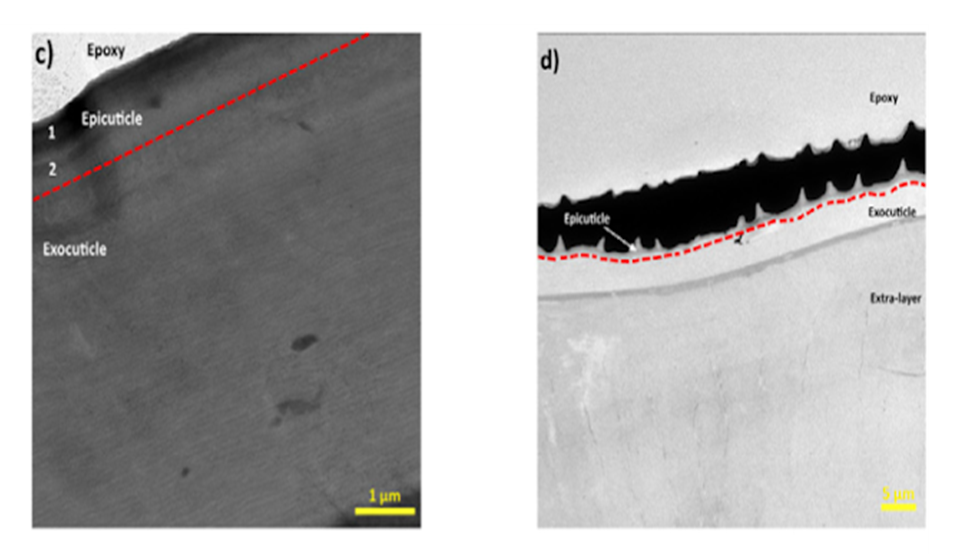
Fig. 8 TEM images of the epicuticle of the c) SP and d) BO chela (Kellersztein et al., 2019).
Exocuticle
The load-resistant properties of the chela begin to appear in the layer of the exocuticle. Common to all arthropods, the pincer exocuticle is one of the densest structures. However similar, the exocuticle stack and layers differ between species, providing unique mechanical advantages. In both the freshwater lobster and freshwater crabs, the exocuticle is made from stacked layers of Bouligand structures as seen in Figure 9b-d below.
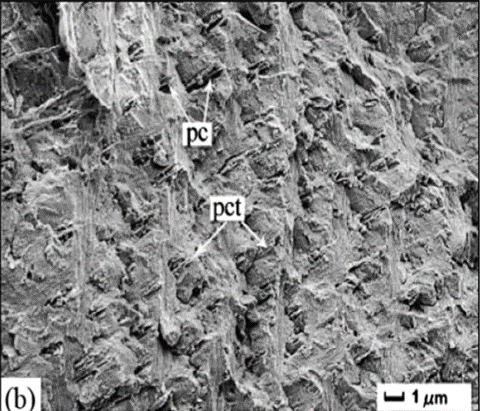
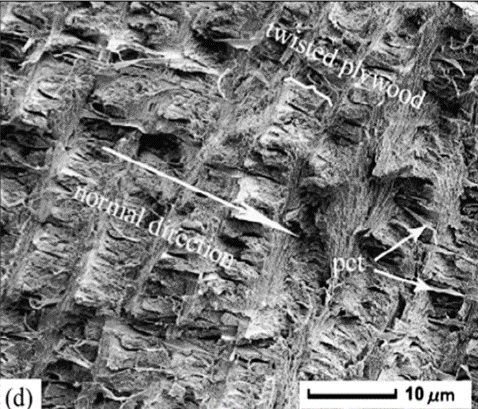
Fig. 9 Cross-section microstructure of b) freshwater lobster, P. clarkii, and d) freshwater crab, E. Sinensis, pincer, where b) pore canals and b-d) pore canal tubes are shown along with the Bouligand twisted plywood layers of the exocuticle and endocuticle (Zhou et al., 2010).
The Bouligand arrangement is a layered and rotated structure of chitin-protein fibers, making a gradual 180-degree twist, and is often referred to as a helicoid twisted plywood structure. This pattern is known for its structural strength. In a study by Zhou et al. (2010), the freshwater lobster, P. clarkii, and freshwater crab, E. Sinensis, shown in Figure 10a-b, were studied to examine the difference in pincer structure. It was shown that the exocuticle twisted plywood layers of the lobster were much thicker than that of the crab, being 11-15 μm and 6-8 μm in thickness, respectively. The lobster exocuticle has many pore canals (pc) and pore canal tubes (pct), as shown in Figure 9b-d, which allow for the transport of nutrients and binding of the tissue layers. These pores, however, decrease the overall density of the Bouligand layers, causing the lobster exocuticle to have a lower hardness modulus than that of the crab, despite being thicker. Although the presence of pore canals suggests a reduction in hardness modulus, the pore canals fibers have also been shown to be beneficial in reducing crack propagation in the left chelae of hermit crabs. The interactions between pore canals and layers provides additional adhesion between the layers which prevents the expansion of interfacial cracks (Lin et al., 2021). The high crack resistance and damage minimization demonstrated by the left chela is a critical feature essential to the pincer's function as a shield capable of enduring the forces inflicted by predators and the natural environment.

Fig. 10 Freshwater Lobster, P. clarkii (a) and freshwater crab, E. Sinensis (b) (Zhou et al., 2010).
The scorpion exocuticle differs slightly from the lobster and crab. An article comparing the exoskeletons of pincers from Scorpio Maurus Palmatus (SP) and Buthus Occitanus (BO) illustrates how the two exocuticles are different as shown in Figure 7 previously. It was observed that the SP scorpion exocuticle is 20 μm and is divided into 4 sublayers: 1) an angled fibrous layer, 2) a large layer of horizontal structures, 3) a vertically oriented high fiber density layer, and 4) a Bouligand arrangement layer. The BO scorpion, however, only has 2 sublayers of exocuticle. It is not made of the twisted plywood structure, and is approximately 10 μm thick. Due to the various orientation of its layers, the SP exocuticle is said to have a strength and stiffness advantage over BO. Different orientations of a layer reduce anisotropy, described as a material having different physical properties when measured along different axes. These differences in the SP and BO scorpion exocuticles are attributed to their evolutionary benefits. The added strength of the thick SP exocuticle gives the pincer a large strong grip that is beneficial for force production and sustaining external loads. The thinner BO chela exocuticle, however, allows for faster grip and accuracy, which benefits this scorpion for capturing small and mobile prey (Kellersztein et al., 2019). Overall, both scorpion chela exocuticles demonstrate lower mechanical properties than the lobster and crab because of the little to no amount of structural Bouligand plywood reinforcing them (Kellersztein et al., 2019).
An additional important feature of the exocuticle is the high curvature. A study on the left chela of hermit crabs by Lin et al. (2021) analyzed how the curvature of the left chela adds additional durability to the exocuticle. The high curvature of the pincers allows the exocuticle to maintain high structural integrity. Furthermore, the curvature of the exocuticle allows for more effective distribution of load on the exocuticle by reducing radial deformation of the chela as shown in Figure 11. By applying force against a surface with high curvature, the exocuticle undergoes compression rather than tension, creating a stable dome-like structure much like those found in arched architectural structures. The compression-aiding properties of mineral-based materials emphasize the necessity of high curvature chelae structure, capable of withstanding tremendous load while mitigating the weakening effect from the intrinsic brittleness of the calcium-chitin layers (Lin et al., 2021).
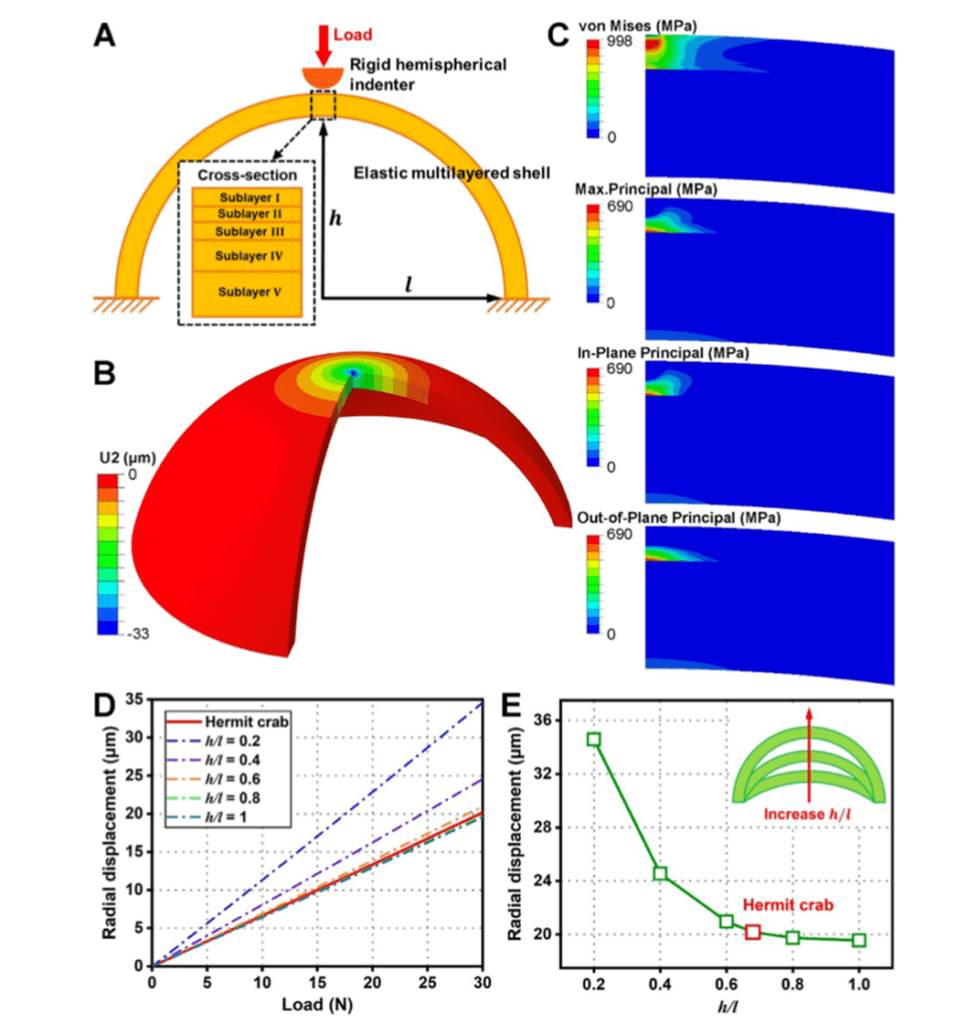
Fig. 11 Depicts the relationship between curvature and radial displacement. (A) Models the multi-layered chela structure while (B) models the radial displacement of the shell in space. The Von Mises and Principal stress are shown in (C). Sections (D) and (E) illustrate the reduction in radial displacement as curvature increases with graph (E) demonstrating an extreme decrease in deformation with increasing curvature.
Extra-layer
The extra-layer of stacked lamellae is an intermediate layer of the chela cuticle, scarcely found in arthropods. Neither crabs nor lobsters have this layer. Scorpions possess the extra-layer in their chela, yet it was not observed in their body cuticle, whereas the other layers were. This layer is known to contribute to mechanical balance in the pincer along with providing added resistance to bending loads, preventing damage to the pincer if used as a shield. The extra-layer is made of unidirectional chitin fibers forming tight lamellae, stacked vertically as shown above (Fig. 12g-h). The extra-layer is a dominant layer of the scorpion chela; where the SP extra-layer is ~50 μm thick, consisting of 30% of its chela cuticle, and the BO extra-layer is ~60 μm and 50% of the total structure. Like the lobster exocuticle, the extra-layer of these scorpions has pore canals. These canals are directed, perpendicular to the surface (Kellersztein et al., 2019).

Fig. 12 Transverse cross-section of extra-layer of the stacked lamella of g) SP and h) BO (Kellersztein et al., 2019).
The vertical extra-layer of the scorpion is thought to give a mechanical in-plane balance between the isotropic layers. However, due to the anisotropic property of having a layer pointing only in the vertical direction, as opposed to the exocuticle with several multidirectional layers, the extra-layer is shown to reduce local strength. This is compensated by the added balance to the entire cuticle due to its thickness. The added thickness to the cuticle provided by the large intermediate layer, improves resistance to high bending external loads. The increased radius of the chela cuticle creates an overall larger moment of inertia (Kellersztein et al., 2021). The moment of inertia is a physical quantity of an object which describes its resistance to changes in rotational motion. The moment of inertia I can be defined as Equation (i):

Where the moment of inertia I, is the sum of each particles mass mi times its distance squared from the center of rotation ri2 (Serway, 2014). Therefore, the added diameter provided by the extra-layer gives a mechanical advantage to the pincer, preventing it from bending under extreme stress, due to its higher moment of inertia.
Endocuticle
Common to all arthropod cuticles, the last layer of the chela is the endocuticle, a horizontal stack of lamellae made solely of strong Bouligand structures. The Bouligand layers can be seen as having a parabolic shape as shown in Figure 13i-j. The jagged parabola is a result of the oblique cuts in the twisted helical plywood structure, a cut where the structure is left with two angled sides. The addition of this interior layer of Bouligand arrangement displays advantages in limiting crack propagation in the cuticle, as a crack cannot follow a straight path along the helix shape (Kellersztein et al., 2019).
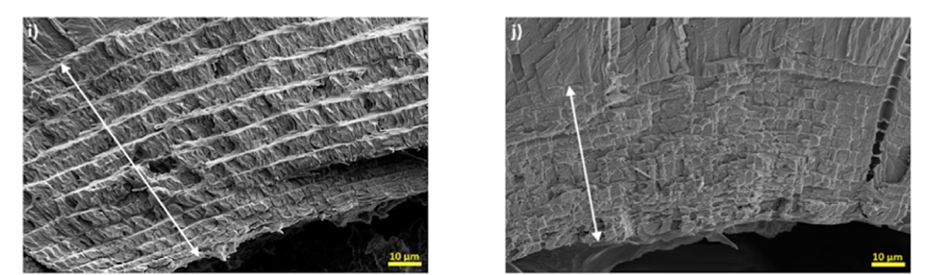
Fig. 13 Cross-section of the i) SP and j) BO endocuticle (Kellersztein et al., 2019).
In the lobster and crab cuticle, the endocuticle is shown to have lower mechanical properties than the exocuticle. The lobster exocuticle is found to have a mean hardness that is 13 times higher than that of its endocuticle. This is a result of having a much looser arrangement of Bouligand structures and more pore canals in both crab and lobster endocuticles (Zhou et al., 2010). A study observing the freshwater lobster and crab found that the lobster cuticle is shown to have an uneven gradient of hardness and elastic advantages as can be seen in Figure 14. The freshwater crab, however, can be seen (Fig. 15) to gradually decrease in mechanical properties throughout the layers of the cuticle. In total, it was found that the crab had a larger amount of twisted plywood structure in its layers than the lobster cuticle yet was thinner. This indicated that these structures were more densely packed in the crab chela and exhibited better overall mechanical properties of hardness and elasticity as shown in the graphs below.
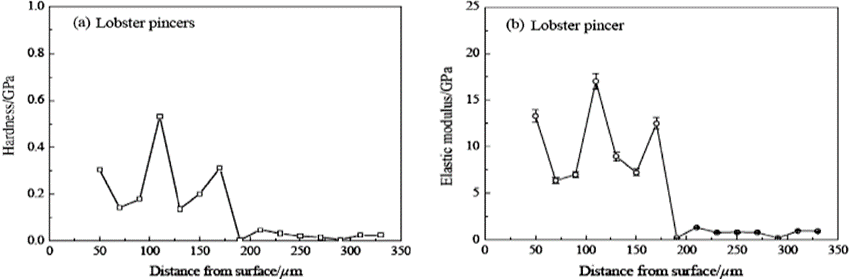
Fig. 14 Hardness and elastic modulus of lobster pincers along the cross-sectional direction of the pincer (Zhou et al., 2010).
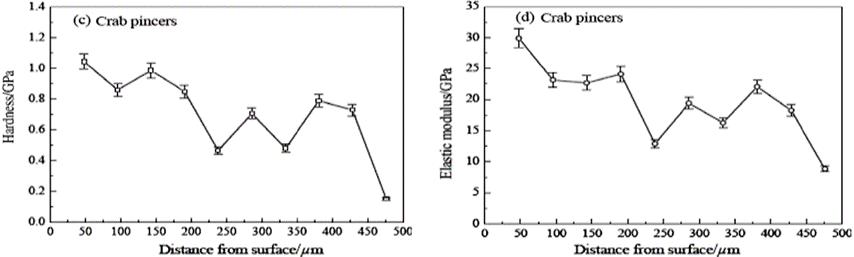
Fig. 15 Hardness and elastic modulus of lobster pincers along the cross-sectional direction of the pincer (Zhou et al., 2010).
As opposed to previously researched arthropod cuticles, the scorpions studied by Kellersztein et al. (2019) exhibited stronger mechanical properties in their endocuticle than their exocuticle. This difference is a result of having less Bouligand or no Bouligand structure in the exocuticle, while having an endocuticle made solely of the helicoidal arrangement. Of the two scorpion species studied, the SP chela endocuticle was thicker than that of BO. The SP endocuticle had a total thickness of 80 μm, consisting of ~50% of the total thickness, and followed a gradient of thickness of about 40-93 layers of twisted plywood. The BO endocuticle is ~40% of its cuticle thickness, being 45-50 μm with 13-35 layers of plywood and was stacked irregularly (Kellersztein et al., 2019). These aspects can be observed in the hierarchical structure shown in Figure 7.
Structural properties
Hardness and elasticity
As described, the crustacean and scorpion pincers must withstand substantial mechanical stresses when used in their natural environment, such as fighting and defence. These environmental factors have created a pincer that has evolved to withstand deformation or indentation and bending. Resistance to these mechanical stresses is known as the hardness and elastic modulus, a property of the material of the chela cuticle.
Researchers found that freshwater crabs and lobsters had mean hardness modulus values of 0.33 GPa, and 0.27 GPa, respectively, and an elastic modulus of 8.18 GPa and 5.44 GPa, respectively (Zhou et al., 2010). This data supports previous observations of the lobster and crab exoskeleton. Both the exocuticle and endocuticle of the freshwater crab had denser layers of Bouligand arrangement.
Bouligand structure
As is evident, the amount and stacking of the Bouligand structure is crucial to the performance of the pincer cuticle. It would thus be beneficial to delve deeper into how these structures provide physical reinforcement. The Bouligand laminate unit (BLU) is an asymmetrical helix consisting of a matrix of 40-100 laminae of chitin-protein fibers. The BLU has two dominant characteristics: its laminae twist off-axis about their corner, and the individual BLU shows an overall tilt. Both properties can be seen in Figure 16a-c. Because of these two features, the BLUs can be tightly packed together, as the off-axis twist of one structure matches the shape of the neighbor in all planes of their axes. The interlocking of these BLUs creates a strong shear resistance, where displacement between the BLUs is prevented while undergoing stress (Greenfeld et al., 2020).

Fig. 16 Bouligand laminate unit geometric model (Greenfeld et al., 2020).
Mechanical properties
How pincers generate a lot of power
Although the size of some pincers may seem modest, pincers can exert a large amount of force, depending on their function. Crustaceans can exert mean maximum forces greater than the forces exerted by most other animals using their extremities. These forces are due to the high stresses induced by the chelae closer muscle (Taylor, 2000). The forces produced by crustaceans and their predatory behaviour are associated with the morphology of the chelae. Based on their diet and environmental differences, crustaceans have varied chelae structures that allow them to produce the forces required for their survival (Elner & Campbell, 1981).
One of the highest force-producing crustaceans is Birgus latro, or the coconut crab. This crab can use its chelae to lift up to 30 kg, and the force exerted by a large coconut crab (4 kg) can be up to 3300 N (Oka et al., 2016). Although the forces exerted by the chelae of crustaceans vary greatly, the force exerted is predominantly determined by pincer size.
In the pincers of the coconut crab, the pinching forces are exerted by the closure of a moveable finger, the dactyl. The efficiency of force transfer to the closure of the dactyl from the contraction of the chela closer muscle is largely a function of the mechanical advantage of the chela lever system. This system and its resulting forces are the product of the closer muscle acting on the dactyl, treating it as a lever arm. The chela lever system can be seen in Figure 17 which illustrates the chela length, and the closer apodeme, which provides attachment points for the closer muscles. The closer muscle produces exceedingly high levels of maximum muscle stress to apply extensive force via the lever system (Taylor, 2000). The maxima of these forces can be analyzed by the sliding filament model of muscle contraction. This model explains that maximum muscle stress should increase isometrically with sarcomere, or muscle length, as long as other factors are constant (Taylor, 2000). The increase should be constant with changes in the muscle length because there is a linear relationship between stress and the overlap of filaments within the muscle. This is accurate in coconut crabs and explains their exceptionally high force production as they have a considerable concentration of muscle fibers featuring particularly long sarcomeres (Oka et al., 2016).

Fig. 17 The measurements used for chela length (CL), chela height (CH), and chela width (CW), and the placement of a pressure measurement sensor (Oka et al., 2016).
Muscle stress in the coconut crab can be calculated using Equation (ii) where F is the applied force to the dactyl base, A is the area of one side of the closer apodeme and is the mean angle of the fiber attached to the closer apodeme of the chelae (Taylor, 2000).

This relationship between sarcomere length and pinching force can also be seen by measuring the force exerted by coconut crabs in Newtons and comparing it to the body weight of these crabs, which is proportional to their sarcomere length. This can be seen in Figure 18 where the log of the body weight (g) is compared to the log of the pinching force (N), and is shown to have a linear relationship (Taylor, 2000).

Fig. 18 The relationship between body weight and pinching force of the coconut crabs (Oka et al., 2016).
The trade-off between speed and power
The pincers of a fiddler crab operate as a closed kinematic chain, or a series of fixed and unfixed rigid bodies that allows movement in one joint to cause movement in every other joint. This can be seen in Figure 19 where one chela is fixed, and the other acts in a lever system. One finger of the chelae is fixed while the moveable finger, or dactyl, is closed against it via the contraction of pennate muscles attached to a blade-like apodeme, rotating the dactyl about a fulcrum. The closing force is proportional to the muscle cross-sectional area (Levinton & Allen, 2005). Chela closing force is a function of this lever system and is determined by the proportions of the lever arms on each side of the fulcrum (Levinton & Allen, 2005). The major chelae of males are fully functional, yet as male fiddler crabs grow, their major chelae change reducing mechanical advantage and relative closing force with increasing pincer size.

Fig. 19 Major chela of a male Uca Pugnax. Measurements taken of crabs in this study: A-A, chela length, B-B, dactyl length, C-C chela height, D-D length of the pollex (Levinton & Allen, 2005).
While large chelae produce high absolute closing force, they exert less than would be exact if pincer proportions were isometric. In the fiddler crab, this is concluded as a decrease in mechanical advantage with increasing pincer size. To analyze the chela force, Equation (iii) is used to relate the muscle cross-sectional area, mechanical advantage, chela length, and an index of closing force.

The muscle cross-sectional area, MCA, is calculated as the apodeme area. The ratio of dactyl height at the base to dactyl length or the ratio of levers in/out is defined as the mechanical advantage, MA (Warner & Jones, 1976). Under isometry, or under equal conditions, the length of the chela is equal to the index of closing force, ICF (CL = ICF). This would occur if the force produced by the chela increased proportionally with the length of the chela, or dactyl. In this equation, a closing force estimated with different size indices scaled to the chelae length, using an exponent much less than the value that is expected under isometry, confirms that the proportional decrease in the closing force of the major pincer results from unequal relationships of muscle cross-sectional area and mechanical advantage to chelae length (Levinton & Allen, 2005).
While the relationship between the index of closing force, the chela length, apodeme area, and closing velocity of the tip of the dactyl are all proportional and increase isometrically, the mechanical advantage and chela length are inversely proportional. This relationship can be seen in Figure 20 below, where the chela length is compared to the mechanical advantage and where pincer length is compared to the index of closing force in a study analyzing the mechanical advantage of fiddler crabs and its relation to their pincer size (Levinton & Allen, 2005).
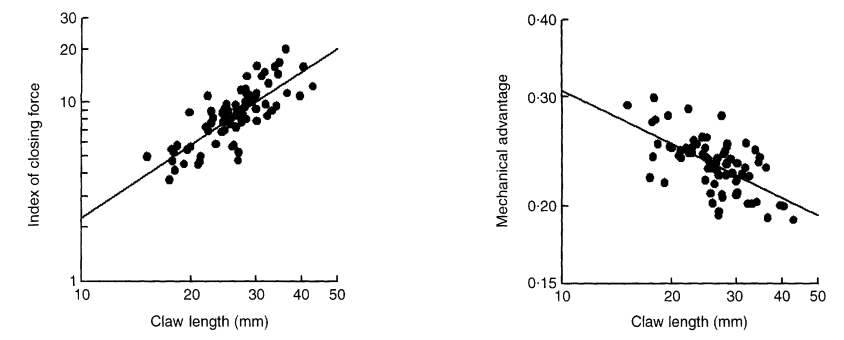
Fig. 20 The relationship between relative index of closing force and chela length for Uca Pugnax, calculated with direct measures of apodeme area and mechanical advantage. Also the relationship between mechanical advantage and chela length for Uca Pugnax (Levinton & Allen, 2005).
Therefore, as the mechanical advantage decreases with increasing pincer size, the closing force must concurrently decrease as the closing velocity increases. Additionally, the angle of insertion is constant with respect to chela size for multiple species of crab (Schenk & Wainwright, 2001).
As in decapods, scorpions having a long pincer may give them an advantage when hunting for prey by allowing their pincers to have a wider gape and a higher closing speed of the tips of the fingers. But as for the fiddler crab, from the perspective of lever mechanics, longer fingers, and thus a longer out-lever, lowers mechanical advantage, resulting in the generation of a smaller pinch force (Simone & van Der Meijden, 2017). Having faster pincers thus restricts the bearer to consuming softer and smaller prey and contributes to the niche partitioning between different species of scorpions (Simone & Van Der Meijden, 2021).
Conclusion
In analyzing the structural, mechanical, and physical properties of pincers, it is clear that pincers serve as an essential tool for the survival of many creatures. For scorpions, pincers provide a method for capturing prey and deterring predators. In crabs, pincers vary substantially in morphology depending on how the pincer is used. Although a relatively simple design at first glance, the functional properties of pincers demonstrate diverse intricacies that contribute to their usefulness in different animals. While some creatures favor immense crushing power, the hunting behaviours of others prioritize speed. In the coconut crab, the pincer exhibits the ability to generate a tremendous amount of force, allowing it to break and consume coconuts. In hermit crabs, the high curvature of the left chelae prevents superb structural stability enabling the crab to use the pincer as a protective gate. For each of the arthropods discussed, the multi-layered hierarchical structure and Bouligand architecture provide the fundamental source of durability to the pincer, allowing them to withstand the forces necessary to crush, pinch, and shield. Furthermore, the fibrous pore canals help guide and reduce crack propagation to mitigate damage to the pincer.
A considerable amount of research has been conducted studying the structural, mechanical, and physical properties of pincers. For example, researchers speculate that the multilayered hierarchical structure in chelae could prevent insight into making more robust multi-layered materials (Lin et al., 2021). Furthermore, the Bouligand twisted architecture is also a topic of interest to engineers hoping to replicate the strength it provides (Yang et al., 2017). Pincer mimetic machines are also currently in use. For industrial purposes, crab-type devices are used for high-efficiency loading in mineral mining and earthwork (Luo & Li, 2012). These are just a few examples of how pincers are used in biomimetic devices. Given how effective and diverse pincers are in nature, it is understandable that pincers have great potential and provide a large plethora of inspiration for potential uses in biomimetics.
References
Alexander, A. J. (1967). Problems of limb extension in the scorpion, opisthophthalmus latimanus koch [Article]. Transactions of the Royal Society of South Africa, 37(3), 165-181. https://doi.org/10.1080/00359196709519065
Backwell, P. R. Y., Christy, J. H., Telford, S. R., Jennions, M. D., & Passmore, N. I. (2000). Dishonest signalling in a fiddler crab [Article]. Proceedings of the Royal Society B: Biological Sciences, 267(1444), 719-724. https://doi.org/10.1098/rspb.2000.1062
Baerg, W. J. (1954). Regarding the Biology of the Common Jamaican Scorpion1, 2. Annals of the Entomological Society of America, 47(2), 272-276. https://doi.org/10.1093/aesa/47.2.272
Barth, F. G., & Wadepuhl, M. (1975). Slit sense organs on the scorpion leg (Androctonus australis L., Buthidae) [Article]. Journal of morphology, 145(2), 209-227. https://doi.org/10.1002/jmor.1051450207
Behrens Yamada, S., & G. Boulding, E. (1998). Claw morphology, prey size selection and foraging efficiency in generalist and specialist shell-breaking crabs [Article]. Journal of Experimental Marine Biology and Ecology, 220(2), 191-211. https://doi.org/10.1016/S0022-0981(97)00122-6
Berrill, M., & Stewart, R. (1973). Tunnel-Digging in Mud by Newly-Settled American Lobsters, Homarus americanus. Journal of the Fisheries Research Board of Canada, 30(2), 285-287. https://doi.org/10.1139/f73-048
Betz, O., & Kölsch, G. (2004). The role of adhesion in prey capture and predator defence in arthropods [Article]. Arthropod Structure and Development, 33(1), 3-30. https://doi.org/10.1016/j.asd.2003.10.002
Bowerman, R. F., & Larimer, J. (1973). Structure and physiology of the patella-tibia joint receptors in scorpion pedipalps [Article]. Comparative Biochemistry and Physiology — Part A: Physiology, 46(1), 139-142,IN135-IN110,143-151. https://doi.org/10.1016/0300-9629(73)90567-7
Brown, S. C., Cassuto, S. R., & Loos, R. W. (1979). Biomechanics of chelipeds in some decapod crustaceans [Article]. Journal of Zoology, 188(2), 143-159. https://doi.org/10.1111/j.1469-7998.1979.tb03397.x
Brownell, P., & Farley, R. D. (1979). Detection of vibrations in sand by tarsal sense organs of the nocturnal scorpion, Paruroctonus mesaensis [Article]. Journal of Comparative Physiology □ A, 131(1), 23-30. https://doi.org/10.1007/BF00613080
Bub, K. (1979). PREY CAPTURE.BY THE SCORPION hIADRURUS ARIZONENSIS EWING (SCORPIONES: VAEJOVIDAE).
Casewell, N. R., Wüster, W., Vonk, F. J., Harrison, R. A., & Fry, B. G. (2013). Complex cocktails: The evolutionary novelty of venoms [Review]. Trends in Ecology and Evolution, 28(4), 219-229. https://doi.org/10.1016/j.tree.2012.10.020
Casper, G. S. (1985). Prey Capture and Stinging Behavior in the Emperor Scorpion, Pandinus Imperator (Koch) (Scorpiones, Scorpionidae). The Journal of Arachnology, 13(3), 277-283. http://www.jstor.org/stable/3705310
Cushing, B. S., & Matherne, A. (1980). STINGER UTILIZATION AND PREDATION IN THE SCORPION PARUROCTONUS BOREUS. The Great Basin Naturalist, 40(2), 193-195. http://www.jstor.org/stable/41711742
Durale, M., & Vyas, A. (1968). The structure of the chela of Heterometrus sp. and its mode of operation. Bulletin of the Southern California Academy of Sciences, 67(4), 240-244.
Edmunds, M. C., & Sibly, R. M. (2010). Optimal sting use in the feeding behavior of the scorpion Hadrurus spadix [Article]. Journal of Arachnology, 38(1), 123-125. https://doi.org/10.1636/Hi09-38.1
Elner, R. W., & Campbell, A. (1981). Force, function and mechanical advantage in the chelae of the American lobster Homarus americanus (Decapoda: Crustacea) [Article]. Journal of Zoology, 193(2), 269-286. https://doi.org/10.1111/j.1469-7998.1981.tb03444.x
Fet, V., Soleglad, M. E., Neff, D. P. A., & Brewer, M. S. (2006). Constellation array: a new sensory structure in scorpions (Arachnida: Scorpiones).
Fujiwara, S. I., & Kawai, H. (2016). Crabs grab strongly depending on mechanical advantages of pinching and disarticulation of chela [Article]. Journal of morphology, 277(10), 1259-1272. https://doi.org/10.1002/jmor.20573
Gilai, A., & Parnas, I. (1970). Neuromuscular Physiology of the Closer Muscles in the Pedipalp of the Scorpion Leiurus Quinquestriatus. Journal of Experimental Biology, 52(2), 325-344. https://doi.org/10.1242/jeb.52.2.325
Glaeser, G., & Nachtigall, W. (2019). Shape, Movement, Lever. In The Evolution and Function of Biological Macrostructures (pp. 0-35). Springer Berlin Heidelberg. https://doi.org/10.1007/978-3-662-59291-5_1
Govind, C. K. (1989). Asymmetry in lobster claws [Article]. American Scientist, 77(5), 468-474. https://www.scopus.com/inward/record.uri?eid=2-s2.0-0024895679&partnerID=40&md5=1039ded9300eef8e52d0b9a64b4f1a9d
Greenfeld, I., Kellersztein, I., & Wagner, H. D. (2020). Nested helicoids in biological microstructures [Article]. Nature Communications, 11(1), Article 224. https://doi.org/10.1038/s41467-019-13978-6
Hsu, C. H., Otte, M. L., Liu, C. C., Chou, J. Y., & Fang, W. T. (2018). What are the sympatric mechanisms for three species of terrestrial hermit crab (Coenobita rugosus, C. Brevimanus, and C. Cavipes) in coastal forests? [Article]. PLoS ONE, 13(12), Article e0207640. https://doi.org/10.1371/journal.pone.0207640
Kellersztein, I., Cohen, S. R., Bar-On, B., & Wagner, H. D. (2019). The exoskeleton of scorpions' pincers: Structure and micro-mechanical properties [Article]. Acta Biomaterialia, 94, 565-573. https://doi.org/10.1016/j.actbio.2019.06.036
Kellersztein, I., Greenfeld, I., & Wagner, H. D. (2021). Structural analysis across length scales of the scorpion pincer cuticle [Article]. Bioinspiration and Biomimetics, 16(2), Article 026013. https://doi.org/10.1088/1748-3190/abd2d2
Lamoral, B. H. (1971). Predation on terrestrial molluscs by scorpions in the Kalahari Desert. Annals of the Natal Museum, 21(1), 17-20. https://doi.org/doi:10.10520/AJA03040798_669
Lane, S. M. (2018). What is a weapon? [Article]. Integrative and comparative biology, 58(6), 1055-1063. https://doi.org/10.1093/icb/icy083
Levinton, J. S., & Allen, B. J. (2005). The paradox of the weakening combatant: Trade-off between closing force and gripping speed in a sexually selected combat structure [Article]. Functional Ecology, 19(1), 159-165. https://doi.org/10.1111/j.0269-8463.2005.00968.x
Lin, W., Liu, P., Li, S., Tian, J., Cai, W., Zhang, X., Peng, J., Miao, C., Zhang, H., Gu, P., Wang, Z., Zhang, Z., & Luo, T. (2021). Multi-scale design of the chela of the hermit crab Coenobita brevimanus [Article]. Acta Biomaterialia, 127, 229-241. https://doi.org/10.1016/j.actbio.2021.04.012
Luo, Z., & Li, S. (2012). Optimization design for crank arc guide bar gathering mechanism of crab claw type loading machine.Applied Mechanics and Materials 4th International Conference on Mechanical and Electrical Technology, ICMET 2012, July 24, 2012 – July 26, 2012, Kuala Lumpur, Malaysia.
Meyers, M. A., Chen, P. Y., Lin, A. Y. M., & Seki, Y. (2008). Biological materials: Structure and mechanical properties [Review]. Progress in Materials Science, 53(1), 1-206. https://doi.org/10.1016/j.pmatsci.2007.05.002
Murayama, G. P., & Willemart, R. H. (2019). Are trichobothria used in terrestrial prey capture by the yellow scorpion Tityus serrulatus Lutz & Mello, 1922 (Buthidae)? [Article]. Arachnology, 18(3), 287-290. https://doi.org/10.13156/arac.2019.18.3.287
Newlands, G. (1969). Scorpion defensive behaviour. African Wildlife, 23, 147-153.
Nisani, Z., Honaker, A., Jenne, V., Loya, F., & Moon, H. (2018). Evidence of airborne chemoreception in the scorpion Paruroctonus marksi (Scorpiones: Vaejovidae) [Article]. Journal of Arachnology, 46(1), 40-44. https://doi.org/10.1636/JoA-16-092.1
Oka, S. I., Tomita, T., & Miyamoto, K. (2016). A mighty claw: Pinching force of the coconut crab, the largest terrestrial crustacean [Article]. PLoS ONE, 11(11), Article e0166108. https://doi.org/10.1371/journal.pone.0166108
Olsen, A. M. (2019). A mobility-based classification of closed kinematic chains in biomechanics and implications for motor control. Journal of Experimental Biology, 222(21), jeb195735. https://doi.org/10.1242/jeb.195735
Polis, G. A. (1979). Prey and feeding phenology of the desert sand scorpion Pamroctonus mesaensis (Scorpionidae: Vaejovidae) [Article]. Journal of Zoology, 188(3), 333-346. https://doi.org/10.1111/j.1469-7998.1979.tb03419.x
Polis, G. A. (1990). The biology of scorpions. Stanford University Press.
Rein, J. O. (2003). Prey capture behavior in the East African scorpions Parabuthus leiosoma (Ehrenberg, 1828) and P. pallidus Pocock, 1895 (Scorpiones: Buthidae).
Schenk, S. C., & Wainwright, P. C. (2001). Dimorphism and the functional basis of claw strength in six brachyuran crabs [Article]. Journal of Zoology, 255(1), 105-119. https://doi.org/10.1017/S0952836901001157
Schmitt, S. E. (2015). That Crab With One Big Claw. SiOWfa15 Science in Our World Certainty and Controversy. https://sites.psu.edu/siowfa15/2015/10/05/that-crab-with-one-big-claw/
Seed, R., & Hughes, R. N. (1995). Criteria for prey size-selection in molluscivorous crabs with contrasting claw morphologies [Article]. Journal of Experimental Marine Biology and Ecology, 193(1-2), 177-195. https://doi.org/10.1016/0022-0981(95)00117-4
Serway, R., Jewett, J. (2014). In Physics for Scientist and Engineers with Modern Physics (9 ed., pp. 303). Cengage Learning.
Simone, Y., & van Der Meijden, A. (2017). Fast and fine versus strong and stout: a trade-off between chela closing force and speed across nine scorpion species. Biological Journal of the Linnean Society, 123(1), 208-217. https://doi.org/10.1093/biolinnean/blx139
Simone, Y., & Van Der Meijden, A. (2021). Armed stem to stinger: A review of the ecological roles of scorpion weapons [Review]. Journal of Venomous Animals and Toxins Including Tropical Diseases, 27. https://doi.org/10.1590/1678-9199-JVATITD-2021-0002
Swanson, B. O., George, M. N., Anderson, S. P., & Christy, J. H. (2013). Evolutionary variation in the mechanics of fiddler crab claws. BMC Evolutionary Biology, 13(1), 137. https://doi.org/10.1186/1471-2148-13-137
Szwast, Z., Sieniutycz, S., & Shiner, J. S. (2002). Complexity principle of extremality in evolution of living organisms by information-theoretic entropy. Chaos, Solitons and Fractals, 13(9), 1871-1888. https://doi.org/10.1016/S0960-0779(01)00204-1
Taylor, G. M. (2000). Maximum force production: Why are crabs so strong? [Article]. Proceedings of the Royal Society B: Biological Sciences, 267(1451), 1475-1480. https://doi.org/10.1098/rspb.2000.1167
Travers, L. M. (2017). Runaway Selection. In J. Vonk & T. Shackelford (Eds.), Encyclopedia of Animal Cognition and Behavior (pp. 1-6). Springer International Publishing. https://doi.org/10.1007/978-3-319-47829-6_430-1
Van Der Meijden, A., Coelho, P. L., Sousa, P., & Herrel, A. (2013). Choose your weapon: Defensive behavior is associated with morphology and performance in scorpions [Article]. PLoS ONE, 8(11), Article e78955. https://doi.org/10.1371/journal.pone.0078955
van der Meijden, A., Herrel, A., & Summers, A. (2010). Comparison of chela size and pincer force in scorpions; getting a first grip [Article]. Journal of Zoology, 280(4), 319-325. https://doi.org/10.1111/j.1469-7998.2009.00628.x
van der Meijden, A., Kleinteich, T., & Coelho, P. (2012). Packing a pinch: Functional implications of chela shapes in scorpions using finite element analysis [Article]. Journal of Anatomy, 220(5), 423-434. https://doi.org/10.1111/j.1469-7580.2012.01485.x
Vermeij, G. J. (1976). Interoceanic differences in vulnerability of shelled prey to crab predation [Article]. Nature, 260(5547), 135-136. https://doi.org/10.1038/260135a0
Warner, G. F., & Jones, A. R. (1976). Leverage and muscle type in crab chelae (Crustacea: Brachyura) [Article]. Journal of Zoology, 180(1), 57-68. https://doi.org/10.1111/j.1469-7998.1976.tb04663.x
White, J. (2005). Fiddler Crab (Uca pugnax). In F. C. (Ucapugnax).jpg (Ed.), (Vol. 171983 Bytes). Animal Photo Album.
Wolfe, J. M., Luque, J., & Bracken-Grissom, H. D. (2021). How to become a crab: Phenotypic constraints on a recurring body plan. BioEssays, 43(5), 2100020. https://doi.org/https://doi.org/10.1002/bies.202100020
Wu, Z. W., & Zhou, F. (2011). Structure and mechanical properties of pincers for freshwater lobster [Article]. Science China Technological Sciences, 54(3), 650-658. https://doi.org/10.1007/s11431-010-4224-9
Yang, Y., Chen, Z., Song, X., Zhang, Z., Zhang, J., Shung, K. K., Zhou, Q., & Chen, Y. (2017). Biomimetic Anisotropic Reinforcement Architectures by Electrically Assisted Nanocomposite 3D Printing [Article]. Advanced Materials, 29(11), Article 1605750. https://doi.org/10.1002/adma.201605750
Zhang, Y. J., Sack, L., Cao, K. F., Wei, X. M., & Li, N. (2017). Speed versus endurance tradeoff in plants: Leaves with higher photosynthetic rates show stronger seasonal declines [Article]. Scientific Reports, 7, Article 42085. https://doi.org/10.1038/srep42085
Zhou, F., Wu, Z., Wang, M., & Chen, K. (2010). Structure and mechanical properties of pincers of lobster (Procambarus clarkii) and crab (Eriocheir Sinensis) [Article]. Journal of the Mechanical Behavior of Biomedical Materials, 3(6), 454-463. https://doi.org/10.1016/j.jmbbm.2010.05.001