An Evolutionary Analysis of the Chemical Composition of Marine Suction Cups
Juan Manuel Rocha Angel, Mika Chang, Emily Martin, Tokiniaina Raharison Ralambomihanta
Abstract
Suction cups are important adaptations for aquatic animals, allowing for predation, locomotion, stability, and other species-specific functions. The chemical structure of each suction cup is designed by nature to be as energetically efficient as possible in performing the suction cup's species-specific purpose, giving the suction cup chemical properties that enhance suction without excessive energy input. This paper explores the unique chemical structures of the suction cups of the remora fish, northern clingfish, suckermouth catfish, and octopus. This discussion includes chemical tissue composition, cell composition, arrangement of tissue fibers, and the effect of these characteristics on overall musculature function. The chemical composition and function of mucus secreted to enhance adhesion are discussed in detail for the clingfish and octopuses, and the polymerization and cross-linking of friction-generating filaments on the clingfish suction cup is explored at length.
Introduction
Multiple species have adapted different suction apparatus over time. In the case of aquatic species, their suction cups are essential for nutrition, movement and livelihood. While suction primarily relies on hydrodynamics at a macro level due to a force that pushes the suction cup to a substrate because of a pressure difference, the chemistry at a micro level is also a key factor in making suction possible. Underwater suction cups share common characteristics across various species, for example, the presence of a soft lip. This structure must be flexible enough to properly form a seal with the substrate while still being sufficiently stiff to maintain the seal when suction is created. Due to these characteristics, most soft lips of suction cups share similar chemical compositions. This article first explores the tissue and cell composition of various suction cups. Remoras, clingfish, and catfish use a system of muscle and connective tissue to give their suction cups the right physical properties for their specific uses. Collagen, specifically, is one of the most important proteins that make up suckers. It is the most common protein in the animal kingdom, and in this case, it gives soft lips elastic capabilities. The synthesis and structure of this protein will be discussed as a common background for all species.
Supporting structures such as papillae, muscles, and other microstructures are also essential for the proper function of suction mechanisms. In the case of clingfish, this paper will explore the different tissue layers, from the papillae to the disc's musculature and skeletal system, that play critical roles in creating and maintaining suction. Octopuses have adapted a semi-autonomous sensing system that gives suckers extremely precise and fast control over suction. In the case of the suckermouth catfish, the specific needs related to the environment and consequent specific tissue composition of the suction cup's margin will be examined. This paper will also investigate how both catfish and clingfish have developed microstructures that help generate friction between the disc's margin and the surface to help maintain adhesion. For the clingfish, the properties of actin will be explored in detail to better understand its role on the surface of the papillae. Besides friction, another way to improve adhesion is to use Stefan adhesion by secreting mucus. Therefore, an analysis of mucus protein composition is needed to understand its properties, such as viscosity.
Background: collagen is key
Each organism explored in this paper has unique chemical structures adapted to their distinctive environments and competitive needs, contributing to their energetic efficiency. These species' suction cups serve wildly different purposes: the large octopus uses its suction cups to locomote gracefully, while the tiny remora fish hangs onto the skin of a shark for dear life as a hitchhiker. Despite their differing purposes, in order to adapt to irregular surfaces and create a tight seal, the suction cup on each of these animals must be strong yet very flexible. This contributes to the most notable commonality between all the suctioning structures in the different species: collagen. To understand the important role that collagen plays in the tough but flexible nature of these suction cups, we must first understand the chemical structure of collagen.
Collagen is the most common protein in the animal kingdom. In the structure of collagen, three parallel polypeptide chains (each one a left-handed polyproline II-type (PPII) helix) self-assemble into a right-handed helix structure. These three parallel polypeptide chains are wound into the right-handed helix structure by a network of hydrogen bonds between the individual amino acids, as shown in Figure 1 (Shoulders & Raines, 2009). These right-handed helices—also called tropocollagen (TC)—self-assemble to form the basis for intricate systems of tissues.

Fig. 1 Model of collagen self-assembly. a) External view of the right-handed helix tropocollagen chain. b) Axis view of the right-handed helix tropocollagen chain, showing the supercoiling of three PPII helix polypeptide chains (ball-and-stick, ribbon, and space-filling models are each used for one of the three chains) (Shoulders & Raines, 2009).
Hydrogen bonding between the individual PPII helices is immensely stabilizing for the collagen structure, with each hydrogen bond reducing the energy of the molecule by approximately 2.0 kcal/mol. Another stabilizing factor lies in the fact that collagen has a high percent composition of two amino acids, Proline (Pro) and Hydroxyproline (Hyp). Their prevalence allows the strands to self-assemble into the PPII helices with very low energy input, efficiently reducing the molecule's entropy (Shoulders & Raines, 2009).
The collagen helix is also stabilized by prioritizing favorable stereochemical arrangement at specific sites in the helix. One of the most crucial is the gauche configuration (shown in Figure 2) caused by the electron-withdrawing group, labelled R, that stabilizes the exo-pucker, favored over the endo configuration due to the minimization of steric effects (Shoulders & Raines, 2009).

Fig. 2 Newman projection representation of the gauche conformation leading to an exo pucker (Shoulders & Raines, 2009).
As mentioned above, at a macro scale, the tropocollagen chains self-assemble, adding yet another layer to the collagen hierarchy, forming fibers that comprise most animal tissues. In order to self-assemble, the individual tropocollagen chains must arrange themselves into larger right-hand twisted microfibrils, and these microfibrils then cross-link into a larger unit with the help of cross-linking proteins, forming a fibril. Collagen fibrils are super twisted, highly stable, and act as one secure unit, like a “nanoscale rope” (Shoulders & Raines, 2009). The prevalence of collagen in suction cups thus allows for a pliable, highly sturdy, and energy-efficient tissue structure.
Remora fish
The Echeneid family is composed of eight different species known as remora. Many studies about suction cups have had this family of fish as their basis of investigation. The remora fish, especially the common remora, is best known for its hitchhiking lifestyle. It attaches itself to a host, usually a large marine predator such as sharks or dolphins, allowing the remora to gain access to food, transportation and protection from these predators. Some species of the Echeneid family are known to eat the parasites of the skin of predators (Leao, 2022). Two prominent features give remora their impressive adhesion capabilities: the soft lip on the outside of the suction cup, and the pectinate lamellae, as illustrated in Figure 3.

Fig. 3 Lateral view of the Remora osteochir and its suction cup (Britz, 2012).
The soft lip of the suction cup gives the remora suction cup its adhesive capabilities. When the lip encounters a surface, it goes through deformation. This deformation expulses water out of the surface. The lip then recovers its original shape, creating a zone of sub-ambient pressure. This is because water is expelled from beneath the suction cup, so when the rim naturally goes back to its original shape, a pressure differential is created. Based on this mechanism, one can see that the soft lips must be resilient to allow for deformation and to recover their original form. Furthermore, the water drag is exerted on the remora due to the movement of the host, meaning that the brim must also be resistant to external forces (Chen, 2020).
The viscoelastic property of the lips can be explained by their composition and morphology. Based on cellular morphology, orientation and tissue proportion, five different areas have been identified inside the remora's lips (Cohen, 2020). These regions are displayed in Figure 4. The outermost layer, the epidermis, is composed of stratified epithelium. Papillae can be found embedded in the surface of the epidermis. The papillae enhance adhesion by increasing the surface area of the lips. Additionally, they increase friction, an essential component of the adhesion mechanism. Each papilla is innervated by a single nerve and supported by cartilage. Cartilage is a type of flexible connective tissue made up of a highly hydrated extracellular matrix and resident cells called chondrocytes. Through chondrogenesis, these cells produce a collagen-based extracellular matrix rich in water-binding proteoglycans, meaning that cartilage can be bent and still recover its original shape (Ferng, 2022). The endings of push-rod receptor complexes can be found as well. These receptors are specialized in sensing mechanical pressure and distortion. They are essential as they allow the remora to fine-tune its adhesion when in contact with a host (Cohen, 2020). Indeed, the remora uses small tooth-like projections that are on top of the lamellae called spinules to enhance its adhesion to the host. By altering the angle between the spinules and the contact surface, friction and adhesion to the host are enhanced. Mechanoreceptors sense the pressure generated by the contact with the host, which tells the remora how to adjust the inclination of the spinules. These receptors are also supported by cartilage and are stimulated by three different nerves.

Fig. 4 a) Schematic of the five regions of the remora soft lip tissue, b) zoom-in of push-rod mechanoreceptor complex, c) schematic, and d) microscopic image of crossed-fiber collagen (Cohen et al., 2020).
Push-rod complexes have a dome shape, curving inwards and arranged in such a way that the compression of the skin does not affect nerve activity. These are also mostly positioned in the anterior (i.e. close to the head) side of the suction mechanism. This leads to the conclusion that sticking and unsticking processes are initiated from the anterior side of the suction cup. These nerves are used to regulate another element of the suction cup mechanism known as pectinated lamellae. To continue, the second layer, the dermis, is primarily composed of collagen fibers and loosely connected connective tissue. These collagen fibers are arranged lengthwise horizontally. Large channels for interstitial fluid can also be found. Although the specific mechanics between interstitial fluid and collagen fibers is unknown, the movement of fluid throughout the soft structures is essential to the viscoelastic properties of the soft lip. This relationship has led scientists to hypothesize that the lip of the remora acts as a “hydrostatically mediated viscoelastic seal” (Cohen, 2019). More anterior regions have a section of tightly packed collagen fibers in a crossed-fiber pattern. Multiple nerve fibers can also be found in this section. These nerves innervate the dermal and epidermal sensory organs of the remora.
The third region is the most complex and varied in its composition. Three forms of collagen, elastin, fat cells, and connective tissues are found distributed along the entire area. Both superior and inferior ends of this territory are composed of dense, irregularly shaped, connective tissue. Connective tissue is arranged tightly, leaving no gaps between fibers. The tissue is formed by the matrix of fibroblasts and collagen fibers. Fibroblasts are a diverse population of cells essential in the structure of tissues for organisms. Their extracellular matrix is formed by structural and non-structural substances (such as collagen and osteopontin, respectively). These cells are responsible for connective tissue stroma (supportive networks for organs). They create, maintain, and modify these connective tissue structures through the production of the extracellular matrix and proteinases (Sorell, 2009). Proteinases are a complex group of enzymes whose main role is the regulation of metabolic processes (Kalisz, n.d.). Fibroblasts constantly modify the extracellular matrix through these enzymes.
On the other hand, the mid-portion of this section consists of kinked collagen bundles oriented longitudinally. These fibers are longer than any other element in the area and connect the superior and inferior parts of the region. The kinked nature of these fibers helps the remora resist vertical forces from separating it from the host to which it is attached. When pulled, the collagen fibers straighten, giving extra resistance to upright forces. The tensile modulus is also regulated by the kinked strands. At lower magnitudes of forces, the modulus is lower than when the force is increased. This helps the material to be extremely flexible, creating a seal even when attached to an irregular surface. The kinked collagen fibers are separated by fat cells. Elastin is also abundant in this section. Elastin is a protein that has elastic capabilities, and is in part responsible for the behavior of the lips (Mithieux, 2005). This protein is formed by the assembly of tropoelastin through a process called coacervation (Wise, 2009). This process is an inverse temperature transition, where tropoelastin molecules ultimately accumulate with increasing temperature. This amalgamation of tropoelastin is necessary to facilitate the crosslinking of tropoelastin molecules. Cross-linking occurs in the extracellular space and is mediated by the action of copper-dependent amine oxidase, lysyl oxidase. At the end of this process, elastin has been formed.
Elastin is involved in giving the remora's soft lips its elastic properties. This property of elastin can be seen when analyzing its chemical composition. In fact, elastin's composition is so complex that many theories have been developed around it. At first, it was believed that elastin was a random network of chains with an entropic elastic force. In a relaxed state, the system of random chains would have a very large entropy. When stretching occurs, entropy in the system is decreased, which therefore provides the necessary force to return to a resting state. Afterwards, it was discovered that the hydrophobic groups inside elastin also contributed to the decrease in entropy. These groups, usually located inside the unstretched networks of tropoelastin chains, typically do not come into contact with water. As soon as stretching occurs, the matrix of the structure is deformed, exposing the hydrophobic groups to water. This interaction contributes to the storage of elastic energy by the elastin molecule. Later, anisotropic and fibrillar models of elastin were developed (Samouillan, 2004). These models regard the tropoelastin molecules as fibers instead of random chains. Each monomer of tropoelastin is fibrillar and has both crosslink and hydrophobic regions, each granting the molecule rigidity and flexibility. Anisotropic means that the elastin molecule would have different elastic properties depending on the direction it is measured. As evidenced by its arrangement in each section of the remora lip, its elasticity is at its maximum when measured vertically. Finally, most recent models propose that the structure of elastin is elastic due to its β-spiral structure. This β-spiral structure is created by the repetition of β-turns, which in turn are made up of repeating sequences of amino acids (e.g., VPGVG, VPGG, and APGVG) found in tropoelastin. Elastin is still considered to have an entropic elastic force, but now its composition is considered organized and structural (Mithieux, 2005).
Skeletal muscle attaches at this area in the section closer to the head of the remora in the fourth region. These skeletal muscles are responsible for controlling the movement of the pectinate lamellae. As well, this section is composed of cross-fiber collagen. The fibers are organized horizontally. Finally, the fifth region has a composition that is virtually equal to the first section discussed. Skeletal muscle is also attached to this region.
Overall, the ability of the soft lip to be stretched while maintaining its original shape is an evolutionary adaptation essential to the remora's lifestyle. Its viscoelastic nature allows it to attach itself perfectly to any given surface while resisting any external forces acting on it.
Northern clingfish
The northern clingfish inhabits intertidal environments characterized by rough rocks and strong tides. It has developed an impressive reversible suction disk capable of adhering to a wide range of irregular surfaces and resisting shear forces from strong currents (Wainwright et al., 2013). A clingfish attached to a rock substrate and the corresponding adhesive forces are shown in Figure 5.
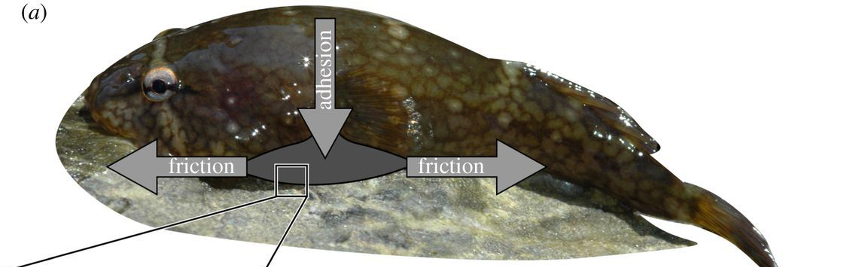
Fig. 5 Northern clingfish adhering to a rock. The suction adhesive force acts perpendicular to the substrate and friction acts parallel to the substrate (Wainwright et al., 2013).
Tissue composition
The clingfish's adhesion is primarily controlled by a system of strong, flexible connective tissues. To create and maintain the pressure differential, the material of the disc's margin first needs to be flexible to adapt to irregular surfaces and increase the volume between the suction cup and the substrate to decrease the pressure inside the cup passively. The suction disc then needs to collapse to reduce volume and restore its initial shape after a seal is created to create a pressure differential (Arita, 1976). This means that the disc's margin needs to be flexible while also passively trying to revert to its original shape. These structural properties are possible because of collagen-rich connective tissue.
Under the layer of papillae is a layer of dense connective tissue with some regions of loose connective tissue. Supporting it, there is another region of dense connective tissue with layers of collagen. Underneath that region lies muscle tissue and the connective tissue that supports the disc's skeleton. These layers of connective tissue, which can be seen in Figure 6, allow for the suction cup's margin to be flexible while still giving stress resistance and strength (Green & Barber, 1988).
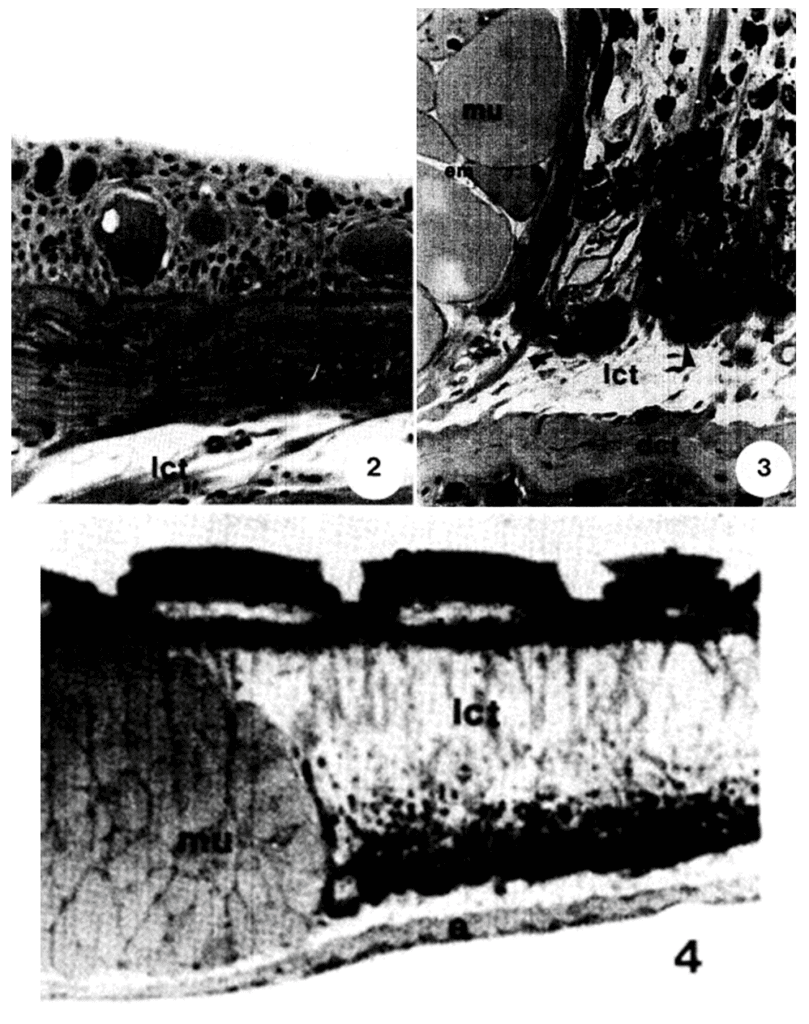
Fig. 6 Vertical skin section of clingfish from another region than the suction disc with cuboidal epithelium as seen in light microscopy. We can see a layer of loose connective tissue (l cm) under the epidermis. 3: The region under the borders of the suction disc. It shows a layer of dense connective tissue (dct) under loose connective tissue (lct) separated from the muscle cells (mu) by strands of collagen. 4: Lower-power micrograph of the suction disc. The image shows a loose connective tissue layer with strands of vertically oriented collagen. We can also see that there is a muscle bundle (mu) under the papillae (Adapted from Green & Barber, 1987).
Furthermore, the skeleton provides rigidity to the top of the disc to prevent its edges from slipping inward and also generates the pressure difference. Additionally, clingfish have fin rays attached by ligaments. However, they are not fully ossified, they are made of multiple joints as can be seen in Figure 7C rather than a single ossified piece such as in the case of Eumicrotremus orbis (Figure 7). In the case of Gobiesox, they allow the disc to collapse through muscle action while forcing it back to a cup shape once muscle tension is released (Arita, 1976).
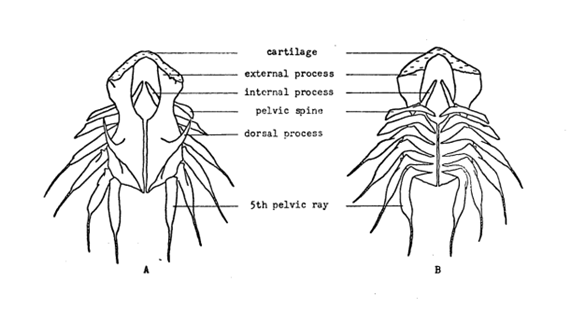

Fig. 7 A, B: Bones in the suction disc of Eumicrotremus orbis, where A is the front view and B is the ventral view. C: Bones in the suction disc of Gobiesox meandricus. We can see that the fins are made of multiple joints for Gobiesox unlike the ossified bones of Eumicrotremus orbis (Adapted from Arita, 1976).
In addition to the creation of a pressure differential, the two main mechanisms required for adhesion enhancement are the secretion of mucus for adhesion on rough surfaces, and the generation of friction between the cup and the substrate by microstructures and the cuticle. These functions are achieved by four main cell types present in the suction disc: acidophil cells, mucous cells, epithelial cells, and “alarm substance” cells (Green & Barber, 1988). Amongst these cells, the epithelial and the mucous cells are the main contributors to adhesion. As discussed later in this section, the epithelial cells and their hierarchy of microstructures that generate friction make up the papillae. The columnar epithelium cells at the surface are also responsible for secreting the cuticle and holding it in place with their microvilli. Finally, mucous cells are responsible for the mucus that helps the disc stick through a specific type of Stefan adhesion.
Stefan adhesion describes the perpendicular resistive force between two plates as they are separated in the presence of a fluid. In the traditional definition of Stefan adhesion, several assumptions are made, including the Newtonian nature of the fluid. In the presence of a non-Newtonian fluid, meaning that the viscosity changes with the magnitude of applied force, this adhesive force is termed the “viscous flow” adhesive force. The Stefan adhesive force equation is modified to account for the non-Newtonian fluid, resulting in Equation 1. R4 represents sucker pad size, m describes the viscosity of the fluid in the cavity, 1D3t is gap size, and dDtdt is the speed at which the surfaces move away from each other (Chan & Carlson, 2019).

This force is additive to the force created by the pressure differential across the suction cup, as illustrated in Figure 8.

Fig. 8 Viscous flow (Tsukagoshi & Osada, 2021).
Clingfish mucus
In other marine organisms, notably mussels, a glue-like mucus secreted by the organism allows for powerful adhesion generation primarily dependent on the presence of adhesive proteins (Waite & Qin, 2001). Clingfish secrete a viscous mucus, similar to that of mussels in its glycoprotein composition, that has been hypothesized to enhance the clingfish's pressure-based suctioning mechanism.
A team of scientists set out to determine the function of the clingfish mucus in suction (Wainwright et al., 2013). Using a tensile testing machine, base-level measurements for clingfish adhesion were obtained by measuring the force generated by dead clingfish on various surfaces in the presence of seawater. It is important to note that previous studies have shown that 96% of suction capability is retained by freshly euthanized clingfish, so clingfish cadavers were appropriate models for force testing (Arita, 1967). A tensile testing machine pulls opposite the surface of adhesion at a set speed, in this case 1 m/min, reading out the force applied by the machine. The force reading on the machine at the moment that the cup breaks suction is equal to the suction force generated by the sucker. Traditional suction is area-dependent, as represented by Equation 1, where p is atmospheric pressure and A is area of the suction pad:

The group adjusted their force measurements to account for differences in pad size by using the peak tensile stress, where K is the tensile stress and F' is the force measured by the force sensor:

The adhesion of manufactured suction cups was then tested using a similar procedure on a variety of surfaces with different levels of irregularity. As expected, manufactured suction cups performed 80% better than the clingfish on smooth surfaces, but their adhesion broke down on any surface with surface crevices, also termed “gap size,” wider than 21.8 µm. In comparison, although performing worse on smooth surfaces, clingfish were able to adhere to surfaces with gap sizes up to 269.0 µm, more than twelve times the grit size manufactured suction cups can withstand.
Manufactured suction cups were tested using viscous liquids to test the hypothesis that mucus allows for better adhesion on irregular surfaces. Methylcellulose, 20 times more viscous than water, saw no improvement compared to seawater, still unable to allow adhesion to surfaces with grit size larger than 21.8 µm. Using glycerine, 1400 times more viscous than water, adhesion on surfaces with up to 78.0 µm grit size was achieved.
The viscosity of a fluid is well known to increase suction due to increased Stefan adhesion. Although the use of a viscous fluid improves adhesion, glycerine is 70 times more viscous than the clingfish mucus, and the manufactured suction cups still underperformed in comparison to clingfish suction cups (Wainwright et al., 2013). The authors of the study concluded that another factor must be at play that contributes to the adhesion capabilities of the clingfish.
It was initially hypothesized that the clingfish might be taking advantage of a system of adhesive proteins producing high intermolecular force, or van der Waals attraction, between the substrate and the mucus. This technique is employed by geckos, arthropods, mussels, and barnacles (Wainwright et al., 2013). In a histochemical study of the mucus secretion of the Lepadogastor, it was discovered that the sacciform cells' secretion contained high concentrations of –SH radials, tryptophan, and serotonin (Zaccone, 1981). The authors of the study hypothesized that these components served primarily as irritants for predators or as regulators of water levels in the epidermis. The authors also confirmed the glycoprotein nature of the mucus and the cuticle through histochemical reactivity tests for proteins and polysaccharides. Glycoproteins at the surface of the epithelial cells of the clingfish were already well-known to promote cell aggregation. The study's author found that the glycoproteins were also likely involved in increasing adhesive strength between the suction disk and the substrate. They even went as far as to assert that the suction cup's cuticle was likely more contributory to adhesive strength than protection from physical damage (Zaccone, 1981).
In summary, both the high glycoprotein content and viscous nature of the clingfish mucus do indeed improve adhesion. The study by Wainwright et al. explores another interesting feature of the clingfish suction cup, the complex system of microfilaments present at the edges of their suction cups.
Microfilament composition
The edges of the clingfish suction cups are covered in a hierarchical array of microfilaments that prevent the disc's margin from slipping, which would collapse the cup and create leaking. Clusters of epithelial cells have microvilli theorized to generate friction and allow the clingfish to adapt to rougher surfaces (Wainwright et al., 2013). Where traditionally manufactured suction cups would collapse inwards, the microvilli contribute to friction so the fish can grip onto a surface and prevent the cup's edges from sliding. The chemical structure of these microfilaments is critical to their ability to prevent leakage and improve adhesion. Microvilli are 2 µm protuberances on the surfaces of cells, and thousands of microvilli make up a “brush border” on the surface of specific types of epithelial cells. These structures are made up of actin, which forms actin filaments when polymerized (Pollard & Mooseker, 1981). The organization of the microvilli is shown in Figure 9.
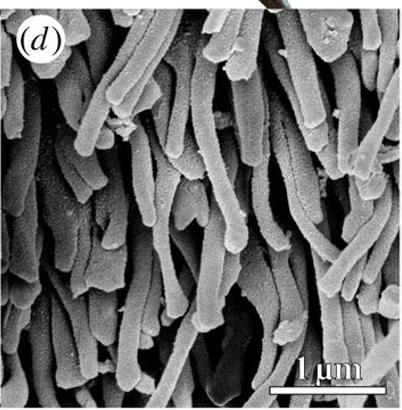
Fig. 9 Scanning electron microscopy (SEM) image of the organization of the microvilli (Wainwright et al., 2013).
Actin polymerization
Actin polymerization involves several major steps: nucleation, elongation, and breaking-annealing. The actin polymerization process is well characterized quantitatively in bacterial flagellum and nonpolar polymers, where the elongation of the actin chain is unidirectional. The change in length of a polymer is defined by Equation 3, where Kfwd is the rate at which new monomers are added to a polymer chain and Krev is the rate at which monomers are lost from the chain.
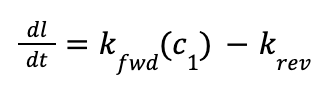
Equilibrium is reached when kfwd(c1) = krev which suggests an equilibrium constant of K=kfwdkrev=1c0 , where c0 is the critical concentration of monomers for polymer elongation. When the concentration of monomers exceeds this value, meaning that the reaction quotient is less than the equilibrium constant, the reaction will shift towards the product, forming a longer polymer chain (Pollard & Mooseker, 1981).
However, in the formation of microtubules and microvilli, the polarized nature allows for the extension of the polymer from both ends at differing rates. In a study by Pollard & Mooseker, 1981, transmission electron microscopy (TEM) was used to visualize actin polymerization in microvilli, and the growth rates at each end of the filaments were quantified to determine rate constants for elongation. As shown in Figure 10 below, the “barbed” end (top left) of the stained microvilli electron micrograph grows significantly faster than the “pointed” end (bottom right).
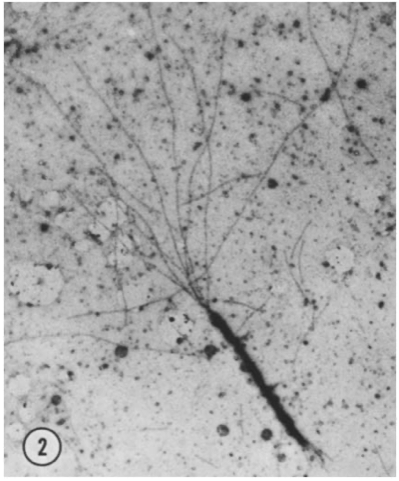
Fig. 10 Electron micrograph of dual-ended actin polymerization. The barbed end (top left) elongates faster than the pointed end (bottom right) (Pollard & Mooseker, 1981).
In order to determine the rate constants of elongation for each end, the length of each filament was measured from the site of nucleation, and the mean length of the filament was plotted against time. Least squares regression was performed to determine the rate constant for both the barbed and pointed ends, as shown in Figure 11.

Fig. 11 Plot of least squares regression for the length of the actin polymer (µm) as a function of time (s). Circles represent the fast-growing barbed end; triangles represent the slow-growing pointed end (Pollard & Mooseker, 1981).
As shown in the plots of polymer elongation, the rate of elongation follows the same linear form already characterized for flagellum construction. It was determined that the rate of elongation of the barbed end was approximately six times faster than that of the slow end. The differing rates of elongation at each end also suggest a phenomenon now known as “actin treadmilling,” in which one end of the polymer is growing while the other end of the polymer is shortening, leading to a net “steady-state flux” through the entire polymer. This steady state occurs when the rate of elongation at the barbed end is equal to the rate of shortening at the pointed end, or when dlbarbeddt= -dlpointeddt.
Actin cross-linking
These filaments are crosslinked by one of twenty-three actin cross-linking proteins. Crosslinking of actin filaments allows collections of individual filaments to act together to control motile and adhesive properties. Actin filaments are viscoelastic, meaning they can resist movement like a viscous fluid and store energy when compressed (Tseng et al., 2005).
In a study by Tseng et al., 2005, microinjections of two actin cross-linking proteins, ɑ-actinin and fascin were shown to provide increased mechanical strength, as measured by reduced local deformation—or “compliance”—of the cell in response to a small force. As shown in Figure 12A, the actin in cells injected with only ɑ-actinin cross-linked into a dense network of orthogonal filaments, almost like a spider web. In Figure 12A', the actin in cells injected with fascin cross-link into dense, straight, spear-like bundles. Cells injected with both cross-linking proteins (Figure 12A'') displayed actin cross-linking characteristic of both ɑ-actinin and fascin, developing a complex network of spear-like bundles intertwined with a fine orthogonal mesh.
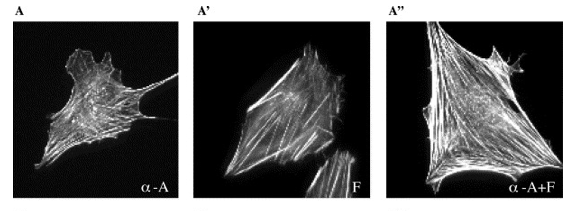
Fig. 12 Fluorescence micrographs of cross-linked actin structure for cells injected with (A) ɑ-actinin, (A') fascin, and (A'') ɑ-actinin and fascin (Pollard & Mooseker, 1981).
As seen in Figure 13, cells containing both ɑ-actinin and fascin in comparison to both the control and cells containing only one cross-linker, combinations of cross-linking proteins indeed increase mechanical strength.
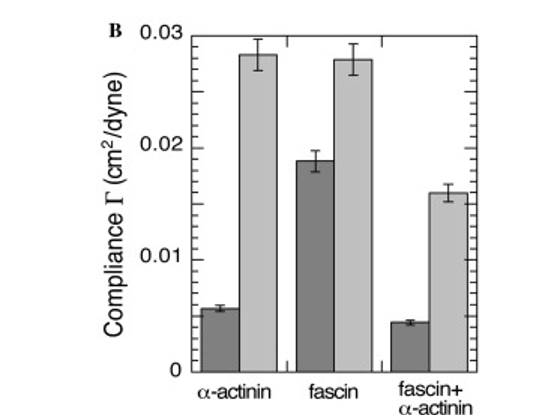
Fig. 13 Compliance of cells injected with ɑ-actinin, fascin, and ɑ-actinin and fascin (Pollard & Mooseker, 1981).
In the case of the clingfish microvilli, this increased strength allows the clingfish to better resist forces caused by strong currents. Amazingly, the cross-linking also increased elasticity, as shown in Figure 14.
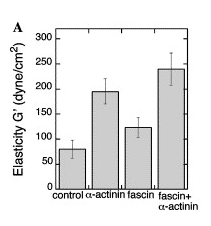
Fig. 14 Elasticity of cells injected with ɑ-actinin, fascin, and ɑ-actinin and fascin (Pollard & Mooseker, 1981).
Based on this study, crosslinking of actin filaments has proven to increase their viscoelastic character. This enhancement of viscoelastic behavior allows the microvilli to both provide support due to increased mechanical strength while simultaneously adapting elastically to uneven surfaces to improve the strength of the seal.
Suckermouth catfish
Suckermouth catfish are small freshwater fishes that have adapted an oral suction cup. Species like Mochokidae can be found in Africa, while Astroblepus and Loricariidae can be found in South America. This diversity in habitats means that studying different suckermouth catfishes can help understand the role of tissue composition by seeing how it changes in similar species that have specific needs. The rivers and streams they inhabit are advantageous because there are fewer predators. However, this means they must be able to resist the low to high currents in the water. While clingfish and remoras evolved suction discs from their fins, the suckermouth catfish have evolved oral suction cups that vary depending on their specific needs. For instance, neotropical suckermouth catfish like the Loricariidae rely less on their suction cups because their environment is rich in surfaces with loose particles (Bressman et al., 2020). On the other end of the spectrum, the Astroblepus can use suction to climb waterfalls (Bressman et al., 2020). Because they are oral suction cups, their mouths must also allow for respiration and feeding on top of allowing adhesion to a surface. For these functions, they have also evolved sensory cells that work with epithelial cells both for feeding and aiding in the suction mechanism.
To have a flexible and well-supported suction cup, a suckermouth catfish's lips are fleshy because of the large collagen content. Bressman et al. (2020) used Verhoeff-Van Gieson stain, a staining technique used to determine the presence of collagen, to visually measure the disc's areas with high concentrations of collagen. This allowed them to compare the collagen content and repartition of different catfish. Their results show that collagen is the primary component of the tissue in the lips of Chaetostoma. They also observed that collagen content and the parts where it was most prominent varied between species. They also have “elastin and/or elastin-like proteins” to give flexibility and support (Bressman et al., 2020). Because their suction cups are also their mouths, the surface of the suction cups is covered in sensory cells that help them find food. In certain species, the suction cup's margin is also covered in papillae that help them adhere under higher currents (Ono, 1980). To improve adhesion, the papillae (Figure 15) have epidermal brushes ranging from 0.9 to 5.5 µm in height from hexagonal cells. Besides helping with adhesion, the brushes also help scrape food off surfaces.

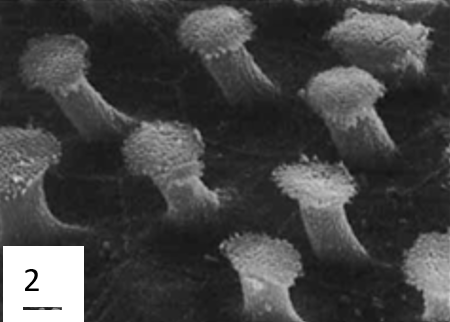
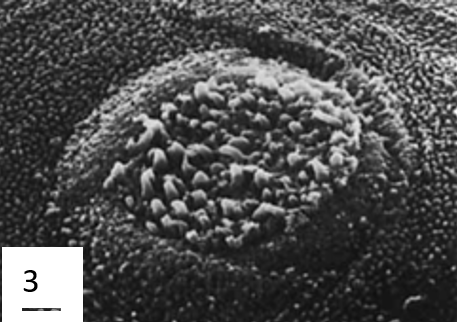
Fig. 15 Microstructures present on the surface of Ancistrius sp. 1: Oral papilla with epidermal brush field (EB) and taste buds (TB). 2: Detail of epidermal brushes. 3: Detail of a taste bud (Ono, 1980).
To determine the function of collagen in the catfish's suction cup, Bressman et al. (2020) hypothesized that there would be a relationship between the amount of collagen, the typical amount of current a catfish is exposed to, and the substrate type. Their results for drag were not significant, but they did get significant results for substrate type. They speculated that the non-significant results for drag were partly due to the small sample size. On the other hand, they suggested that drag could correlate with substrate type, since the high currents in their environment could hinder the accumulation of sand and wood that would make up a soft substrate. The idea that soft substrates are associated with less collagen could be explained by the fact that loose particles make adherence difficult. Even though the results were not significant, they still found that Astroblepus and Chaetostoma, the two species living in the habitats with the most current, had the most collagenous lips. This could be explained by the fact that collagen is semi-rigid, potentially preventing the margin from slipping inward and buckling.
Octopuses
Cephalopods are a family of animals in which the common octopus is included. Its life cycle ranges from approximately 12-20 months and is broken up into five phases: embryonic, paralarva, juvenile, subadult, and adult (Jereb et al., 2015). In order to help identify diseases that invade a species, the skin is analyzed and “the characteristics that must be observed in the skin are the presence of peeling, bruising, ulcers, and mucus hypersecretion” (Gonzales et al., 2020).
Nerve sensing
Each arm of the octopus contains a central axial nerve cord connected to clusters of nerve cell bodies, called ganglia, at each sucker. The ganglia allow each sucker to behave autonomously which means that there is no intervention from the central nervous system of the octopus. This enhances the speed at which the suckers react to external stimuli, as responses can be made without central nervous system processing. The chemical receptors of the suckers detect hydrophobic compounds in water whenever they encounter them. These receptors are called chemotactile receptors (CRs) and are said to mediate “touch-taste arm behavior” (van Giesen, 2020). These chemotactile receptors diverge from the common neurotransmitter receptors; they are specific to cephalopods' and were shown to react when coming in contact with fish and crab extract. More specifically, the CRs were activated when meeting specific terpenoids produced by mollusks and cnidarians. Terpenoids encompass a large range of hydrocarbon compounds, consisting of a terpene attached to an oxygen-containing group. Terpenes are aromatic groups that can be found in plants and are usually responsible for their characteristic aroma (Juthi, 2019). These substances tend to have unsaturated molecules composed of linked isoprene, whose molecular structure can be seen in Figure 16.

Fig. 16 Molecular structure of Isoprene (Pubchem, 2020).
Interestingly, not all chemotactile receptors are equal. Some may react to specific compounds, while others may remain inactive. The responsive and unresponsive nerves form sub-units, which allow for fine-tuning of the sucker movement. The response of active CRs is affected when in proximity to an inactive CR. Collectively these units allow for signal processing and coding, which give the arms their autonomy. Research has identified four different types of receptor cells that innervate the sucker: type 2a and type 2b, which are the most abundant, are CRs. On the other hand, the specific function of type 4 and type 3 cells (the notation skips type 1) have not yet been identified. It is theorized that type 4 cells are responsible for the detection of delicate pressure. These would classify them as mechanoreceptors, but this also remains uncertain. Type 3 cells are theorized to be responsible for either stretch and deformation sensing, or seal formation. These cells would tell the sucker whether a tight seal has been formed around the object in contact (Graziadei, 1976).
Another protein involved in sensing is Ov-GRK1. Expression of this protein is concentrated in the retina of the cephalopods but can also be found in the epithelium rim of the suckers. The Ov-GRK1 is a protein coded by the O. vulgaris GRK1 gene with light-sensing properties. The presence of these molecules would allow the octopus sucker to detect the presence of possible prey in places without direct visual access to the cephalopod. One can say that the octopus possesses extraocular vision. Further proof of this theory can be seen by the presence of rhodopsin, another sensory protein that changes light into electrical signaling (Al-Soudy, 2021).
Mucus composition
The skin has a multitude of purposes in addition to protecting the body. These include lubrication, osmotic and ionic management, exchange of nutrients and oxygen, intraspecific communication, camouflage, and even attracting prey (Accogli et al., 2017). Most organisms and their skin contain mucous epithelium (a barrier that separates tissue from their environment), so most up-to-date aquatic skin research narrows in on secretory characteristics. There are two layers in the skin of cephalopods: epidermis and dermis, as seen in Figure 17. Not only do these layers cover the entire external surface of the octopus (including its suckers), but they also run along a couple of the internal body components.
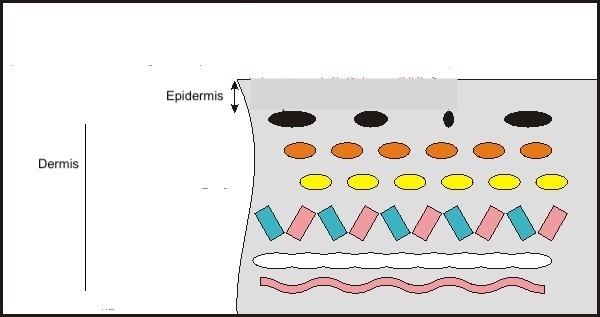
Fig. 17 Octopus skin is composed of two layers: epidermis and dermis (Stanley, 2015).
A few of the roles are influenced by the make-up and characteristics of the glycoconjugates, which express the secretory cells of the epidermis that create the mucus layer (Gonzales et al., 2020). Glycoproteins and proteoglycans make up the majority of mucus ingredients, with lesser amounts of lysozymes, immunoglobulins, and other proteolytic enzymes. The chemical makeup of the mucus in the genus Octopus is more restricted than that of other molluscs (species with soft unsegmented bodies with, usually, an external calcareous shell) (Smith, 2002). The theory developed was that the cellular structure and chemical composition of the mucus changes over the course of the Octopus vulgaris life cycle. The epidermal structure of fish has been shown to be influenced by a variety of other factors, including their environment, size, sex, level of maturation, and malnutrition. Understanding the makeup of mucus may help explain how it functions in various bodily regions. In cephalopods, mucus properties also serve as a quality indicator of their health. The mantle, arm, and other sections of the sucker have all been examined to gain a deeper knowledge of the morphological characteristics of the epidermis and the composition of glycoconjugates. These sections of study can be observed in Figure 18 (Gonzales et al., 2020).
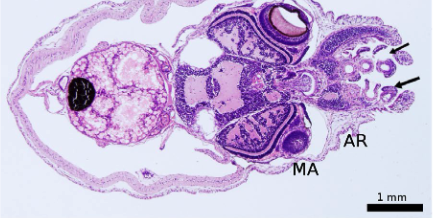
Fig. 18 This figure shows the three areas of study: the mantle, arm, and suckers (indicated by arrows) (Gonzales et al., 2020).
The mucus layer that covers the skin of the majority of marine species is dense with glycoconjugates and symbolizes how an individual interacts with their environment. Adults and paralarvae have a simple cylindrical and pseudostratified epithelia covering their mantle and arm. The epithelia are made up of epithelial cells and two types of secretory cells known as mucocytes 1 and 2, shown in Figure 19 (in nomenclature: m1 and m2). In the mantle and the arm, epithelial cells along with neutral glycoconjugates, loaded with mannose and glucose (ConA+) are found (Álvarez Nogal & Molist García, 2015). It is uncertain how lectin Con A binds to the O-glycans of the glycoproteins in animal cells, though it does bind to several kinds of N-glycans. This result, therefore, implies that the n-glycoproteins produced by the epithelial cells of the mantle and arm of Octopus vulgaris would contribute to the creation of an extracellular surface layer, which would be implicated in enhancing of the lubrication on the body's surface (Cummings & Etzler, 2009).

Fig. 19 Epithelium formed by epithelial cells and two types of mucocytes (m1 and m2) (Gonzales et al., 2020).
There also has been evidence that sialic acids—monosaccharide units that link together to create the glycoconjugate (produced by the secretory m1 and m2 cells)—are the WGA's (Tricium vulgare agglutinin) preferred binding sites. The release of acid glycoconjugates on the epidermis of aquatic animals has been linked to an increase in mucus viscosity, which is essential for trapping bacteria and other pathogens and protecting the body against mechanical harm. Therefore, during the life cycle of Octopus vulgaris, both types of cells contribute to making the mucus more viscous in the mantle and arms (Accogli et al., 2017).
Both segments of the sucker have different cuticle and apical glucidic compositions. An acid mucin cuticle made of N-acetylglucosamine and N-acetylgalactosamine (derivatives of glucose) covers the surface of the infundibulum. The acetabulum's cuticle expresses mannosylated neutral glycans rather than the acid glycan seen in the infundibulum (Gonzales et al., 2020). Since they perform different functions in the adhesion process, the difference in the makeup of the two chambers is presumably a result of their different functions. The infundibulum makes contact with the substrate, while the acetabulum is responsible for creating the pressure to form a seal. In gastropods, adhesion mucus has more acid glycans than mucus on surfaces that promote mobility. One could conclude that the acetabulum region would not be as crucial to the adhesive process of the sucker as the acid glycans of the infundibular cuticle in the octopus.
In simple terms, the epithelial cells of the mantle and arm's epidermis appear to have an apical surface covered in N-glycoproteins, which may help to keep the body lubricated and facilitate mobility. However, proteoglycans, which make mucus more viscous and are crucial to the body's defence against external threats, are the fundamental characteristic of secretory cells.
Conclusion
Nature, as an engineer, searches for the path of least resistance, creating the most energy-efficient mechanism to perform a specific function. Each suctioning mechanism is chemically fine-tuned to enhance adhesive properties and flexibility while minimizing energy output, allowing each organism to benefit most from its adhesive mechanism at the lowest energetic cost. The characteristics of the remora soft lip reveal the importance of its hierarchical organization, and high concentration of collagen and other supporting proteins. This can also be seen in the clingfish and catfish adhesive mechanisms. The importance of supporting structures, such as papillae and microcapillaries can be highlighted in the clingfish and suckermouth catfish. The viscous mucus produced by clingfish and octopuses is a major evolutionary advantage that enhances adhesion for these species without excessive additional energy input. Activation of chemical receptors when octopus suckers detect terpenoids specific to their prey allows fast, efficient predation without activating the central nervous system. In conclusion, the chemical composition, structure and organization of the different elements involved in suction cups are expertly constructed to allow for energy-efficient powerful adhesion without compromising elastic adaptability on irregular surfaces.
References
- Accogli, G., Scillitani, G., Mentino, D., & Desantis, S. (2017). Characterization of the skin mucus in the common octopus Octopus vulgaris (Cuvier) reared paralarvae. European Journal of Histochemistry, 61, 204–214. https://doi.org/10.4081/ejh.2017.2815
- Álvarez Nogal, R., & Molist García, P. (2015). The outer mantle epithelium of Haliotis tuberculata (Gastropoda Haliotidae): An ultrastructural and histochemical study using lectins. Acta Zoologica, 96, 452–459.
- Accogli, G., Scillitani, G., Mentino, D., & Desantis, S. (2017). Characterization of the skin mucus in the common octopus Octopus vulgaris (Cuvier) reared paralarvae. European Journal of Histochemistry, 61, 204–214. https://doi.org/10.4081/ejh.2017.2815
- Al-Soudy, A.-S., Maselli, V., Galdiero, S., Kuba, M. J., Polese, G., & Di Cosmo, A.. (2021). Identification and Characterization of a Rhodopsin Kinase Gene in the Suckers of Octopus vulgaris: Looking around Using Arms?. Biology, 10(9), 936. https://doi.org/10.3390/biology10090936
- Arita, G. S. (1967). A comparative study of the structure and function of the adhesive apparatus of the Cyclopteridae and Gobiesocidae (T). University of British Columbia. Retrieved from https://open.library.ubc.ca/collections/ubctheses/831/items/1.0104391
- Bressman, N. R., Armbruster, J. W., Lujan, N. K., Udoh, I., & Ashley‐Ross, M. A.. (2020). Evolutionary optimization of an anatomical suction cup: Lip collagen content and its correlation with flow and substrate in Neotropical suckermouth catfishes (Loricarioidei). Journal of Morphology, 281(6), 676–687. http://doi.org/10.1002/jmor.21136
- Chen, Y., Meng, J., Gu, Z., Wan, X., Jiang, L. et Wang, S.. (2020). Bioinspired Multiscale Wet Adhesive Surfaces: Structures and Controlled Adhesion. Advanced functional materials, 30(5), 1905287. http://doi.org/10.1002/adfm.201905287
- Cohen, K. E., Crawford, C. H., Hernandez, L. P., Beckert, M., Nadler, J. H., & Flammang, B. E.. (2020). Sucker with a fat lip: The soft tissues underlying the viscoelastic grip of remora adhesion. Journal of Anatomy, 237(4), 643–654. https://doi.org/10.1111/joa.13227
- Cohen, K. E., Flammang, B. E., Crawford, C. H., & Hernandez, L. P.. (2020). Knowing when to stick: touch receptors found in the remora adhesive disc. Royal Society Open Science, 7(1), 190990. https://doi.org/10.1098/rsos.190990
- Cummings, R. D., & Etzler, M. E. (2009). Antibodies and lectins in glycan analysis. In A. Varki, R. D. Cummings, & J. D. Esko (Eds.), Essentials of glycobiology (pp. 633–647). New York, NY: Cold Spring Harbor Laboratory Press.
- Dana Ono, R. (1980), Fine structure and distribution of epidermal projections associated with taste buds on the oral papillae in some loricariid catfishes (Siluroidei: Loricariidae). Journal of Morphology, 164(2), 139-159. https://doi-org.proxy3.library.mcgill.ca/10.1002/jmor.1051640204
- Ferng, A. (2022, July 8). Cartilage. Kenhub. https://www.kenhub.com/en/library/anatomy/cartilage
- González-Costa A, Fernández-Gago R, Carid S, Molist P. Mucus characterisation in the Octopus vulgaris skin throughout its life cycle. Anat Histol Embryol. 2020;49:502–510. https://doi.org/10.1111/ahe.12554
- Graziadei, P. P. C., & Gagne, H. T.. (1976). Sensory innervation in the rim of the octopus sucker. Journal of Morphology, 150(3), 639–679. https://doi.org/10.1002/jmor.1051500304
- Green, D. M., & Barber, D. L. (1988). The ventral adhesive disc of the clingfish Gobiesox maeandricus: integumental structure and adhesive mechanisms. Canadian Journal of Zoology, 66(7), 1610–1619. https://doi.org/10.1139/z88-235
- Jereb, P., Allcock, L. A., Lefkaditou, E., Piatkowski, U., Hastie, L. C., & Pierce, G. J. (2015). Cephalopod biology and fisheries in Europe: II. Species Accounts (pp. 360). ICES. https://doi.org/10.13140/RG.2.1.1081.6164
- Juthi, T. Z. (2019, August 4). Terpenoids: A Structurally Diverse Class of Natural Products. Plantlet. https://plantlet.org/terpenoids-a-structurally-diverse-class-of-natural-products/
- Kalisz, H. M.. (n.d.). Microbial proteinases (pp. 1–65). https://doi.org/10.1007/bfb0047944
- Leao, M. (2002). Remora remora Brown sucker. Animal Diversity Web. https://animaldiversity.org/accounts/Remora_remora/
- Mithieux, S. M., & Weiss, A. S. (2005). Elastin. In Advances in Protein Chemistry (Vol. 70, pp. 437-461). Academic Press. https://doi.org/https://doi.org/10.1016/S0065-3233(05)70013-9
- Pollard, T. D., & Mooseker, M. S. (1981). Direct measurement of actin polymerization rate constants by electron microscopy of actin filaments nucleated by isolated microvillus cores. The Journal of cell biology, 88(3), 654-659. https://doi.org/10.1083/jcb.88.3.654
- PubChem. (2020). Isoprene. @Pubchem; PubChem. https://pubchem.ncbi.nlm.nih.gov/compound/Isoprene
- Samouillan, V., André,, C., Dandurand, J., & Lacabanne, C.. (2004). Effect of Water on the Molecular Mobility of Elastin. Biomacromolecules, 5(3), 958–964. https://doi.org/10.1021/bm034436t
- Shoulders, M. D., & Raines, R. T. (2009). Collagen structure and stability. Annual review of biochemistry, 78, 929. 10.1146/annurev.biochem.77.032207.120833
- Smith, A. M. (2002). The structure and function of adhesive gels from invertebrates. Integrative and Comparative Biology, 42(6), 1164–1171. https://doi.org/10.1093/icb/42.6.1164
- Sorrell, J. M., & Caplan, A. I. (2009). Chapter 4 Fibroblasts—A Diverse Population at the Center of It All. In International Review of Cell and Molecular Biology (Vol. 276, pp. 161-214). Academic Press. https://doi.org/https://doi.org/10.1016/S1937-6448(09)76004-6
- Stanley, A. (2015). Light Sensitive Proteins Enable Octopuses To “See” With Their Skin. (n.d.). DOGOnews. Retrieved November 4, 2022, from https://www.dogonews.com/2015/9/6/light-sensitive-proteins-enable-octopuses-to-see-with-their-skin
- Terpenes. (n.d.). VEDANTU. https://www.vedantu.com/chemistry/terpenes
- Tseng, Y., Kole, T. P., Lee, J. S., Fedorov, E., Almo, S. C., Schafer, B. W., & Wirtz, D. (2005). How actin crosslinking and bundling proteins cooperate to generate an enhanced cell mechanical response. Biochemical and biophysical research communications, 334(1), 183-192. https://doi.org/10.1016/j.bbrc.2005.05.205
- van Giesen, L., Kilian, P. B., Allard, C. A. H., & Bellono, N. W. (2020). Molecular Basis of Chemotactile Sensation in Octopus. Cell, 183(3), 594-604.e514. https://doi.org/https://doi.org/10.1016/j.cell.2020.09.008
- Wainwright, D. K., Kleinteich, T., Kleinteich, A., Gorb, S. N., & Summers, A. P. (2013). Stick tight: suction adhesion on irregular surfaces in the northern clingfish. Biology letters, 9(3), 20130234. https://doi.org/10.1098/rsbl.2013.0234
- Waite, J. H., & Qin, X. (2001). Polyphosphoprotein from the adhesive pads of Mytilus edulis. Biochemistry, 40(9), 2887-2893. https://doi.org/10.1021/bi002718x
- Wise, S. G., & Weiss, A. S. (2009). Tropoelastin. The International Journal of Biochemistry & Cell Biology, 41(3), 494-497. https://doi.org/https://doi.org/10.1016/j.biocel.2008.03.017
- Álvarez Nogal, R., & Molist García, P. (2015). The outer mantle epithelium of Haliotis tuberculata (Gastropoda Haliotidae): An ultrastructural and histochemical study using lectins. Acta Zoologica, 96, 452–459. https://doi.org/10.1111/azo.12090
Zaccone, G. (1981). Histochemical Studies of Glycoproteins and Activities of Some Enzymes in the Skin and Sucking Disk of the Connemara Clingfish Lepadogaster Candollei Risso 1810. Institute of Zoology and Comparative Anatomy, Messina-University. 92, 317-342.