Speed, Sense, and Strike: The Biochemistry of Tentacles and their Chemical Interactions with the Environment
Yousif Al Shajlawi, Henry Bain, Charles Barakett, Julianna Bede
Abstract
The tentacle has long been a curiosity to scientists for its unique and diverse properties and functions, perhaps since antiquity when Aristotle observed the ability of cephalopods to regenerate lost tentacles and arms. Today, tentacles continue to inspire scientific innovation, such as a biomimetic recognition strategy for capturing and releasing tumor cells based on the adhesion domains of jellyfish tentacles used to effectively capture moving food (Zheng, et al., 2021). This paper will therefore explore the unique biochemical properties of the tentacle in organisms of the class Cephalopoda, Gastropoda, and Cnidaria, as well as the chemical interactions of this appendage with the environment. This paper will begin by examining the specialization of muscle tissues in cephalopod tentacles which facilitate rapid movements and the chemical processes which lead to their adhesive, regenerative, and sensory abilities. Furthermore, chemosensation in gastropod tentacles will be analyzed, with a particular focus on snails. Finally, the nervous system of the jellyfish tentacle and its specialization for prey capture via its stinging mechanism will be discussed.
Introduction
Tentacles serve various functions across different classes of species. In cephalopods, for example, tentacles provide adhesion, allowing them to easily hold their prey or cling to their partner during mating. In addition, cephalopods can regenerate their arms and tentacles through multiple steps of wound healing, tissue degeneration, and renewal. Cephalopod tentacles, also very useful in predation, contain chemosensors that can detect chemicals in the environment, which they can use to find their prey. When they find their prey, cephalopods are able to capture their prey through rapid movements of their tentacles, which is possible because of the muscle tissue specialization in the tentacles. Like cephalopods, gastropods' tentacles provide chemosensation, used to navigate the environment. It can help them find the best path to get to food or to mate, but it can also help them avoid areas where predators may be present. In cnidarians, tentacles are lined up with specialized cells called nematocysts, which can be used to paralyze prey or fend off predators by discharging sodium channels targeting toxins. These cnidarians have one of the simplest nervous systems, yet it is adequate and provides them with the necessary functions to locomote, predate, and survive.
Cephalopods
Cephalopod tentacles, such as those of the squid and cuttlefish, are particularly fascinating appendages due to their optimization for the aquatic environment. It was found that the tentacle of the squid Doryteuthis pealeii undergoes an 80% extension within 20-40 ms during prey strike, with a maximum velocity of 2 m/s and a peak acceleration of 250 m/s2 (Gilly et al., 2020). They have also developed unique characteristics, such as adhesive and regenerative abilities. These characteristics can not only be attributed to the mechanical function of the tentacle, but also its specialized biochemical properties.
Muscle tissue specialization
The musculature of the cephalopod tentacle consists of an optimal arrangement of various muscle fibers so as to achieve rapid and efficient movements during predation (Kier, 2016). Indeed, it consists of an axial nerve cord running longitudinally down the center of the tentacle stalk, surrounded by an extensive mass of transverse and radial muscle that extends across the diameter of the appendage. These muscle fibers also perpendicularly intersect the longitudinal muscle bundles running parallel to the longitudinal axis. The transverse muscle bundles near the external surface of the stalk turn and become part of the surrounding thin circular muscle layer. Layers of connective tissue surround the axial nerve cord and divide the various muscle groups. Surrounding the tentacular stalk, there is a layer of loose dermal connective tissue that contains blood vessels, nerves, and chromatophores, the latter of which being the specialized organs that contain pigment sacs responsible for color change in cephalopods. This morphology is illustrated in Figure 1.
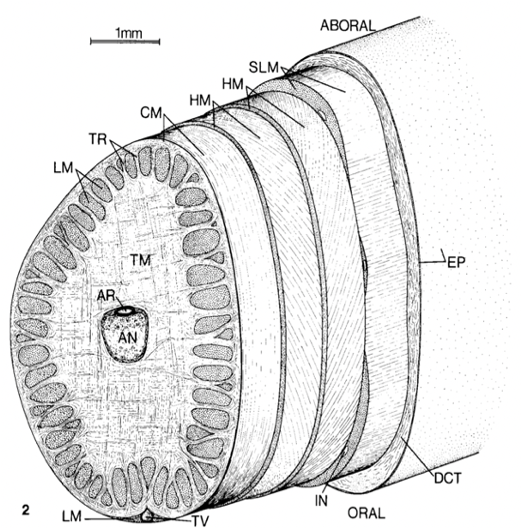
Fig. 1 Cross section of the tentacle stalk showing the muscle layout. TM-transverse muscle, LM-longitudinal muscle, HM-helical muscle, CM-circular muscle, AR-artery, DCT-dermal connective tissue, EP-epithelium, IN-intramuscular nerve cord, SLM-superficial longitudinal muscle, TR-trabeculae of transverse muscle, TV-superficial tentacular vein (Kier, 1982).
The majority of the musculature of the tentacles and arms of cephalopods is obliquely-striated muscle as in many other invertebrates, meaning that the thick and thin myofilaments are arranged in a staggered array along the long axis of the fiber (Kier, 2016). This forms an oblique or helical pattern of A-bands (containing thick filaments), I-bands (containing thin filaments), and Z-material or dense bodies, as seen in Figure 2. This oblique striation of myofilaments is likely meant to accommodate movements across the length of the appendage. Furthermore, the myofilaments of muscle fibers surround a central core containing mitochondria and a single cell nucleus. It has been found that the fibers of cephalopod tentacles (and arms) are predominantly mitochondria-poor and thus function anaerobically in order to accommodate short-term maximal efforts.

Fig. 2 Schematic diagram of a cephalopod obliquely-striated muscle fiber. A, A-band; I, I-band; M, mitochondria core; S, sarcoplasmic reticulum; Z, elements (Kier, 2016).
In typical vertebrate muscle, the shortening velocity of fibers is often influenced by the myosin isoforms they contain (Kier & Schachat, 1992). In other words, the speed at which the sarcomeres of muscle fibers contract is typically attributed to the specific form of myosin they contain from a family of functionally similar proteins whose amino acid sequences only slightly vary (Li & DeMayo, 2018). These isoforms may include myosin heavy chains, myosin light chains, troponins, and tropomyosins, and variation in these proteins has been found to affect physiological performance. However, studies conducted to analyze the myosin heavy chain mRNA transcript sequences and expression profiles from the various appendages of different species of squid, cuttlefish, and octopus have shown little difference in myosin isoforms amongst the muscle types. This evidence, therefore, further suggests that the ultrastructure of the tentacular muscle fibers specializes them for high shortening velocity (Kier & Schachat, 1992; Kier, 2016). Indeed, the short sarcomere and thick filament lengths contribute to the ten-fold increase in shortening velocity between the arm and tentacle muscle fibers due to the shortening velocity of elements in series being additive.
Nerve signal conductance
The rapid shortening velocity of the transverse muscle fibers and their greater force output can also be explained by the specialization of the peripheral nervous system in cephalopod tentacles (Gilly et al., 2020). In fact, it has been found that the tentacles of cephalopods possess a 20-fold higher twitch-to-tetanus force ratio than the arms, which suggests greater electrical excitability and thus the ability to produce faster and more powerful reactions to the organism's environment. This faster response rate and explosive force development can be attributed to the greater expression of voltage-gated sodium channels in tentacle muscle tissue (Gilly et al., 2020). These large integral membrane proteins are predominantly expressed at neuromuscular junctions and selectively conduct sodium ions into muscle fibers when a chemical message is being transmitted from the terminal branch of the axon of a motor neuron (Simkin & Bendahhou, 2011).
A study conducted by Gilly et al. investigated the conductance of sodium and potassium ions in the neurons of tentacle and arm tissue in the squid Doryteuthis pealeii. After using an antisense probe to D. pealeii GFLN1, the unique voltage-dependent Na channel in the species, there was found to be intense labeling of the mRNA encoding this channel around the axial nerve cord, as well as the transverse muscle fibers which are responsible for rapid extension of the tentacle during prey strike. However, there were no detectable Na channel signals in the transverse muscle fibers of the arm, with this contrast clearly displayed in Figure 3. This supports the idea that only tentacle muscle fibers produce action potentials, leading to a greater twitch-to-tetanus ratio. In fact, the greater concentration of Na channels allows for maximal activation during tentacle strike due to a few closely spaced action potentials as opposed to the absence of such signals–and rather a series of postsynaptic potentials over a longer period of time–in arm fibers. This can be shown in Figure 4 by the virtual absence of Na current in arm tissues and a significant presence in tentacle tissues at a given applied voltage. It was therefore found that the maximum sodium conductance density was ten times greater in tentacles versus arms. Additionally, the activation of potassium current (IK) at a given voltage was slower in tentacle fibers than arm fibers, which coincides with the fact that sodium current (INa) activates much faster than IK during the transmission of an explosive action potential.

Fig. 3 a) Low magnification image of the cross section of the tentacular stalk of the squid Doryteuthis pealeii hybridized with an antisense Na channel probe showing labeling in the transverse muscle mass and axial nerve cord. b) Tentacle cross- section with a negative control probe showing no labeling. (c, d) High magnification views of boxed regions of a) and b). e) Cross section of arm with the antisense Na channel probe showing no labeling in the muscle mass and only in the axial nerve cord. f) Arm cross section hybridized with negative control probe showing no labeling. (g, h) High magnification views of the boxed regions of e) and f). Imaging technique not specified (Gilly et al., 2020).
Interestingly, the same homolog of the Na channel mRNA was detected in D. pealeii, the California two-spot octopus, Octopus bimaculoides, and the Hawaiian bobtail squid (Euprymna scolopes), thus indicating that a single gene may encode voltage-dependent Na channels in cephalopods (Gilly et al., 2020). Another unique feature of cephalopod tentacle muscle activation is that the excitability of tentacle fibers is graded because of the variation of sodium conductance throughout the appendage. In fact, while this feature of invertebrate muscle is often associated with calcium ion channels, graded Na conductance results in a faster rate-of-rise of action potentials along tentacle fibers. This provides greater control and accuracy during prey strikes by enabling peripheral elements, such as muscle tissues, to play a more significant role in coordinating neuromuscular outputs.

Fig. 4 a) Inward and outward sodium current recorded in K-free solutions from a transverse tentacle fiber at -70mV. b) INa recorded from a transverse arm fiber at -70mV [Adapted from Gilly et al., 2020].
Tentacle adhesion
Another important property of cephalopod tentacles is their adhesive abilities. The adhesion of cephalopod tentacles can be achieved not only through mechanical mechanisms (such as the use of suckers), but also through the use of adhesive substances (von Byern & Klepal, 2006). For instance, this biochemical mechanism is observed in the Nautilus tentacle, which lacks suckers and arm hooks. Indeed, this adhesive ability is necessary for handling prey, attaching to the substratum, or for clinging to another individual during mating. The thick epithelium on the proximal surfaces (as seen in Figure 5), therefore, contains columnar cells that release a secretory material consisting of granules made of neutral mucopolysaccharides (long chains of repeating saccharide units found in mucus) and glycoproteins. These glycoproteins, also referred to as mucins, consist of various protein domains, which are then glycosylated by O-linked and N-linked oligosaccharides (Bansil & Turner, 2018). On the other hand, the thin epithelium, found on the distal surfaces of the tentacle, contains mucous cells with acidic mucopolysaccharides, which are thought to help increase the viscosity of the mucus and thus make it more adhesive. Although this mechanism has not been greatly investigated in cephalopods, the adhesive and viscous properties of the mucous can likely be attributed to the various intermolecular interactions that mucopolysaccharides and glycoproteins are capable of forming with other molecules, as in the mucoadhesive mechanisms of other organisms (Boat & Cheng, 1980). For instance, hydroxyl, carboxyl, and amine groups of the glycoproteins form hydrogen bonds, anionic groups and cationic components form ionic interactions, and other functional groups form covalent bonds, van der Waals interactions, and hydrophobic interactions with molecules to which they adhere (John, 2005). Important to note, however, is that the aquatic environment influences this adhesive mechanism. In fact, certain adhesives are activated by moistening and thus require the uptake of water in order to strengthen adhesion. However, substantial mucus layers are required in order to reduce the amount of slippery mucilage due to overhydration (John, 2005). Macroscopically, the epidermis of the Nautilus' tentacle also contains transverse ridges and grooves which are more pronounced on the oral side of the appendage in order to facilitate handling of prey (see Figure 5). Finally, mechanical detachment from the tentacle is achieved via bending movements of the longitudinal and radial musculature (von Byern & Klepal, 2006).

Fig. 5 Structure of a longitudinal digital tentacle of the Nautilus including the muscular cirrus. The epithelium of the transverse ridges is thin on the distal surface but thick on the proximal surface (von Byern & Klepal, 2006).
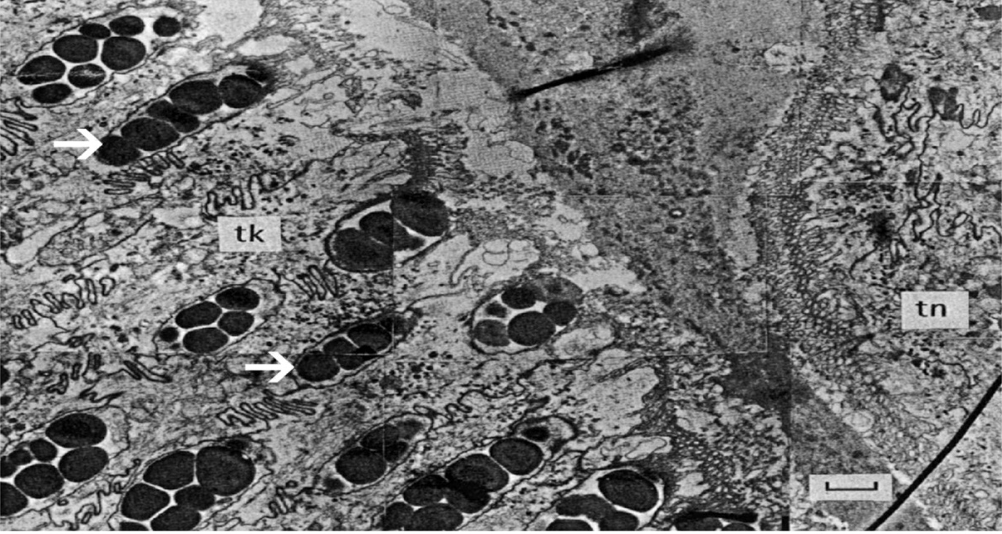
Fig. 6 Transmission electron microscopy (TEM) photograph of a cross section of the Nautilus digital tentacle. Electron-dense granules of the goblet cells are present in the thick epithelium (tk) as indicated by the white arrows. The thin epithelium (tn) lacks granules and instead contains mucus cells which are not present in this image (von Byern & Klepal, 2006).
Regeneration
Another fascinating property of the cephalopod tentacle is its ability to regenerate upon amputation (Imperadore & Fiorito, 2018). Indeed, arm and tentacle damage is a common occurrence amongst cephalopods, making this regenerative ability quite critical for survival. This process can be divided into three stages: wound healing, tissue degeneration, and renewal.
Upon tentacle lesion and wound healing, muscles close to the wound begin degenerating, during which the sarcoplasm (cytoplasm of striated muscle cells) breaks down and the spiral fibers grow thicker (Imperadore & Fiorito, 2018). Muscle fibers will also begin to lose their cylindrical shape and form a “clotty mass.” The epithelium also begins to regenerate as old material rearranges itself and covers the scar tissue. This forms a structure known as the primary blastema, which supplies material for the regenerating connective tissue. Muscle nuclei also fragment into two or three particles and are absorbed by the corpuscles that migrate from the blood to the muscles. Muscle regeneration then begins with the appearance of large cells containing a large nucleus and little protoplasm. These cells, known as sarcoblasts, originate from an area where tissue has degenerated and can develop into new muscle fibers. They, therefore, move to the most distal part of the wound and work with neuroblasts—undifferentiated neural stem cells—to create the second blastema via mitotic division. After twelve to fourteen days, the sarcoblasts differentiate into different muscle fibers.
The nerve cord also begins to degenerate soon after amputation, starting with the nuclei of the layer of ganglion cells (Imperadore & Fiorito, 2018). The nuclei of glial cells shrink and the fibers of the myelin cord swell. After ten hours, the number of nuclei increases in the neuropil and myelin cord with the migration of blood corpuscles and the amitotic division of glia nuclei. Neuroblasts eventually migrate to the distal part of the amputation to help form the second blastema.
Renewal is a longer process than degeneration and begins with the differentiation of cells, such as that of neuroblasts into ganglion cells, protoplasm, and fibers of the newly forming myelin cord. The axial nerve also tends to occupy the majority of the regenerating stump. While the nervous system has been thoroughly investigated, fewer studies have observed the regeneration of the vascular system.
The six principal stages of the regeneration process are summarized below in Figure 7. The images represent the regeneration of a cuttlefish arm; however, the same process occurs in the tentacles of cephalopods (Imperadore & Fiorito, 2018). In the first stage, degeneration of the nerve cords, muscles, and blood vessels is accompanied by cell dedifferentiation–when cells lose their particular features and become a source of regenerative cells—and epidermis eventually covers the wound. The end of stage 1, as well as stages 2 and 3, represent the start of regeneration, which includes blastema formation, cell activation, and the growth of new tissue. The brachial artery and axial nerve cord also penetrate the blastema. Finally, in the last stages, the appendage regrows and cell differentiation occurs. This begins with the differentiation of neuroblasts into nervous tissue, followed by the growth of muscle tissue surrounding the axial nerve cord and the development of myofilaments within the muscle. As well, the basal membrane of the epithelial cells appears with differentiation, upon which it folds to form the starting structure of the suckers, as well as their suction and adherent chambers.

Fig. 7 Schematic overview of the series of events that occur during the regeneration of the cuttlefish arm. A similar process occurs during the regeneration of tentacles (Imperadore & Fiorito, 2018).
Altogether, the complex process of tentacle regeneration in cephalopods further emphasizes how the biochemical properties of this appendage have evolved to best support the survival of cephalopods. Not only is the appendage capable of deconstructing its damaged parts, but it also uses these parts as starting material for its newly formed tissues. This can indeed serve as inspiration for future research in regenerative medicine (Kier, 2016).
Chemosensation
Cephalopods have complex nervous systems with remarkable sensory abilities, making them a very interesting subject to study. They detect chemical cues in their environment through multiple chemosensory organs, including the chemoreceptors on the suckers of the arms and tentacles. The organization of the axial nerve cord that runs down the length of each arm and tentacle makes their chemosensation particularly unique.
Nervous system of cephalopod tentacles and arms
Unlike the nerves of humans, which consist only of axons, the longitudinal nerve cords running down each arm or tentacle consist of a continuous series of ganglia (swellings along the nerve cord), including suction-cup ganglia that lie outside the nerve cord; and therefore the nerve cord acts more like a spinal cord than a nerve, with its structure represented in Figure 8. This means that there can be a motor response even when an arm or tentacle is impaled and disconnected from the brain. It thus leads to faster motor response upon sensory stimulus. Furthermore, a study by Kaitlyn E. Fouke and Heather J. Rhodes showed that the chemosensory cells on the arms of cephalopods were able to elicit a response when in contact with certain amino acids and fish skin extracts. They were thus able to determine that the octopuses studied in the experiment were able to detect chemical signals associated with their prey via these appendages. The signals recorded during the experiment include sensory information, efferent copies of motor commands sent to the proximal segments of the amputated arm, and local activity in the ganglia of the arm adjacent to the electrode. Although this experiment focused on amputated octopus arms, it can also be applied to cephalopod tentacles.
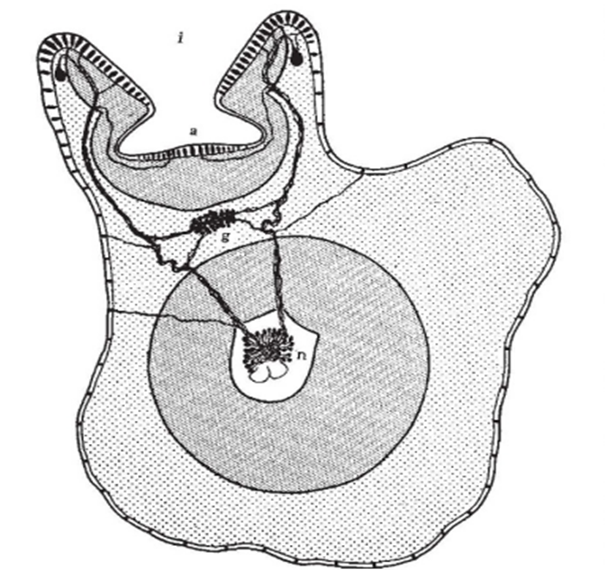
Fig. 8 Cross section of the arm of an octopus where a (acetabulum) and i (infundibulum) are two sections of the sucker, and each of these sections is covered with a prismatic epithelium of chemical and tactile sensory cells. We can see that the sensory information is sent to the ganglion (g) and then routed to the nerve cord of the octopus arm (n) in order to elicit a motor response. This is how an octopus arm, similarly to the tentacle, can move without being in contact with the brain (Graziadei 1962).
Neurotransmitters and receptors
Several cephalopods, such as benthic octopods, use their different receptors located on their arms and tentacles to search for food in hard-to-reach places by utilizing the chemosensory and somatosensory abilities of these appendages. Andouche et al. (2021) analyzed the ionotropic glutamate receptors (IGluR) of cephalopods in order to identify which chemosensory organs have ionotropic glutamate receptors that developed chemosensory capacities (IR), a subfamily of IGluR. They noticed that the suction cups of the arms and tentacles were covered with them, which explains how cephalopods can smell their prey by chemical detection. They also found that the octopus species has a homologous gene encoding the chemosensory co-receptor IR25, Soff-IR25 (Andouche et al., 2021). Thus, they searched for Soff-IR25 expression in whole embryos of the late pre-hatch stage and found it on the cephalopod's suckers, suggesting that the sensation of those animals when they analyze their prey is close to olfaction. Figure 9 represents how and where they found the Soff-IR25 genes. The expression of the different Soff-IR25 genes are as follows: Soff-IR25-F (5′-GGCAGTTTGGCACTACCCTA-3′) and Soff-IR25-R (5′-TG GATTCACTGAAGGCAGGA-3′).

Fig. 9 Images displaying the suction cup of the embryo, consisting of a pseudostratified sensory epithelium. The tissues were obtained after cryostat sectioning of the whole-mount in-situ hybridization. The red staining shows that these cells are positive to Soff-IR25 and are therefore chemosensory cells. B (200 µm), B' (100 µm), and B'' (50 µm) are increasingly magnified to give a closer look at the position of the Soff-IR25 (Andouche et al., 2021).
Altogether, evaluating amputated arms helped establish that it is the chemical and tactile receptors on the suction cups that permit the observed muscular reaction. Had the octopus been in its natural state, it would have been impossible to determine whether the actions were directed by the brain or by the tentacle's complex nervous system.
Gastropods
Slugs and snails make up a group of animals called gastropods, a subsection of the much broader mollusk family. There are three subsections of the gastropod family: the prosobranchs, the opisthobranchs, and the pulmonates (Croll, 1983). These three classes have many things in common; however, this section will focus on their sensory tentacles. Snails and slugs usually have at least one pair of tentacles, and many species, such as Stylommatophora have two pairs, as seen in Figure 10. While gastropod tentacles are similar to those of cephalopods in that they can move omnidirectionally and lack any skeleton, their primary function differs greatly. Cephalopods primarily use their tentacles for prey capture (Kier, 1982), which, as seen in the previous section, involves both chemoreception and specialized musculature. For gastropods, tentacles primarily function as sensory organs and are the location for chemoreceptors (Croll, 1983). It turns out that olfaction is the primary means by which gastropods perceive and navigate their environment.
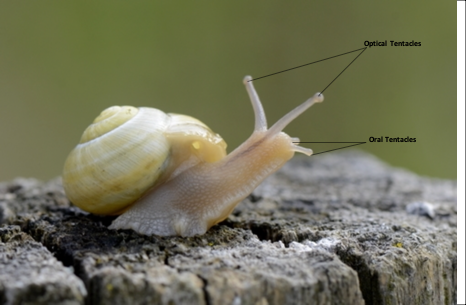
Fig. 10 Two pairs of tentacles in Stylommatophora. [Adapted from Zwahlen, 2018].
Sense of smell plays an important role in much of a gastropod's life, including food finding, predator avoidance, and mating (Emery, 1992). Many of the olfactory chemoreceptors are located on the sensory tentacles. Each tentacle has two layers surrounding its tip: above is the epithelium and below is the muscle. Figure 11 outlines the anatomy of the gastropod tentacle. Below the layer of muscle is the complex neural system of the tentacle (Chase & Tolloczko, 1993). Neurons can act as chemoreceptor cells, and in gastropods they are usually located in the epithelia or below the epithelia (Emery, 1992). In the latter case, dendrites connected to the primary neuron pass through the epithelia to the surface of the cell. For a chemical to be recognized via olfaction by a gastropod, the neuron must express receptors that can attach to specific odorants (Di Cosmo & Winlow, 2014). This receptor will then relay information to the central nervous system. Similar to the cells of mammalian nasal cavities, the cells of the epithelia covering the tip of the tentacle are often ciliated (Emery, 1992). Cilia are hair-like structures that can push chemical odorants toward receptors to be recognized.
Gastropod chemoreception
It is difficult to generalize chemoreception for the entire family of gastropods. Instead, this section will look at various experiments whose findings have outlined the varying uses of olfaction within this group of animals. Experiments that have identified chemicals that induce responses in gastropods will also be discussed.
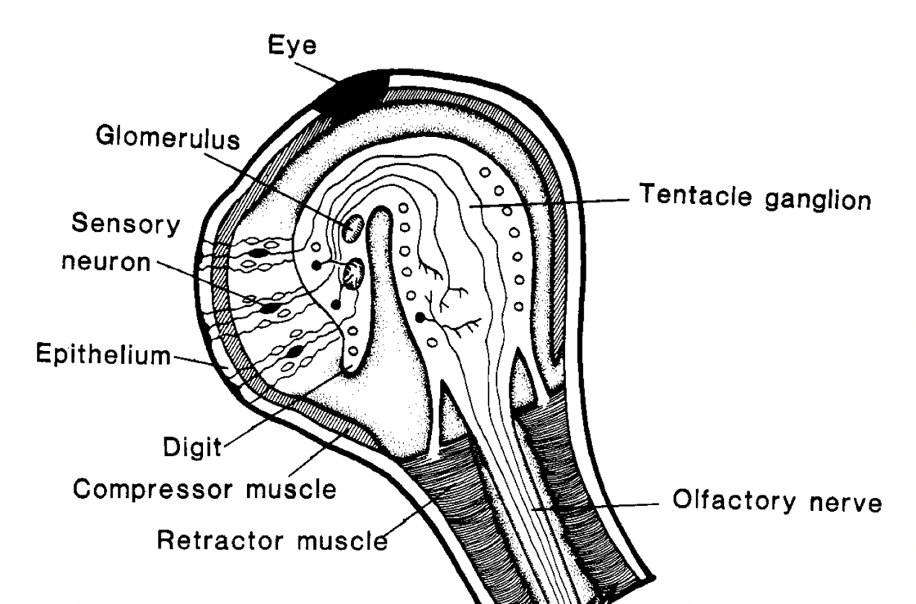
Fig. 11 Anatomy of the snail's olfactory system located in the optic tentacle. [Adapted from Chase & Tolloczko, 1993].
A study conducted by Feder (1963) showed that different species of gastropods were able to sense the presence of a predatory starfish Pisaster. It was observed that when a calm tide pool was disturbed by something other than a starfish, gastropods within would not flee. However, if a starfish was placed in the pool the gastropods would move away from the predator, sometimes exiting the tide pool. With olfaction being one of the only well-developed senses in gastropods, it was suggested that some chemical given off by the starfish was being recognized by the gastropods. In a later study, a chemical from the feet of Pisaster was at least partially purified (Feder & Lasker, 1964). This chemical when placed near gastropods caused them to flee. Another interesting result from this study was that solutions high in salt or distilled water caused a similar response to the compound from starfish.
A relatively early field study by Edelstam & Palmer (1950) pushed the homing abilities of the gastropod Helix. They observed that these snails could orient toward their homes from up to 150 meters. Olfaction was assumed to be the primary sense allowing this to occur, but questions about the memory of these snails arose. Gastropods are of interest to neuroscientists because of their relatively large identifiable neurons. A long-term memory for food odors in the Land snail, Achatina fulica, by Croll & Chase (1977), focused on gastropod memory and learning ability. Two separate groups of snails were fed two different foods—carrot and cucumber—for several days. They were then starved and tested for preference between carrot and cucumber odor in an olfactometer. Both groups were then fed with lettuce. After many starving, testing, and lettuce-feeding cycles it could be seen that the snails preferred their original food source odor. However, their preference lessened as time went on signifying that their memory deteriorated.
Aplysia is a relatively large sea slug that has been studied extensively because of the role that its pheromones play in mating. It was observed that sexually mature slugs tended to congregate in groups and that these groups were either sexually active or not. They also observed that if one slug lays its eggs, other slugs within the same vicinity would lay eggs as well. These observations strongly suggested that pheromones play an important role in synchronizing the Aplysia mating cycle. Many years of research led to Painter et al (1998) discovering “the First Water-borne Pheromone in Invertebrates” (p. 1), namely Aplysia attractin. In a later study, Cummins et al. suggested that the four protein pheromones attractin, temptin, seductin, and enticin together attract Aplysia mates, and keep large groupings of slugs together during mating. Figure 12 shows some candidate chemoreceptors located on the oral tentacle of Aplysia that may recognize important pheromones.
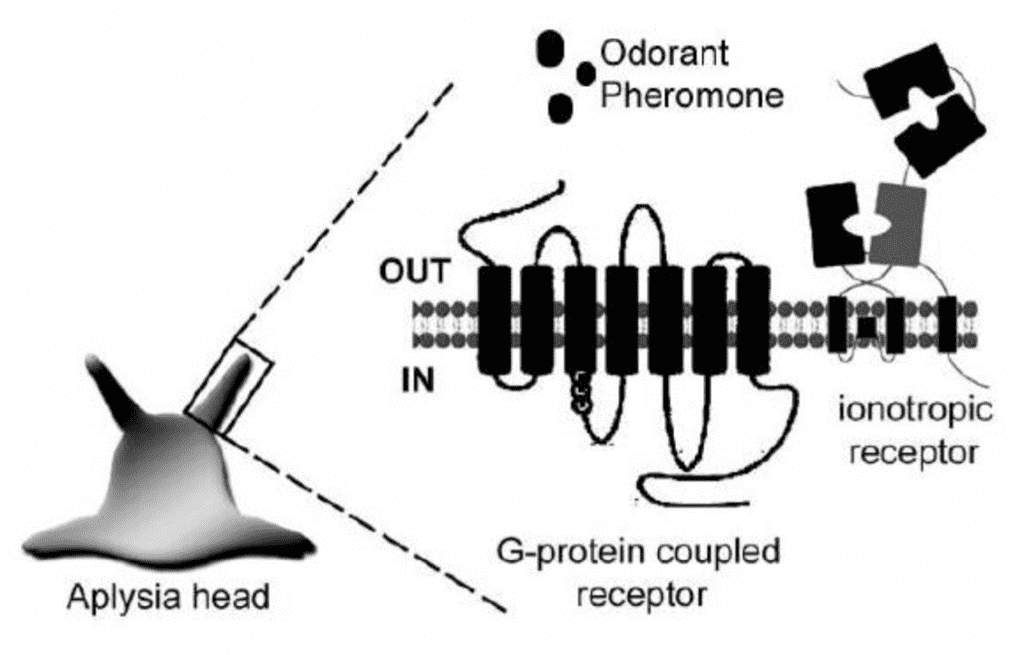
Fig. 12 Possible chemoreceptors located on the oral tentacle of the snail Aplysia (Di Cosmo & Winlow, 2014).
Cnidarians
The nervous system of jellyfish
Jellyfish are one of the first organisms to develop neurons, and yet, today, their nervous system is considered one of the simplest. Jellyfish operate with no brain and instead have multiple nervous systems distributed across their bodies (Katsuki & Greenspan, 2013). These systems allow jellyfish to adapt to their environment by sensing light, orientation, and gravity. Most importantly, however, the nervous system helps jellyfish capture prey through multiple steps of paralysation, contraction, and digestion (Pallasdies et al., 2019).
The jellyfish Scyphomedusae's nervous system has three main components: the rhopalia (as seen in Figure 13), the motor nerve net, and the diffuse nerve net. The rhopalia contain photosensitive structures called oceli, and gravity or directional receptors called statocysts. The rhopalia aids the jellyfish when swimming by sensing light and gravity, encapsulating the swimming pacemakers. The motor nerve net is responsible for the contraction of muscles, and it is spread throughout the subumbrella of the jellyfish (Katsuki & Greenspan, 2013). The diffuse nerve net, shown in Figure 14, sends sensory information to the rhopalia, which helps create the ideal rhythm for swimming. It also helps innervate the contraction muscles along with the motor neuron net, which provides variability in contraction strength (Satterlie, 2011).
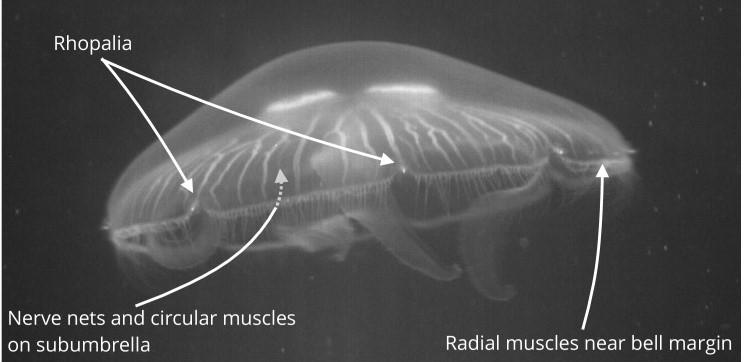
Fig. 13 The Scyphomedusae jellyfish. The rhopalia is where most concentration of neurons occur. There are usually eight rhopalia around the bell of the jellyfish (Pallasdies et al., 2019).

Fig. 14 Image of the diffuse nerve net around the muscles of the subumbrella (Satterlie, 2011).
Nematocysts' structure and production
Nematocysts, the stinging organelles of jellyfish, are pressurized cylindrical capsules with toxic threads encapsulated within (Beckmann & Özbek, 2012). Nematocysts are covered with a lid-like structure called an operculum, and as seen in Figure 15, they also have extremely sensitive hair-like structures called cnidocils. When touched, cnidocils activate the nematocysts causing the operculum to fly open, releasing the venomous thread (Lumen Learning, 2022). This allows the nematocysts to activate on its own command without needing a signal from a brain or a central nervous system. Figure 16 shows how the thread, which looks like a harpoon, is coiled inside the capsule. The thread, which can be seen in Figure 17, consists of a rigid shaft made of three helically coiled filaments and lined up with spines. The tips of these filaments are compressed to create a sharp tip that is able to penetrate the surface of the target organism. In addition to the shaft, the thread also consists of a long thin tubule that is lined up with barbs (Godknecht & Tardent, 1988). During discharging, the shaft goes headfirst followed by the tubule, which comes from inside the shaft and out of the tip.

Fig. 15 Basic structure of nematocysts before they are activated. The thread is coiled and encapsulated in the nematocysts with a hair-like structure called cnidocils sticking out (Lumen Learning, 2022).
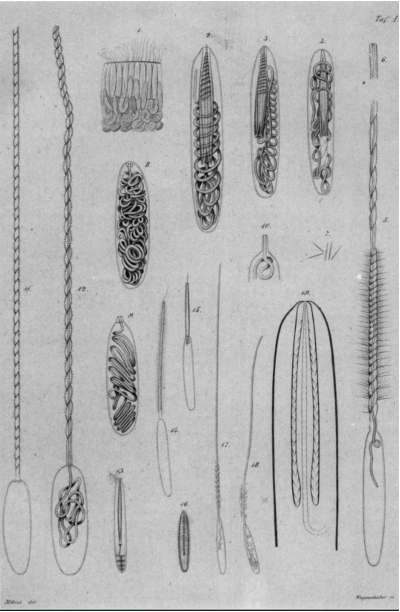
Fig. 16 An early drawing of different types of nematocysts found in cnidarians drawn by Möbius in 1866. Some of the drawings (left) show the stages of nematocysts' discharge (Pierre, 1988)

Fig. 17 The thread of the nematocysts when it has been discharged. The shaft, which is made of tubules, is lined up with spines. The top left picture shows the beginning of the shaft where spines are more spread apart, while the top right picture shows the middle of the shaft until the tip where spines are more condensed. The bottom picture shows the three compressed filaments that create a sharp tip (Östman, 2000).
Nematocysts are produced in the “nematocytes” cells, often called cnidocytes, which are specialized neural cells. Nematocysts are produced in the cytoplasm of these cells starting from the Golgi apparatus. As shown in Figure 18, the nematocyst starts to grow by the addition of different structural proteins from the Golgi apparatus into a vesicle. The formation of the thread begins at the tip of the vesicle where tubules start to form. Once the tubules form, invagination occurs where the thread is coiled around itself and placed into the vesicle followed by the operculum to close the vesicle (Beckmann & Özbek, 2012). Finally, the nematocyst matures by increasing the density of the structure's wall. Outside the vesicle, a protein called Nowa works with minicollagens inside the vesicle to create a double-layered wall that provides nematocysts with mechanical stability (Engel et al., 2002).
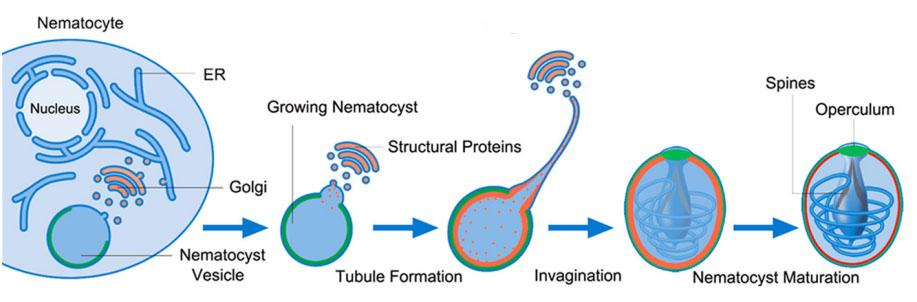
Fig. 18 A simplified overview of the formation of nematocysts starting from a nematocyte (cnidocyte) (Beckmann & Özbek, 2012).
The stinging mechanism
Jellyfish have a great dependency on their stinging mechanism. It gives them an advantage over their prey and provides a reliable defense mechanism against predators. When jellyfish find prey, they wait patiently for it to come close to their tentacles, which contain stinging organelles called nematocysts. Once nematocysts are in contact with the prey, they paralyze it. The paralyzed prey is reeled in toward the jellyfish's mouth by contraction of the tentacles, and digestion begins.
Jellyfish's stinging is one of the fastest biological mechanisms known today. Nematocysts can discharge within 700 nanoseconds thanks to the energy released from the stretched collagen inside the nematocyst's walls (Nüchter et al., 2006). The stinging mechanism, shown in Figure 19, first starts with a trigger caused by touching the cnidocils, which causes the operculum to open and release all the pressure building up inside the nematocyst. The shaft of the threads goes flying out until it is fully extended and the filaments at the tip of the shaft start turning inside out revealing another smaller tip of filaments that also turn inside out. This shaft eversion occurs multiple times at the tip, which ensures that the thread will successfully penetrate the surface of the prey. Once the shaft eversion is done, the tubules also shoot out of the nematocysts through the inside of the shaft and come out of the open tip of the shaft (Karabulut et al., 2022). The tubules also undergo eversion by untwisting the tubules and reforming it into a cylindrical shape (Skaer & Picken, 1965). Depending on the type of nematocyst, neurotoxins could be discharged due to the osmotic pressure in the nematocyst. These neurotoxins travel through the shaft and the tubules, until they reach the untwisted open tip of the tubules where, if the thread penetrates the prey successfully, they are released to the tissue of the prey, thus paralyzing or killing it (Godknecht & Tardent, 1988).
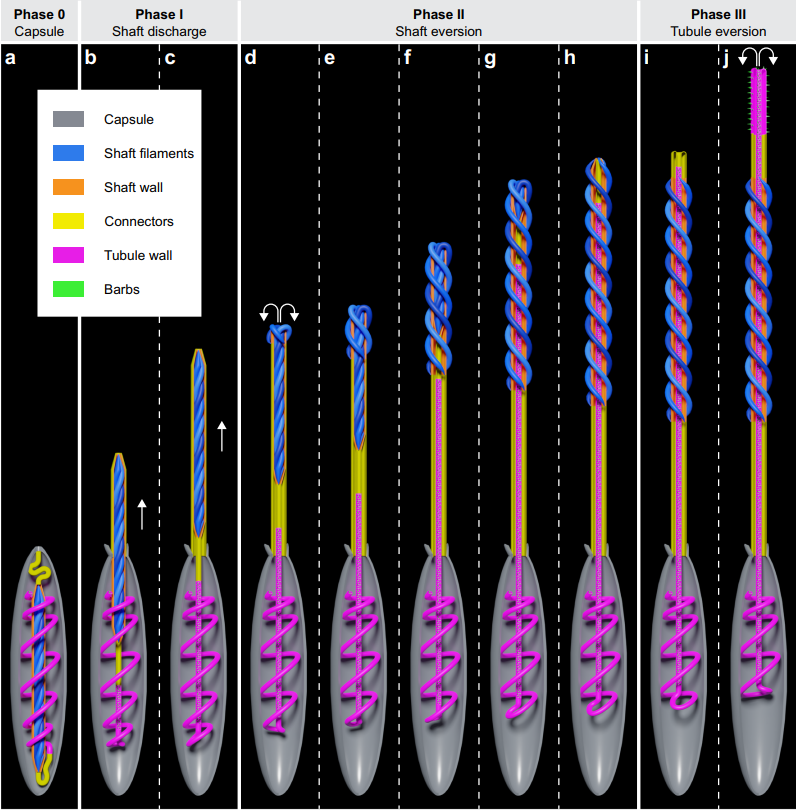
Fig. 19 Schematic diagram showing what nematocyst discharge looks like. It begins with the shaft shooting up and everting, followed by the tubules, which reach the tip of the shaft and keep going beyond that, and, finally, untwist and unfold at the tip when fully extended.
How a jellyfish's sting affects its prey
A jellyfish's sting is not only painful to the prey, but it also paralyzes the prey or even kills it. Neurotoxins made of proteins are responsible for paralyzing the prey by affecting their voltage gated sodium channels (Watson & Mire-Thibodeaux, 1994). Voltage-gated sodium channels are responsible for initiating the action potential inside neurons and thus allowing signals to travel from one neuron to another, which is effectively how muscles are contracted. The neurotoxins affect these channels by prolonging the action potential, which causes an increase in the number of electrical transmissions. The increased number of transmissions makes the muscles contract rapidly, which paralyzes the prey (Rathmayer & Beress, 1976).
Sea anemones and hydra
As seen in Figure 20, the nervous system of the tentacles of the most primitive cnidarians such as sea anemones and hydras consist of a large nerve net. One peculiarity is that the neurons are sensory and motor at the same time, and since there is no centralization, it is the equivalent of an enormous arc reflex. Within each tentacle contacting the stimulus, there is an action potential, a neurosecretion, and neuropeptides synthesized by the neurons to transmit the information. Interestingly, the nervous system of these animals is one of the first to have evolved in the animal kingdom.
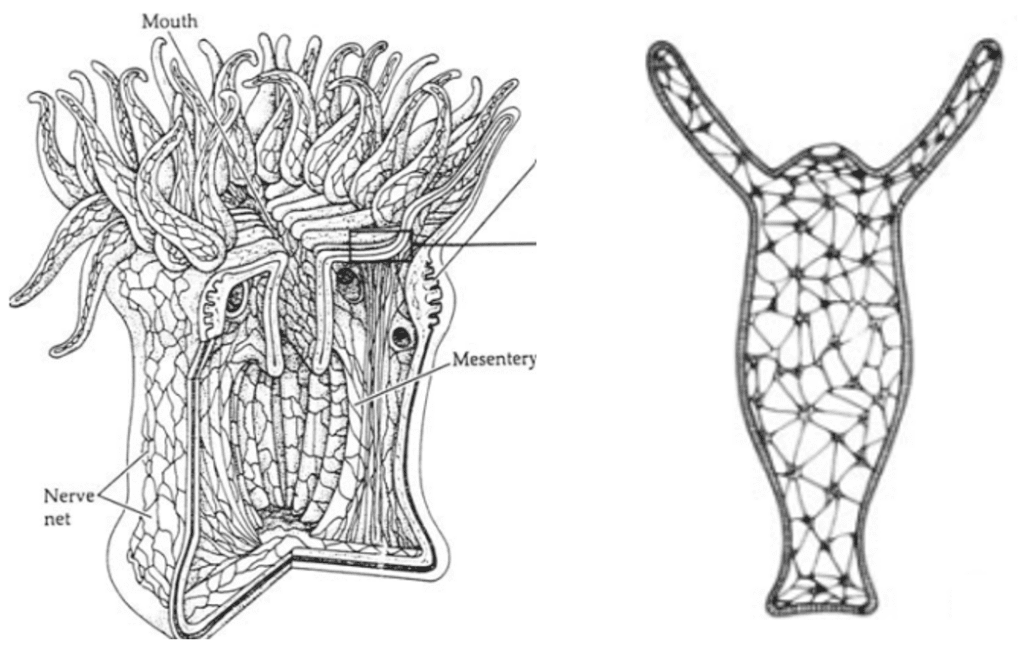
Fig. 20 Nerve net of the sea anemone at the left and that of the hydra at the right. As seen, there is no centralization, and all neurons are sensitive and effective at the same time. This can be compared to the giant arc reflex (Satterlie, 2015).
Role of nematocysts and other cells in cnidarian tentacles
As previously explained, cnidarian tentacles have nematocyst cells. There are several types of nematocytes from which the nematocysts originate, three of which are presented in Figure 21: isorhiza, desmoneme, and stenotheles. Nematocysts are mainly located on the tentacle and consist of a small collagen capsule and a cover which seals the cyst. They are filled with venom, which can be discharged into the tissues of other organisms to paralyze them upon proper external stimulus. Discharge of the poison can occur after only three milliseconds when the epithelial cells encounter a stimulus. All of the sea anemone and hydra's toxins contain peptide chains of 9 to 17 amino acids, ending in Lys-Arg. Toxin proteins are composed mainly of polar (16.7%) and charged (57.5%) amino acid residues (Inderluh et al., 2000).
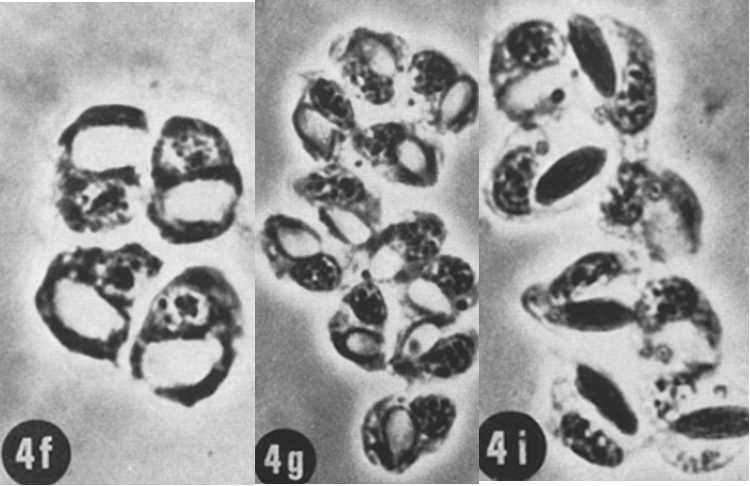
Fig. 21 (4f) Nest of 4 developing stenotheles nematoblasts. (4g) Nest of 11 desmoneme nematoblasts. (4i) Nest of 8 isorhiza nematoblasts. (David, 1972)
Indeed, there are also other cells in the hydra and sea anemone's tentacles. As we know, tentacles have sensory cells that can create a reaction when the neuropeptides are secreted. However, these reactions are not possible without the role of the ganglion cells that create the nerve net with the sensory cells. There are also battery cells that cover most of the exterior of the tentacle and gland cells for secretion of mucus. This mucus explains why tentacles are sticky. There is no free space between tissues, and instead there are cells called interstitial cells. All these different cells are demonstrated in Figure 22.
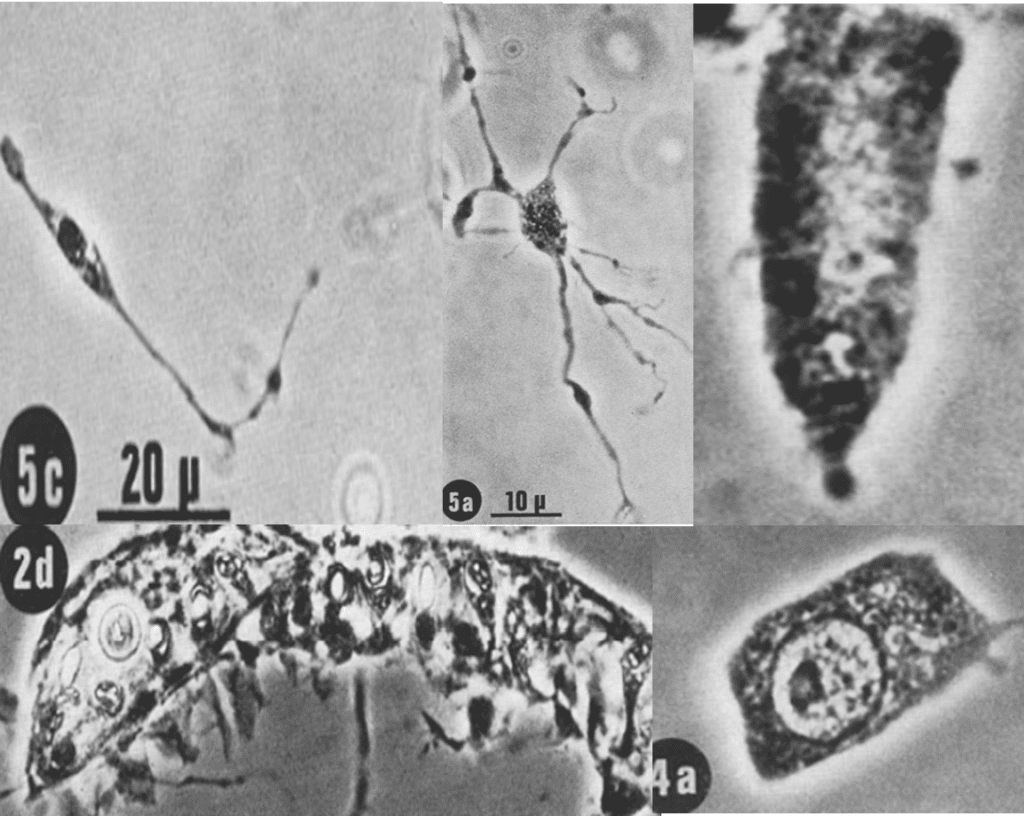
Fig. 22 (5c) Representation of a sensory cell. (5a) A ganglion cell, the most common type of nerve cell in tentacles, which composes the nerve net and the whole nervous system alongside the sensory cell. (2d) Two battery cells containing nematocytes. Which are mainly in the ectodermal epithelial cells of the tentacle. The one without identification in the upper right corner is a gland cell (occurs at the base of the tentacle and is called hypostome in the hydra). This is the mucus-secreting type (secretory type 1 granules) and is usually referred to as a mucous cell. As we can see, the cytoplasm is filled with secretory material which almost obscures the nucleus at the lower end of the cell. (4a) An interstitial cell which lies in the spaces between the functional cells of a tissue. A live-cell imaging technique with time-lapse microscopy was used to obtain these images (David, 1972).
Transmitters
Cnidarians mainly use neuropeptides passing through dependent ion channels to carry out neuronal communications. Some neurotransmitters exit but it is proven that the principal way of communication between cells is by secretion of neuropeptides. As far as the movement of the tentacles is concerned, it is mainly a specific neuropeptide that acts on the muscle and thus causes a movement. Some of these neuropeptides can be seen in Figure 23 below.
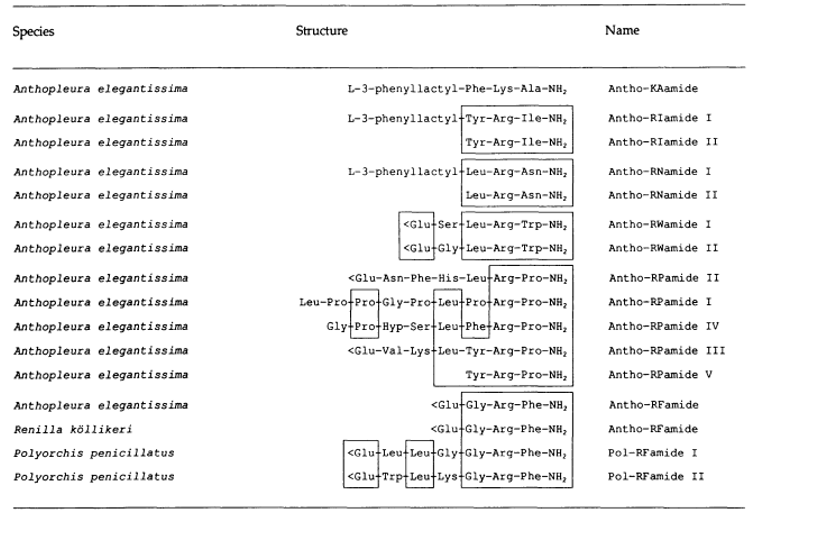
Fig. 23 List of different neuropeptides associated with some species of cnidarians (Westfall, 2005).
With a closer look at some neuropeptides specific to the tentacles of sea anemones, we notice that some peptides can act like human neurotransmitters. In fact, the tentacle's peptides can either activate or inhibit muscles, just as neurotransmitters regulate the activation of muscles in the human body. Some examples of these peptides are represented in Figure 24.
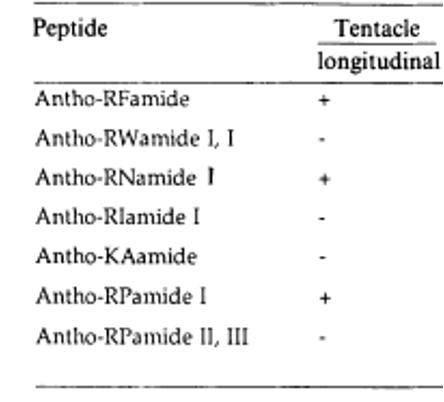
Fig. 24 Examples of the neuropeptides that act on the tentacles of sea anemones, either activating (+) or inhibiting (-). Activating peptides provoke movement and inhibiting peptides provoke muscle relaxation (Westfall, 2005).
Conclusion
While the kinematics of tentacles, like those of the octopus or squid, are exciting and can be observed in the wild, tentacles in general perform a wide variety of functions and have evolved to be extremely diverse in nature. For instance, it has been shown that squid tentacles contain a higher concentration of sodium ion channels within their muscle tissue, allowing for high velocity strikes. Different species of gastropods rely almost exclusively on olfactory chemoreceptors located on their tentacles to interpret and navigate their environment. Jellyfish tentacles are laden with toxin bearing cells called nematocysts, which allow them to immobilize their prey by attacking their nervous system. These jellyfish are members of the cnidarians, which have one of the most rudimentary nervous systems, indicating they may be related to the first animals with nervous systems. There are also some very niche adaptations such as the adhesive or regenerative properties of the squid tentacle, which are controlled by complex biochemical processes. Altogether, the varied biochemical functions of the tentacle emphasize its multifaceted purpose and the efficiency of its composition. Despite being a distal organ, it demonstrates an evolved extension of the nervous system while possessing specialized cells and organelles. Tentacle bearing organisms are capable of interacting with, navigating, and manipulating their environment thanks to this specialised appendage.
References
Anderluh, G., Podlesek, Z., & Maček, P. (2000). A common motif in proparts of Cnidarian toxins and nematocyst collagens and its putative role. Biochimica Et Biophysica Acta (BBA) – Protein Structure and Molecular Enzymology, 1476(2), 372–376. https://doi.org/10.1016/s0167-4838(99)00237-x
Andouche, A., Valera, S., & Baratte, S. (2021). Exploration of chemosensory ionotropic receptors in cephalopods: the IR25 gene is expressed in the olfactory organs, suckers, and fins of Sepia officinalis. Chemical Senses, 46. https://doi.org/10.1093/chemse/bjab047
Audesirk, T. E. (1977). Chemoreception in Aplysia californica III. Evidence for pheromones influencing reproductive behavior [Article]. Behavioral Biology, 20(2), 235-243. https://doi.org/10.1016/S0091-6773(77)90799-4
Bansil, R., & Turner, B. S. (2018). The biology of mucus: Composition, synthesis and organization. Advanced Drug Delivery Reviews, 124, 3–15. https://doi.org/10.1016/j.addr.2017.09.023
Beckmann, A., & Özbek, S. (2012). The nematocyst: a molecular map of the cnidarian stinging organelle. The International journal of developmental biology, 56(6-8), 577–582. https://doi.org/10.1387/ijdb.113472ab
Boat, T. F., & Cheng, P. W. (1980). Biochemistry of airway mucus secretions. Federation proceedings, 39(13), 3067–3074. PMID: 7428951
Cannon, Q., & Wagner, E. (2003). Comparison of Discharge Mechanisms of Cnidarian Cnidae and Myxozoan Polar Capsules. Reviews in Fisheries Science, 11(3), 185–219. https://doi.org/10.1080/10641260390244305
Celichowski, J., & Grottel, K. (1993). Twitch/tetanus ratio and its relation to other properties of motor units. Neuroreport, 5(3), 201–204.
Chase, R., & Tolloczko, B. (1993). Tracing neural pathways in snail olfaction: From the tip of the tentacles to the brain and beyond [Article]. Microscopy Research and Technique, 24(3), 214-230. https://doi.org/10.1002/jemt.1070240303
Croll, R. P. (1983). Gastropod chemoreception. Biological Reviews, 58(2), 293-319. https://doi.org/10.1111/j.1469-185X.1983.tb00391.x
Croll, R. P., & Chase, R. (1977). A long-term memory for food odors in the Land snail, Achatina fulica [Article]. Behavioral Biology, 19(2), 261-268. https://doi.org/10.1016/S0091-6773(77)91554-1
Cummins, S. F., Nichols, A. E., Schein, C. H., & Nagle, G. T. (2006). Newly identified water-borne protein pheromones interact with attractin to stimulate mate attraction in Aplysia [Review]. Peptides, 27(3), 597-606. https://doi.org/10.1016/j.peptides.2005.08.026
David, C, N (1972). A quantitative method for maceration of hydra tissue: Springer-Verlag, 10 pages, page 260-268. A quantitative method for maceration of hydra tissue (springer.com)
Di Cosmo, A., & Winlow, W. (2014). Neuroecology and neuroethology in molluscs: The interface between behaviour and environment [Book]. https://www.scopus.com/inward/record.uri?eid=2-s2.0-84949747628&partnerID=40&md5=7263212f735415aeb996fa73fad06d4c
Edelstam, C., & Palmer, C. (1950). Homing behaviour in gastropodes. Oikos, 2(2), 259-270. https://doi.org/10.2307/3564796.
Emery, D. G. (1992). Fine structure of olfactory epithelia of gastropod molluscs [Article]. Microscopy Research and Technique, 22(4), 307-324. https://doi.org/10.1002/jemt.1070220402
Engel, U., Ozbek, S., Streitwolf-Engel, R., Petri, B., Lottspeich, F., & Holstein, T. W. (2002). Nowa, a novel protein with minicollagen Cys-rich domains, is involved in nematocyst formation in Hydra. Journal of cell science, 115(Pt 20), 3923–3934. https://doi.org/10.1242/jcs.00084
Feder, H. M. (1963). Gastropod defensive responses and their effectiveness in reducing predation by starfishes. Ecology, 44(3), 505-512. https://doi.org/10.2307/1932529
Feder, H. M., & Lasker, R. (1964). Partial purification of a substance from starfish tube feet which elicits escape responses in gastropod molluscs [Article]. Life Sciences, 3(9), 1047-1051. https://doi.org/10.1016/0024-3205(64)90118-3
Fouke, K. E., & Rhodes, H. J. (2020). Electrophysiological and Motor Responses to Chemosensory Stimuli in Isolated Cephalopod Arms. The Biological Bulletin, 238(1), 1–11. https://doi.org/10.1086/707837
Gilly, W. F., Renken, C., Rosenthal, J., & Kier, W. M. (2020). Specialization for rapid excitation in fast squid tentacle muscle involves action potentials absent in slow arm muscle. Journal of Experimental Biology, 223(3). https://doi.org/10.1242/jeb.218081
Godknecht, A., Tardent, P. (1988). Discharge and mode of action of the tentacular nematocysts of Anemonia sulcata (Anthozoa: Cnidaria). Marine Biology 100, 83–92 (1988). https://doi.org/10.1007/BF00392958
Graziadei, P. (1962, July 7). Receptors in the Suckers of Octopus. Nature. https://www.nature.com/articles/195057a0?error=cookies_not_supported&code=3b21c966-fd7c-4022-801c-44be0220dfd6
Imperadore, P., & Fiorito, G. (2018). Cephalopod tissue regeneration: Consolidating over a century of knowledge. Frontiers in Physiology, 9(593). https://doi.org/10.3389/fphys.2018.00593
9.6a: Interactions of skeletal muscles. (2020, August 13). Libretexts. Retrieved Novemeber 15, 2022, from https://med.libretexts.org/Bookshelves/Anatomy_and_Physiology/Book%3A_Anatomy_and_Physiology_(Boundless)/9%3A_Muscular_System/9.6%3A_Overview_of_the_Muscular_System/9.6A%3A_Interactions_of_Skeletal_Muscles
Karabulut, A., McClain, M., Rubinstein, B. et al. (2022). The architecture and operating mechanism of a cnidarian stinging organelle. Nat Commun 13, 3494. https://doi.org/10.1038/s41467-022-31090-0
Kass-Simon, G., & Pierobon, P. (2007). Cnidarian chemical neurotransmission, an updated overview. Comparative Biochemistry and Physiology Part A: Molecular &Amp; Integrative Physiology, 146(1), 9–25. https://doi.org/10.1016/j.cbpa.2006.09.008
Katsuki, T., & Greenspan, R. J. (2013). Jellyfish nervous systems. Current biology : CB, 23(14), R592–R594. https://doi.org/10.1016/j.cub.2013.03.057
Kier, W. M. (1982). The functional morphology of the musculature of squid (Loliginidae) arms and tentacles [Article]. Journal of Morphology, 172(2), 179-192. https://doi.org/10.1002/jmor.1051720205
Kier, W. M. (2016). The musculature of coleoid cephalopod arms and tentacles. Frontiers in Cell and Developmental Biology, 4(10). https://doi.org/10.3389/fcell.2016.00010
Kier, W. M., & Schachat, F. H. (1992). Biochemical comparison of fast- and slow-contracting squid muscle. Journal of Experimental Biology, 168(1), 41–56. https://doi.org/10.1242/jeb.168.1.41
Li, R., & DeMayo, F. J. (2018). Steroid receptors classical. Encyclopedia of Reproduction, 2, 142–157. https://doi.org/10.1016/b978-0-12-801238-3.64635-4
Lumen Learning (2022). Biology of Majors II. Characteristics of Phylum Cnidaria. 14-Module 11 Invertebrates. Lumen Learning. https://courses.lumenlearning.com/wm-biology2/chapter/characteristics-of-phylum-cnidaria/
Nüchter, T., Benoit, M., Engel, U., Ozbek, S., & Holstein, T. W. (2006). Nanosecond-scale kinetics of nematocyst discharge. Current biology : CB, 16(9), R316–R318. https://doi.org/10.1016/j.cub.2006.03.089
Östman, C. (2000). A guideline to nematocyst nomenclature and classification, and some notes on the systematic value of nematocysts. Scientia Marina. 64. 31-46. https://doi.org/10.3989/scimar.2000.64s13
Painter, S. D., Clough, B., Garden, R. W., Sweedler, J. V., & Nagle, G. T. (1998). Characterization of Aplysia attractin, the first water-borne peptide pheromone in invertebrates [Article]. Biological Bulletin, 194(2), 120-131. https://doi.org/10.2307/1543042
Pallasdies F, Goedeke S, Braun W, & Memmesheimer RM. From single neurons to behavior in the jellyfish Aurelia aurita. Elife. 2019 Dec 23. https://doi.org/10.7554/eLife.50084
Pierre, T. (1988), 17 – History and Current State of Knowledge Concerning Discharge of Cnidae, Editor(s): David A. Hessinger, Howard M. Lenhoff, The Biology of Nematocysts, Academic Press, 1988, Pages 309-332, ISBN 9780123453204, https://doi.org/10.1016/B978-0-12-345320-4.50022-5.
Rathmayer, W. & Beress, L. (1976). The effect of toxins from Ameonia sulcata (Coelenterata) on neuromuscular transmission and nerve action potentials in the crayfish (Astacus leptodactylus). J. Comp. Physiol. 109, 373–382. https://doi.org/10.1007/BF00663616
Satterlie RA. Do jellyfish have central nervous systems? J Exp Biol. 2011 April 15 ;214(Pt 8):1215-23. https://doi.org/10.1242/jeb.043687
Satterlie, R. A. (2015). Cnidarian Nerve Nets and Neuromuscular Efficiency. Integrative and Comparative Biology, 55(6), 1050–1057. https://doi.org/10.1093/icb/icv067
Simkin, D., & Bendahhou, S. (2011). Skeletal muscle NA+ channel disorders. Frontiers in Pharmacology, 2(63). https://doi.org/10.3389/fphar.2011.00063
Skaer, R. J., & Picken, L. E. R. (1965). The Structure of the Nematocyst Thread and the Geometry of Discharge in Corynactis viridis Allman. Philosophical Transactions of the Royal Society of London. Series B, Biological Sciences, 250(764), 131–164. http://www.jstor.org/stable/2416732
Smart, J. D. (2005). The basics and underlying mechanisms of mucoadhesion. Advanced Drug Delivery Reviews, 57(11), 1556–1568. https://doi.org/10.1016/j.addr.2005.07.001
Von Byern, J., & Klepal, W. (2006). Adhesive mechanisms in cephalopods: A Review. Biofouling, 22(5), 329–338. https://doi.org/10.1080/08927010600967840
Watson, G. M., & Mire-Thibodeaux, P. (1994). The cell biology of nematocysts. International review of cytology, 156, 275–300. https://doi.org/10.1016/s0074-7696(08)62256-1
Zheng, J., Li, D., Jiao, J., Duan, C., Gong, Y., Shi, H., Wang, Z., & Xiang, Y. (2021). Biomimetic recognition strategy for efficient capture and release of circulating tumor cells. Mikrochimica Acta, 188(6), 220–220. https://doi.org/10.1007/s00604-021-04856-4
Zwahlen, N. (2018). White Lipped Snail [Photograph]. inaturalist. https://www.inaturalist.org/photos/15443371?size=medium