A Biomechanical Review of Animal Tongue Functions
Shunyuan Xiao, Marc Srour, Sophie Allard
Abstract
The animal kingdom is characterized by an astonishing diversity in tongue morphologies, functions and mechanical abilities. Through evolution, different animal tongues have adapted to perform complex mechanical functions in prey-catching and feeding in order to ensure the survival of their species. Chameleons possess the ability to ballistically project their tongues toward fast-moving prey with great accuracy and acceleration due to elastic energy storage and release. Hummingbirds, who rely on nectar as their main food source, make use of their unique tongue shape, and physical concepts such as surface tension and elastic energy, to efficiently extract nectar from flowers. The saliva coating a frog's tongue, which can be modeled as a non-Newtonian fluid, greatly increases the efficiency of prey-catching by adhering strongly to fast-moving insects. Penguin tongues have evolved to be lined with papillae, allowing them to capture and consume slippery fish, their main food source. Not only do these mechanisms promote the survival of animal species, but they can also serve as useful inspirations for the design of biomimetic devices.
Introduction
In nature, the survival of a species depends on an animal's ability to find and consume food in their environment. While this looks different for every animal, this common goal dictates much of animal behavior and governs relationships between species in the animal kingdom. Many species possess interesting physical characteristics, which give them competitive advantages in catching prey and finding food. A prime example of this is how cheetahs use their long legs and low body weight to reach extraordinary speeds when running to catch prey. While it may seem less obvious, the tongue of an animal can provide great advantages in feeding and prey-catching. This complex muscular organ differs greatly in morphology and functions among animals; therefore, a body of research analyzing different animal tongue functions from a mechanical perspective is emerging.
Many animals possess particularly interesting tongue properties and functions. Chameleons possess an impressive ability to project their tongues to great distances in very little time, which gives them a useful advantage in prey-catching. Hummingbird tongues can efficiently extract nectar from flowers through shape changes. The saliva coating on a frog's tongue can be modeled as a non-Newtonian fluid, allowing for strong adhesion to prey during the tongue retraction process. Many species of penguins take advantage of the filiform papillae on their tongue surfaces to grip prey. These impressive evolutionary adaptations have allowed these species to continually compete with other animals in food search, and adapt to inevitable changes in their environments.
Ballistic projection mechanism in chameleon tongues
Morphology of the chameleon tongue
In order to properly analyze the ballistic tongue projection mechanism that allows chameleons to rapidly catch prey, the morphology of their tongues must first be understood. For the sake of this discussion, the chameleon tongue can be separated into three distinct elements, each playing an essential role in the projection mechanism: the entoglossal process, the accelerator muscle and the network of intralingual sheaths (Figure 1). The entire tongue complex rests on a central bone called the entoglossal process, which is mostly parallel-sided, except for the tapering at the anterior-most 1-1.5% of its length (Herrel et al., 2001).
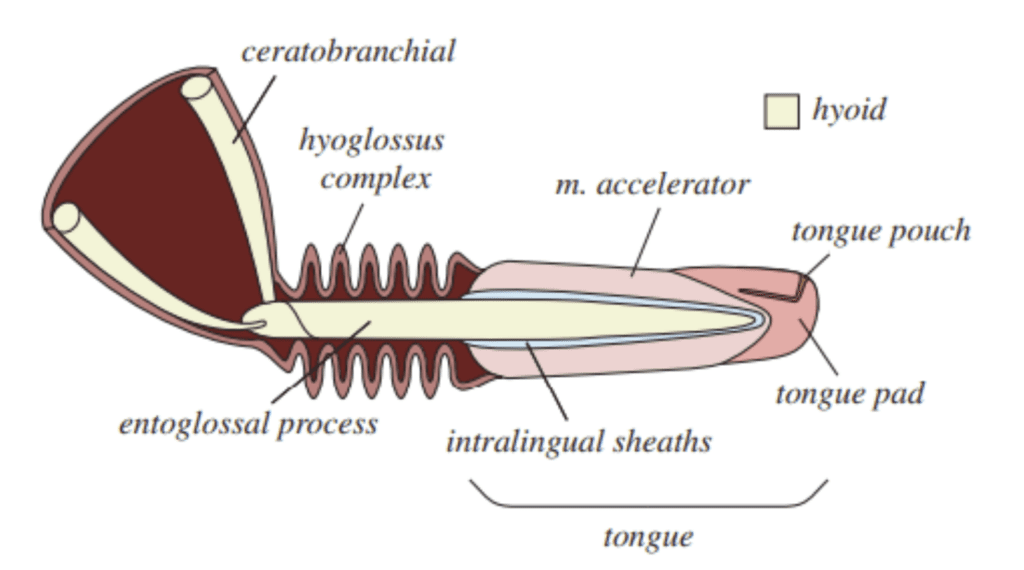
Fig. 1 Anatomical illustration of a chameleon tongue (Debray, 2011).
Attached to the entoglossal process are the intralingual sheaths. This region consists of collagenous tissue between the accelerator muscle and the bone, which was historically believed to only play a lubricating role in tongue projection (de Groot & van Leeuwen, 2004). However, recent research has revealed that these sheaths play a vital role in energy storage and release during projection (Anderson, 2016; Moulton et al., 2016). The intralingual sheaths are composed of helically arranged collagen fibers, organized in pairs of crossed fibers wrapping in opposite directions (Moulton et al., 2016). Within this region, successive sheaths are concentrically stacked, an arrangement which allows rapid telescopic extension of the tongue. Attached to the outermost sheath is the accelerator muscle, which takes the form of a thick cylindrical muscle (Moulton et al., 2016). Essentially, the tongue complex consists of the sheaths and the accelerator muscle, which rests on the central bone (Figure 2).
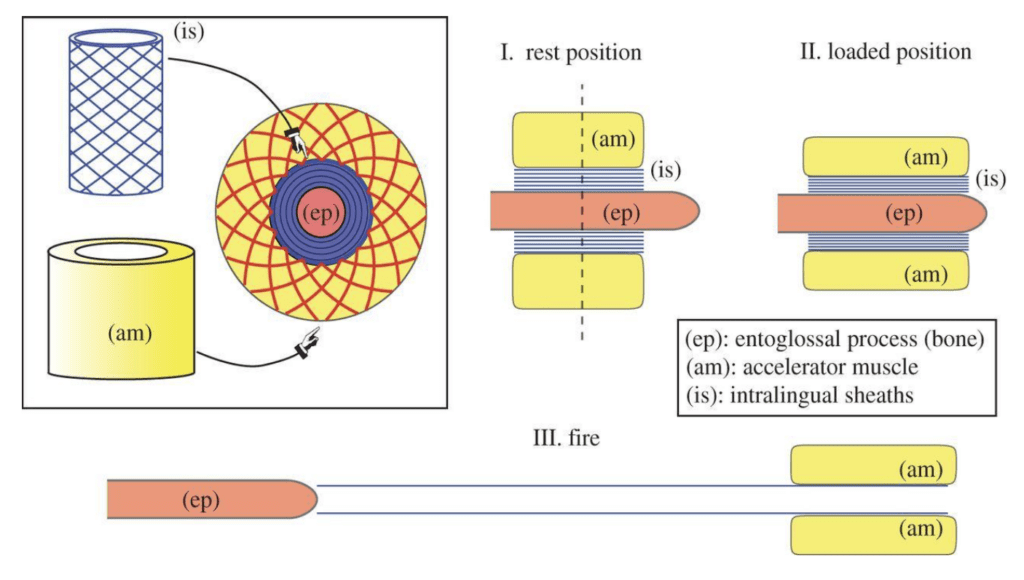
Fig. 2 Overview of the structure of a chameleon tongue and the 3 phases of tongue projection (Moulton et al., 2016).
Elastic energy storage in the loading phase of projection
Chameleons have long been a source of fascination for biologists due to their distinctive features, color-changing abilities and impressive prey-catching abilities. Much like those of salamanders and frogs, chameleon tongues perform ballistic projection, stretching up to 2.5 times their body length at high speeds to catch prey (Anderson, 2016). While it was previously believed that this projection was powered entirely by muscle activation, the peak power density measured during tongue extension can take values between 3000 and 14 040 W/kg, suggesting additional energy is being supplied to the system (Moulton et al., 2016). In this section, mechanisms of energy storage in the chameleon tongue complex and their importance in the projection mechanism will be discussed.
During the first phase of projection, commonly referred to as loading, muscle contraction occurs, and elastic energy is stored. The accelerator muscle contracts, performing mechanical work to extend along the entoglossal process, decreasing in diameter to compensate for the increase in length (de Groot & van Leeuwen, 2004). During this initial extension of the tongue complex to reach the tip of the bone, the helical collagen fibers of the sheaths provide significant resistance to muscle lengthening. As these fibers are stretched along the entoglossal process, tensile strain energy is being stored for later use (de Groot & van Leeuwen, 2004). In a numerical comparison of the extension and energy storage in a Jackson's horned chameleon tongue versus in an accelerator muscle with no sheaths, it is clear that in the absence of sheaths, the complex extends much farther in the loading phase and stores little elastic energy (Figure 3) (Moulton et al., 2016). Elastic potential energy would therefore not be stored in the complex for conversion to kinetic energy and the acceleration of the tongue during extension would be significantly reduced. This conclusion highlights the importance of energy storage in the intralingual sheaths for ballistic tongue projection. In fact, 77% of power during projection can be attributed to energy released by these elastic tissues (de Groot & van Leeuwen, 2004).
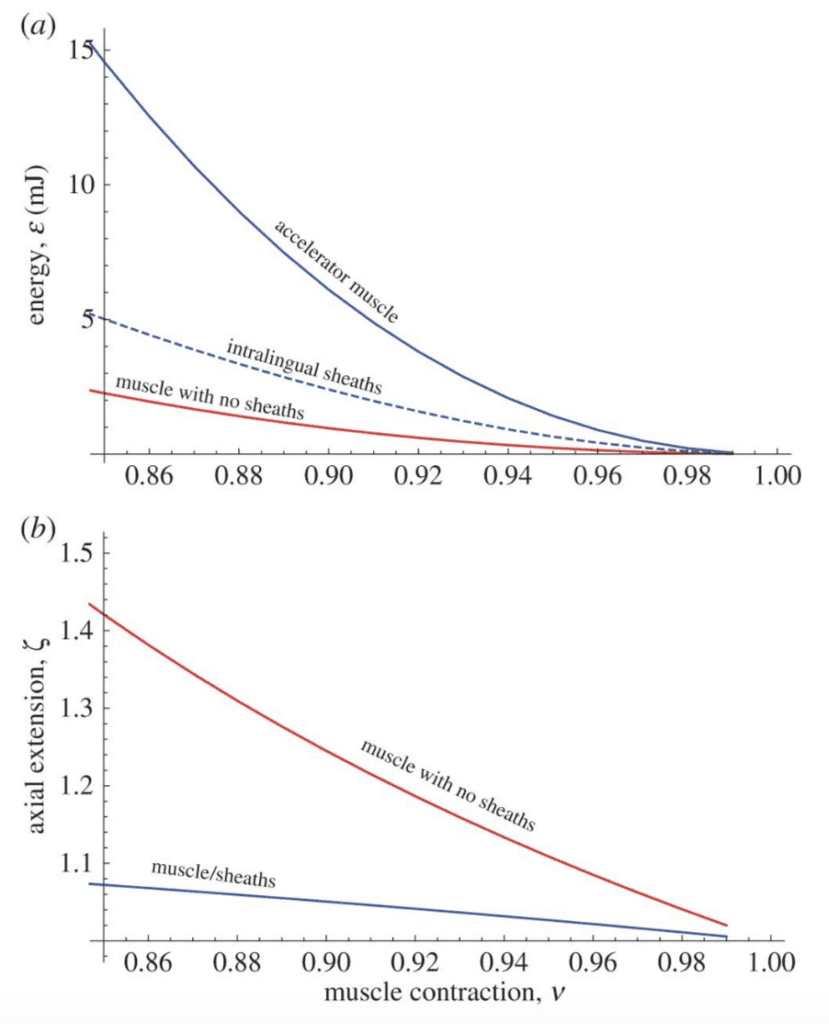
Fig. 3 Elastic energy storage and axial extension of chameleon tongues as a function of muscle contraction, with and without the presence of intralingual sheaths (Moulton et al., 2016).
Dynamics of tongue extension
Once elastic energy has been stored in the collagenous fibers of the tongue, a firing mechanism allows its quick conversion into kinetic energy and produces the large accelerations that chameleon tongues are known for. A model of the dynamics of the system can be used to explain this phenomenon (Figure 4).
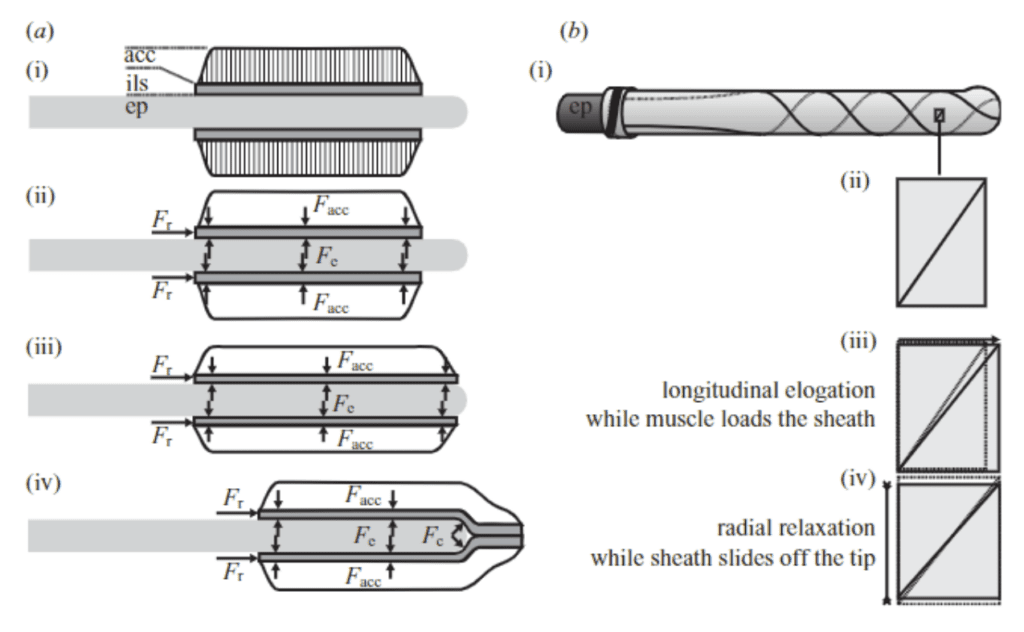
Fig. 4 a) Forces acting on the tongue complex in the loading phase, b) Representation of sheath elongation and relaxation during loading and firing (de Groot & van Leeuwen, 2004).
During loading, action and reaction forces are at play on the tongue complex as mechanical work is done to extend it along the entoglossal process. During compression, the accelerator muscle exerts a force (Facc) on the sheath network, and thus a reaction force (Fe) is exerted by the bone in the opposite direction. These force pairs initially have no acceleration effect, as the system is in equilibrium; however, once the tongue complex reaches the outermost tip of the entoglossal process, a component of Fe in the axial direction is present, thereby causing acceleration of the complex off the bone (de Groot & van Leeuwen, 2004). As such, the tip of the entoglossal process is essentially a “launch pad to convert the stored energy into kinetic energy” (Moulton et al., 2016).
As the sheaths slide off the bone, their internal radii are free to decrease, thus reducing the tensile strain on the collagenous fibers. The tensile strain energy that was stored in these fibers during the loading phase is converted into kinetic energy as the tongue complex is accelerated forward by the reaction force at the tip of the bone (Moulton et al., 2016). However, it is worth noting that not all stored elastic energy is used to accelerate the system. The mathematical model for tongue projection revealed a nonlinear relationship between stored energy and final kinetic energy of the complex (Moulton et al., 2016). This loss of energy can be attributed to the inevitable deformations of the soft tissues in the tongue complex throughout the projection process.
Once the launch process is complete and the tongue complex has left the bone, there are no longer any forces at play on the system. Momentum alone is responsible for carrying the tongue pad and pouch to the targeted prey (Anderson, 2016). Chameleons can accelerate their tongues at rates between 400 and 2590 m/s², depending on species (Moulton et al., 2016). This ballistic tongue projection is truly a remarkable application of mechanics in the animal kingdom and can serve as a model for various engineering designs requiring large accelerations achieved through elastic energy storage.
Factors affecting chameleon tongue projection
In many animals, movements driven by muscular contraction are significantly impacted by the temperature of their environment, muscle contraction in colder temperatures being much slower. As such, studies investigating the thermal dependence of chameleon tongue projection have emerged. In a study of veiled chameleon tongue projection, no significant changes in projection performance were observed over a temperature range of 15-35°C (Anderson & Deban, 2010). More specifically, there was no significant decrease in projection distance and while peak power and accelerations increased slightly at higher temperatures, the increase was not large enough to suggest a significant thermal dependence of the mechanism. These findings allow researchers to draw the interesting conclusion that muscular performance driven by elastic recoil, such as chameleon tongue projection, is thermally robust compared to movements driven by muscular contraction (Anderson & Deban, 2010; Anderson & Deban, 2012). This conclusion demonstrates a chameleon's ability to adapt to its environment and continue to compete for prey during all seasons. To further illustrate this point, an interesting comparison can be made between chameleon tongue projection and retraction, which is powered purely by muscle contraction, at various temperatures (Figure 5). The graphs in Figure 5 show that peak velocity, acceleration and power of the chameleon tongue during projection (green) are much less temperature dependent than during retraction (yellow), as illustrated by the steeper yellow slope.
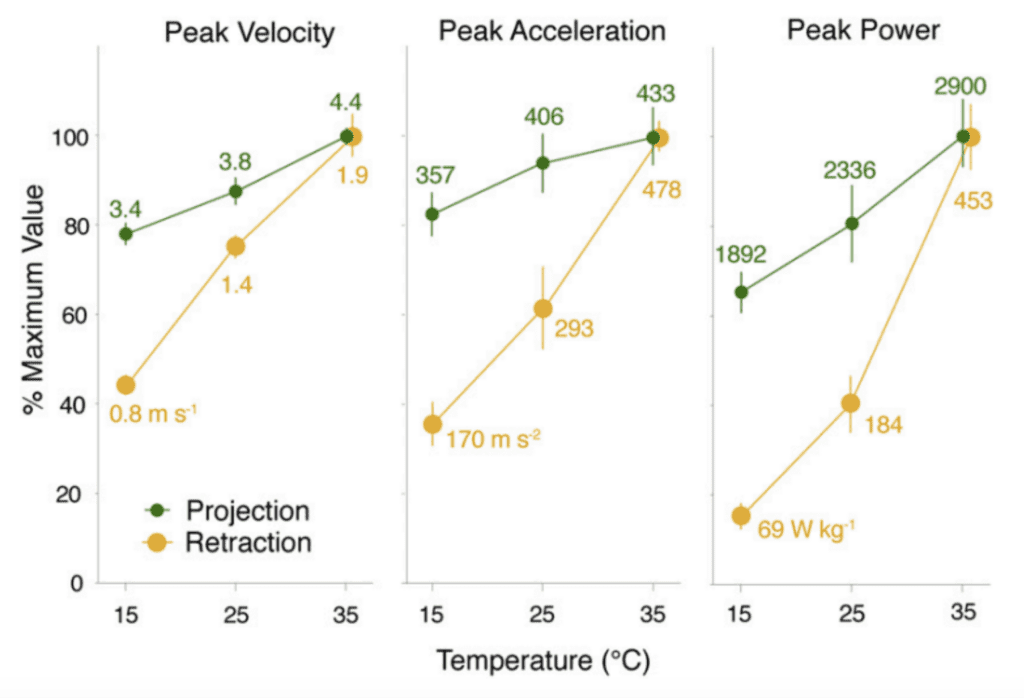
Fig. 5 Comparison of peak velocity, acceleration and power of the chameleon tongue complex during projection (green) and retraction (yellow) at different temperatures (Anderson & Deban, 2010).
In nature, there exist many different chameleon species characterized by drastically different sizes. As such, an interesting branch of chameleon tongue research focuses on investigating the role of chameleon size on projection dynamics. A study on 20 chameleon species revealed that smaller chameleons exhibit greater performance, projecting to proportionally larger distances with greater accelerations and power (Figure 6) (Anderson, 2016).
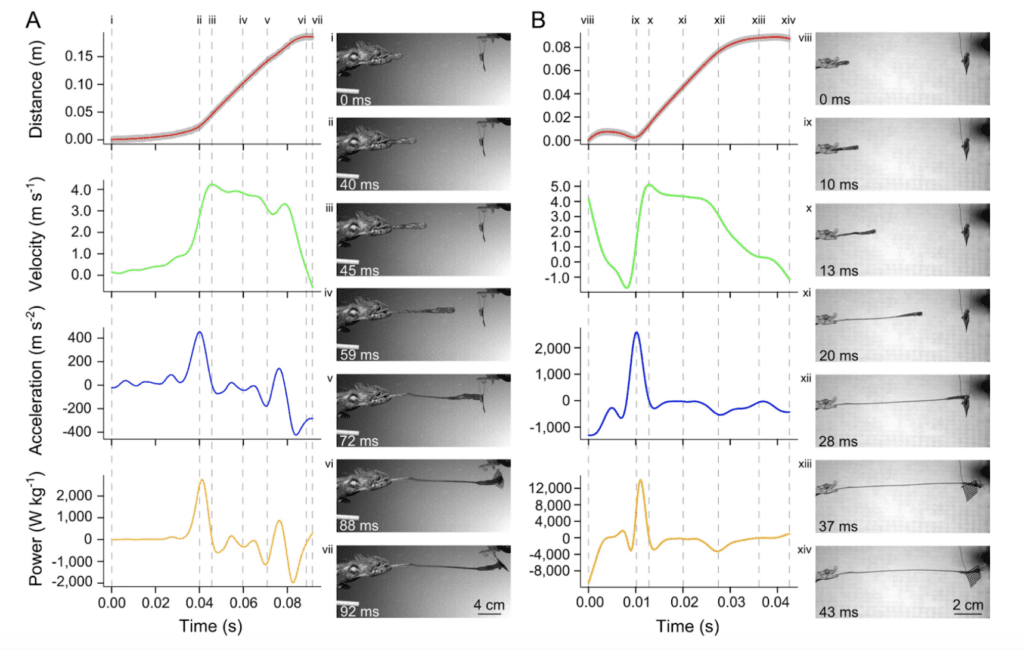
Fig. 6 Visual comparison of kinematic and dynamic profiles of projection for two chameleon species: (A) a 198mm SVL (snout-vent length) Furcifer oustaleti and (B) a 47mm SVL Rhampholeon spinosus (Anderson, 2016).
This result can be explained by the proportionally larger tongue apparatuses present in smaller chameleon species. Based on principles of geometric similarity, these proportionally larger tongue apparatuses can produce greater mass-specific power and greater accelerations (Anderson, 2016). This property suggests that smaller chameleon species have evolved to increase their prey-catching effectiveness through proportionally larger tongues and jaws, in order to compete with larger species for survival.
Biomechanics of nectar extraction in hummingbirds
Hummingbird tongues serve as efficient tools to extract nectar from various kinds of flowers, which is their main food source. While the beak of a hummingbird is imperative in the process of accessing the nectar, a hummingbird's tongue is primarily responsible for nectar extraction. While it was previously believed that hummingbird tongues extract nectar through a process of capillary rise, recent studies have found flaws in this theory. This contradiction arose from the fact that gravity does not increase the amount of nectar ingested from downward facing flowers, like the capillary model would suggest (Rico-Guevara & Rubega, 2011). Instead, the hummingbird tongue can be modeled as a fluid trap, which changes shape and stores elastic energy in order to efficiently extract nectar from a flower (Rico-Guevara & Rubega, 2011). A biomechanical model for this process will be discussed below.
Anatomy of a hummingbird tongue
A hummingbird's ability to efficiently extract nectar from a flower is largely due to the unique structure of its tongue (Figure 7). The distal portion of the tongue splits into two identical grooves, each contributing equally to feeding (Rico-Guevara & Rubega, 2011). Each groove is centered by a supporting rod and lined by lamellae, which are fringed, hair-like structures. These structures are lined longitudinally along the supporting rod at the distal portion of the tongue (Rico-Guevara & Rubega, 2011). Both the rods and the lamellae can change their relative position during protrusion and retraction (Rico-Guevara & Rubega, 2011). Functionally, the orientation of rods can drive the lamellae to change their shape when immersed in nectar, which is crucial to the nectar extraction mechanism.
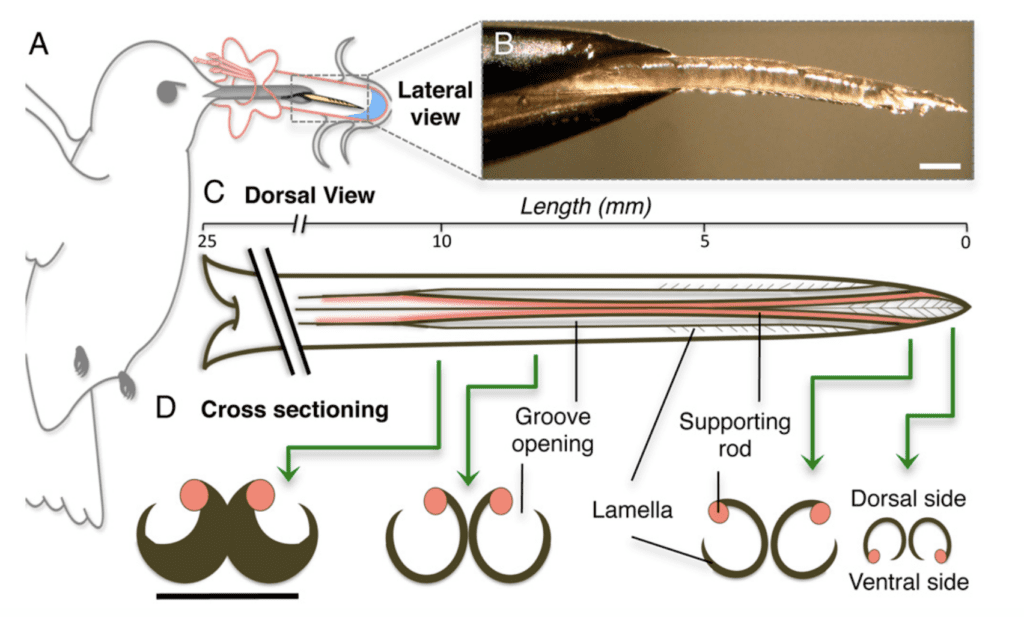
Fig. 7 Anatomy of hummingbird's tongue (Rico-Guevara, 2011).
When the hummingbird's bill tips are closed, the grooves are not yet bifurcated. For the sake of the analysis of energy change during feeding, this is called the resting position (Rico-Guevara & Rubega, 2011). When a hummingbird is ready to begin the extraction process, the bill squeezes itself and pumps out the tongue towards the target flower. The tongue of a hummingbird can reach a maximum of 2 times the length of the bill which contributes to the efficiency of feeding (Rico-Guevara, 2017). During tongue projection, the tips of the grooves remain closed until they reach the surface of the nectar. Once in contact with the nectar, the grooves separate into their bifurcated position, creating a large contact surface area for the nectar collection. The contact with nectar also initiates the expansion of the lamellae, which move to an unfurled position (Rico-Guevara, 2017). When retracting the tongue from the flower, the rods rotate in order to retrieve the expanded lamellae, which return to their original furled position, and store nectar in the spaces between the supporting rod and the fringes. Once the tongue is completely withdrawn from the flower, the tip of each lamella is completely closed to prevent the nectar from leaking during the movement of the tongue back into the bill. This is especially important during the high-frequency repetition of the licking cycle to avoid any unnecessary nectar loss (Rico-Guevara & Rubega, 2011). The protrusion and retraction of the tongue repeats as a cycle, allowing the hummingbird to transport nectar from the flower into the bill with high efficiency, while minimizing nectar loss.
Roles of the lamellae and the supporting rods in nectar extraction
The different components of a hummingbird tongue have specialized roles in the nectar extraction process. As mentioned above, the lamellae are membrane-like structures lined longitudinally along the supporting rod at the distal portion of the tongue. A lamella itself has no muscle to function; therefore, the only strength it can provide is the elastic tension created by the orientation of the supporting rod (Rico-Guevara & Rubega, 2011). This tension allows the lamellae to change shape to either acquire nectar when immersed in a flower or store nectar during the retraction phase. The tip of the tongue contacts the nectar surface with closed lamellae, which then unfurl to let nectar fill the tongue. The purple wall-like structure in Figure 8 shows the shape of the lamellae when unfurling, since salvaged samples are naturally unfurled (Rico-Guevara & Rubega, 2011).
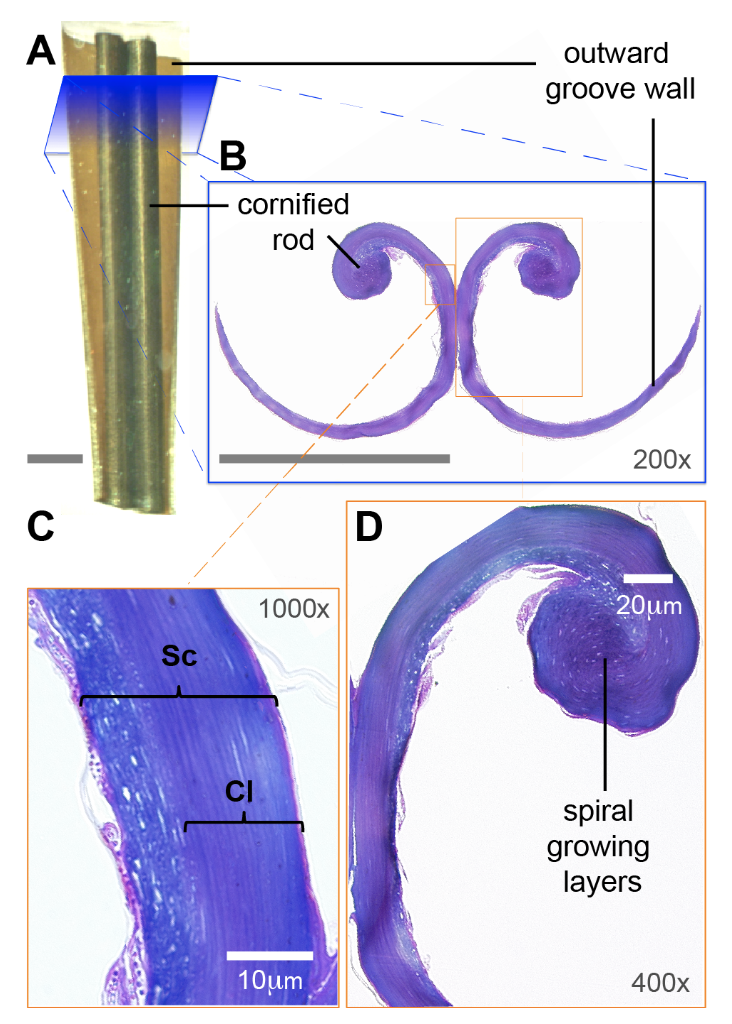
Fig. 8 The microstructure of the grooves of a hummingbird tongue includes the curled lamella (purple spiral layer) and the supporting rod (large round purple structure). a) Illustration of the tongue. b) Magnified illustration of tongue cross section showing the configuration of the grooves. c) Microscopic view of groove walls (Sc = stratum corneum and Cl = cornified layer). d) Spiral layers in the cornified rod (Rico-Guevara, 2017).
The supporting rods rotate while protruding the nectar surface, and the lamellae expand, resulting in the flow of nectar into the tongue. The lamellae will remain open until the tongue is fully retracted into the bill due to the absence of muscle to do work to close them. Since the lamellae cannot close spontaneously due to the absence of muscle, there must be external forces acting on them to cause the shape change. Figure 9 demonstrates the forces acting on the lamellae (Rico-Guevara, 2017).
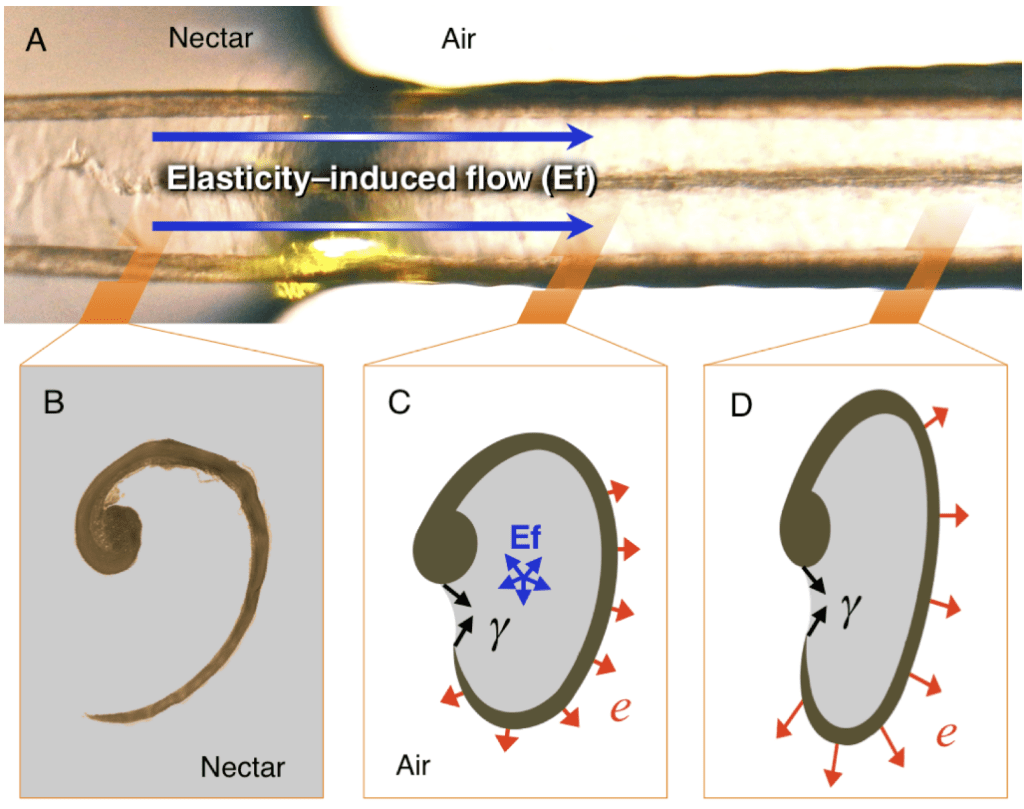
Fig. 9 The forces applied to grooves (Ef: expansion force, Y: surface tension created by nectar, e: expansion effect caused by the expansive force) (Rico-Guevara, 2017).
Physical properties, such as surface tension, Laplace pressure and elastic properties, play an important role in the nectar extraction mechanism (Rico-Guevara & Rubega, 2011). In order to keep the grooves in a flattened configuration during protrusion, the thin layer of nectar collected in the lamellae acts as an adhesive (Rico-Guevara, 2017). The flattened shape of the groove plays a key role in keeping the nectar inside the lamellae (Rico-Guevara, 2017). The nectar in the flower keeps flowing into the tube-shaped grooves creating a jet of fluid. The thick nectar flow induces great elastic potential in the outwards direction, which causes lamellae to unfurl due to the expansive adhesion (Rico-Guevara, 2017). However, the strong surface tension of nectar also applies a cohesive force at the top of the lamellae to keep them in an open configuration, preventing nectar leakage. This surface tension also adheres nectar tightly to the wall of lamellae to prevent the infiltration of bubbles (Rico-Guevara, 2017). The expansion, then, destabilizes the equilibrium position of the lamellae and causes the area outside nectar to expand, which allows more nectar to flow into grooves. This elasticity-induced flow creates a positive feedback loop by extracting more and more nectar from the flower while the surface tension created by the nectar prevents this flow from leaking (Rico-Guevara, 2017).
When the lamellae are withdrawn from nectar, the air outside the lamellae applies Laplace pressure on them to keep the meniscus opening held tightly to prevent it from leaking nectar (Rico-Guevara, 2011). In Figure 10, Rico-Guevara isolates two states of the lamellae based on the surface area of the meniscus opening (Rico-Guevara, 2011). The elastic energy stored in lamellae holds their fundamental shape. Surface tension created by the thick layer of nectar collected inside the lamellae (Yi) reduces the meniscus width whether the lamellae are in nectar or in the air (Rico-Guevara, 2011). When the lamellae are submerged in the nectar, the surrounding nectar also creates surface tension in the opposite direction, allowing the meniscus width to remain large enough for more nectar to flow into the lamellae (Rico-Guevara, 2011). Once the lamellae are extracted from nectar, they are in contact with the air, a much less dense medium, so the surface tension it engenders is considered negligible (Rico-Guevara, 2011). Yet because of the significant pressure differences between air and nectar, Laplace pressure plays the key role of holding the meniscus opening tightly to prevent nectar from leaking (Rico-Guevara, 2011).
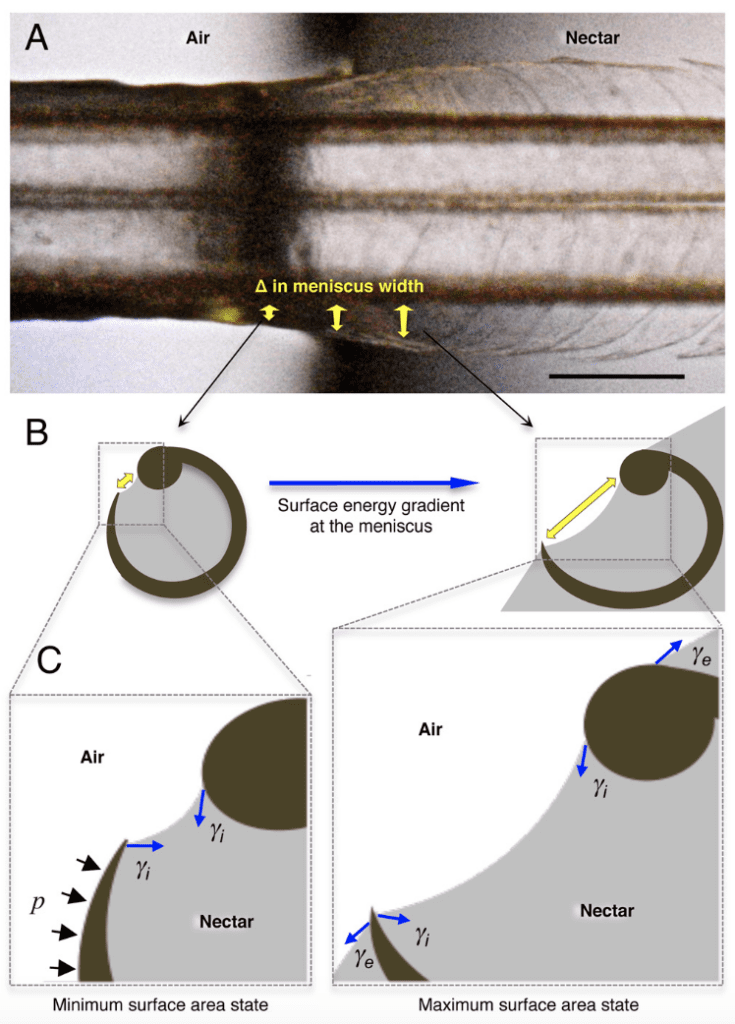
Fig. 10 Illustration of the forces applied to grooves. The yellow arrow represents the meniscus width at the opening of the lamellae, the blue arrow (Yi) represents the surface tension applied by the surrounding nectar and the black arrows (p) represent the Laplace pressure from the air (Rico-Guevara, 2017).
Shape changes of the tongue during feeding
Muscle function is observed during the protrusion phase of the tongue (Rico-Guevara et al., 2019). In Rico-Guevara's studies, the fluid motion requires no muscle participation, and is instead passively driven by the morphological design of the tongue (Rico-Guevara et al., 2019). Before the protrusion, the tongue rests in the bills with no muscle participation. Once the tongue is pumped out by the squeeze of the muscle of the bill, the two groove tips spread. Since the projection of the spiral rods leads to self-orientation, the lamellae also spread in the air, which prepares them for contact with the nectar surface (Rico-Guevara et al., 2019). Figure 11 shows the cycle of muscular and non-muscle-driven actions. While retracting the tongue, the elastic energy has not yet been restored. The round shape of the dorsal view of the lamellae means that there is a larger surface area outside the nectar. This not only creates the positive feedback of nectar licking, but also creates a larger storage inside the groove, which allows hummingbirds to acquire more nectar in one licking cycle (Rico-Guevara, 2017). This advantage allows them to survive in environments where food cannot be easily acquired.
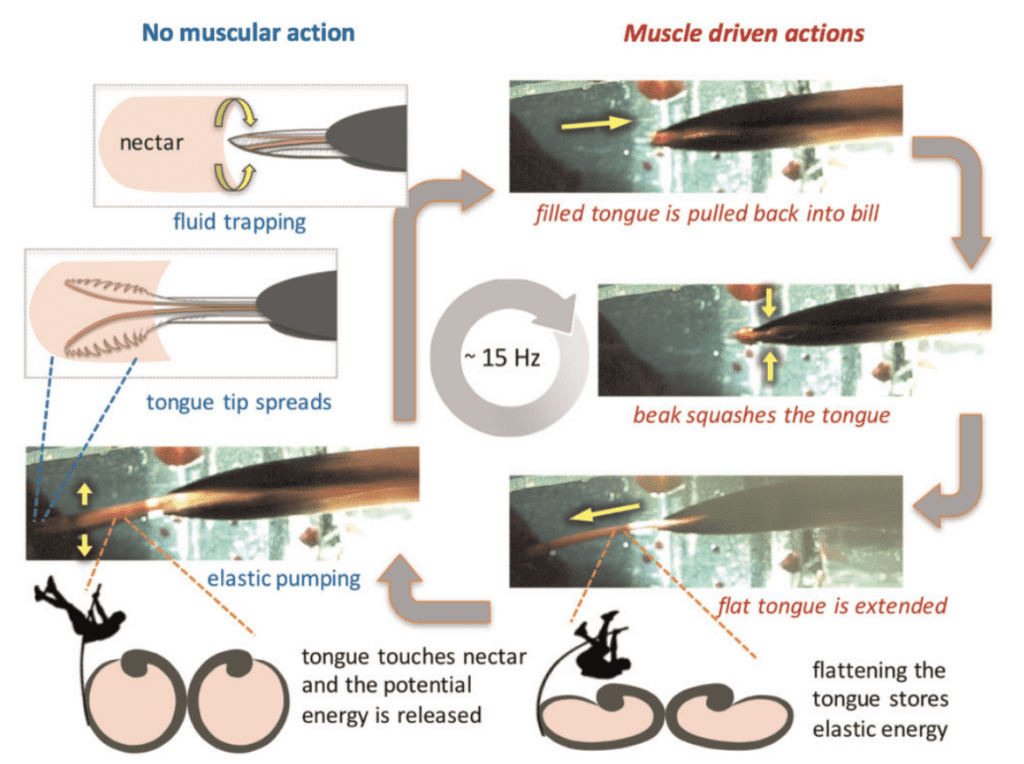
Fig. 11 Muscle function of a licking cycle. Images on the left side show the actions with no muscle participation, when potential energy is released. Images on the right-hand side show actions involving muscle participation, where potential energy is stored (Rico-Guevara et al., 2019).
Prey-gripping mechanisms in the animal kingdom
The frog's saliva: a non-Newtonian fluid
In order to properly understand how a frog's saliva is useful in prey-catching, the concepts of Newtonian and non-Newtonian fluids must be defined. A Newtonian fluid is one that follows Newton's law of viscosity (George & Qureshi, 2013). The law relates the shear stress applied to a fluid and the shear rate of deformation of that fluid (Equation. 1). The constant relating these two quantities is known as the viscosity, and it is constant for a given temperature and pressure (George & Qureshi, 2013). In other words, the viscosity of a Newtonian fluid will remain constant regardless of the shear stress applied to it.
\tau=\mu \gamma
Eq. 1 Newton's Law of viscosity (where is shear stress, is the shear rate and is the viscosity) (Franco & Partal, 2010).
In contrast, a non-Newtonian fluid does not follow this law (Galdi et. al 1993). Hence, its viscosity changes with the amount of mechanical disturbance applied to it (Figure 12). There exist two types of non-Newtonian fluids: shear-thickening (dilatant) liquids, whose viscosity increases with the rate of shear strain (for instance quicksand), and shear-thinning fluids, whose viscosity decreases with the rate of shear strain (like ketchup, paint or blood) (Galdi et. al 1993).
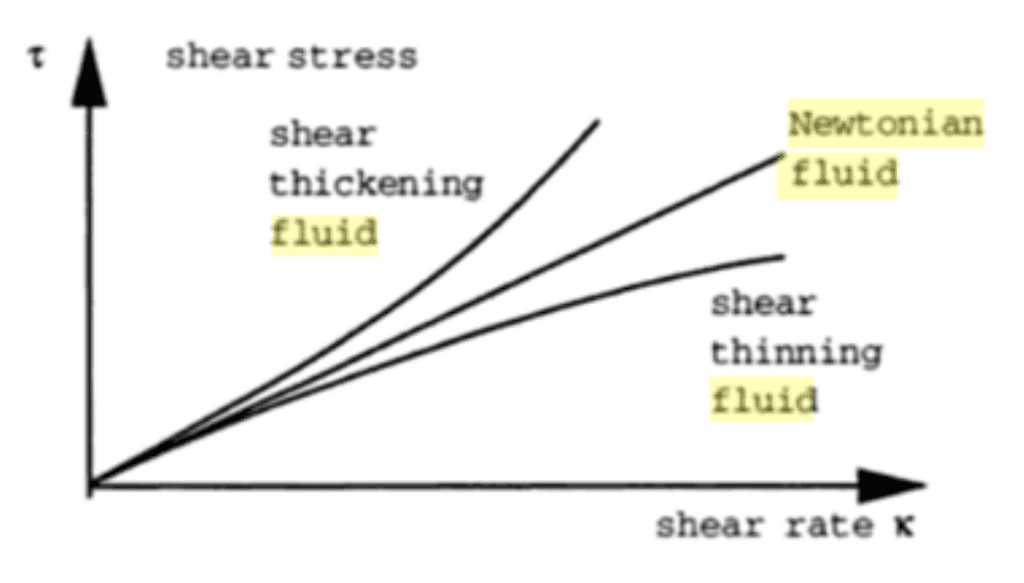
Fig. 12 Viscosity of Newtonian and non-Newtonian fluids (Galdi et. al 1993).
Given an understanding of non-Newtonian fluids, their role on a frog's tongue for prey-catching can be discussed. Frogs are known throughout the animal kingdom for the fast and effective use of their tongues to catch prey. There are over 4000 species of frogs and toads which utilize their tongue to grab prey at impressive speeds and with strong adhesion (Noel et al., 2017). Furthermore, a frog's prey is often agile and can weigh up to 1.4 times its body weight, increasing the need for strong adhesion between the tongue and the prey (Noel et al., 2017).
In order to cope with that, frogs make use of a non-Newtonian fluid: their saliva. The frog's saliva is a shear-thinning fluid which means that, as explained previously, when no pressure is applied to it, it is relatively viscous and thick (Noel et al., 2017). However, when shear stress is applied to it, the viscosity of the saliva changes, allowing it to be waterier. There are many different chemical compounds that make up the composition of saliva, notably electrolytes, proteins, enzymes and mucins (Noel & Hu, 2018a). The mucins, which are long-chain glycoproteins with high molecular weight, are mostly responsible for the fibrosity and viscosity of the liquid, allowing long threads of saliva to be stretched without breaking (Noel & Hu, 2018a).
To measure the shear viscosity of the saliva on a frog's tongue, an experiment was conducted where a sample was placed inside a cone-plate rheometer, and the viscosity was measured at shear rates over a large range of values (Figure 13). This particular study investigated the shear viscosity of 3 types of saliva, but the focus will be on the frog's (Noel & Hu, 2018a).
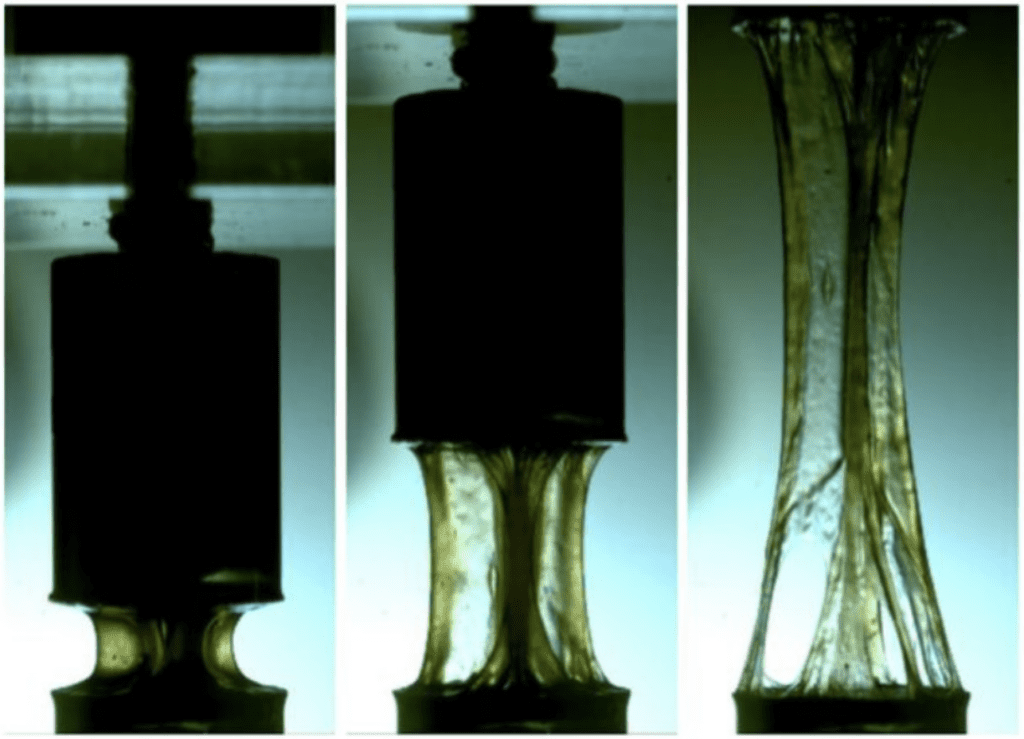
Fig. 13 Stretched frog saliva inside a rheometer (Noel & Hu, 2018a).
Data was collected from this experiment to then draw a graph of the shear viscosity in relation to the shear stress (Figure14). The graph can be interpreted as two distinct plateaus: one at 70 Pa s at low shear rates and one at 0.7 Pa s at high shear rates (Noel & Hu, 2018a). The duality of this saliva viscosity confirmed by the measurements is the key to the capture and release of the frog's prey: viscous saliva attaches the tongue firmly to prey, while watery saliva lets the insect slide off the tongue.
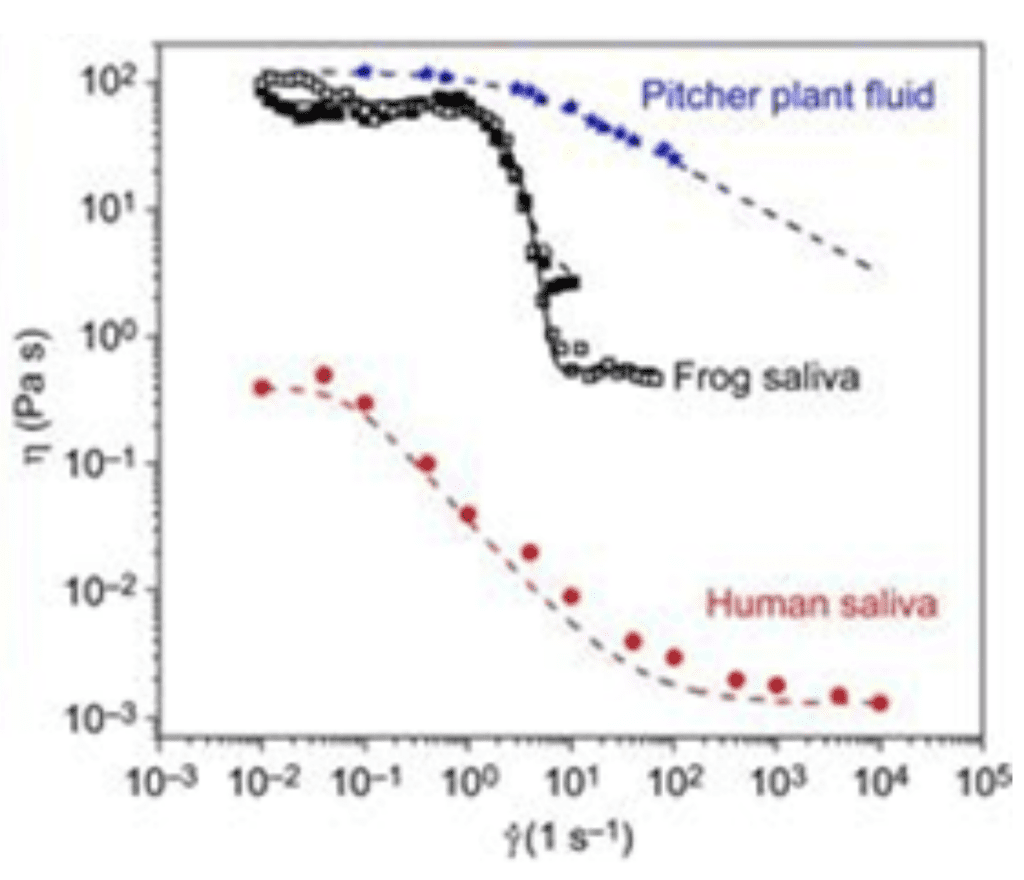
Fig. 14 Shear viscosity η of human saliva (red circles), frog saliva (open and filled black squares) and pitcher plant fluid (blue stars) in relation to shear stress (Noel & Hu, 2018a).
In addition, the tongue must be as adhesive as possible in order to prevent the prey from sliding off during the high-speed retraction into the frog's mouth. During the projection of the tongue, the speed at which the tongue travels creates great shear stress, resulting in a thinning of the saliva before contact with the insect (Noel & Hu, 2018a). This watery saliva is now able to penetrate all of the insect's crevices, filling as much space as possible. This phenomenon greatly increases the surface of contact between the insect and the tongue, allowing for a much stronger adhesion (Noel & Hu, 2018a).
Some species of frogs have been recorded to have tongue impact speeds of up to 4000 mms-1(Noel et al., 2017). This speed is more than necessary to create enough shear stress to make the frog saliva viscosity drop. High-speed impact does not only overcome the insect's reaction time, but it makes use of the saliva's non-newtonian shear-thinning properties to increase tongue adhesion (Noel et al., 2017).
However, even once prey-tongue adhesion is complete, the most challenging part of catching prey is keeping it on the tongue during retraction into the mouth. As shown above, saliva acts as a viscous substance once no shear stress is applied to it. Hence, when the shear stress initially present due to the rapid extension of the tongue wears off, the saliva, now filling all the insect's pores, acts as an extremely strong adhesive due to its increased viscosity (Noel et al., 2017). Thus, when the frog retracts its tongue, the prey can hardly escape, resulting in a very effective catching mechanism.
However, once the prey has reached the frog's mouth, the saliva must be runny again in order for the frog to be able to swallow its meal. Frogs have been observed to retract their eyeballs to push food down their throat (Figure 15) (Levine et al., 2004). The motion provides a shearing force allowing saliva to be runny again, so the frog is able to easily swallow the prey.
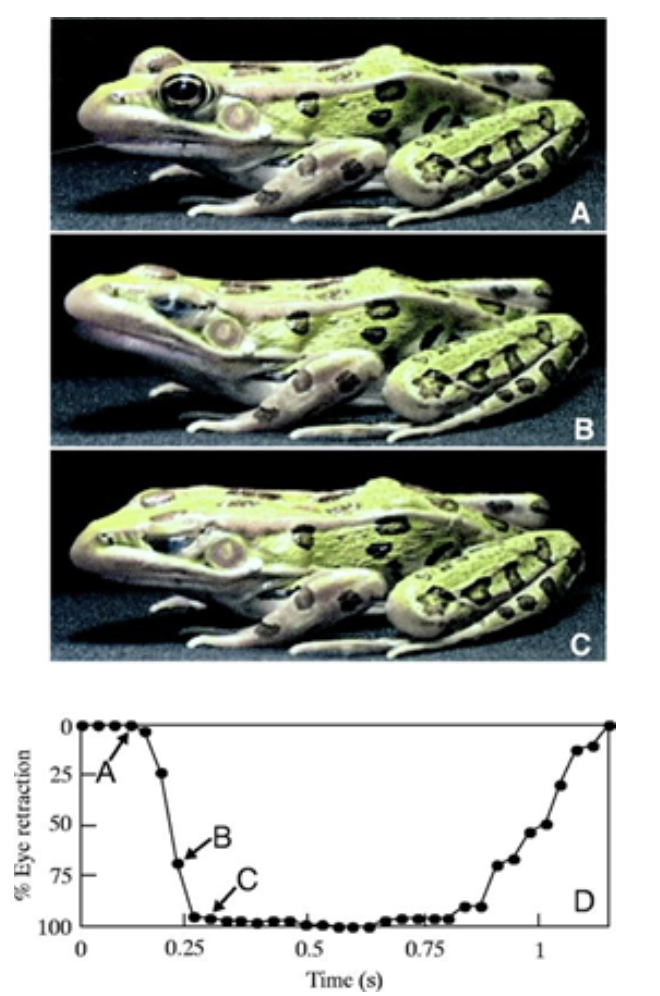
Fig. 15 Frog eye retraction during swallowing (Levine et al., 2004).
Uses of papillae in animals
In order to properly feed themselves in nature, animals often encounter food that is slippery, furry, or hard to reach. Such problems can often be overcome by making use of tongue surface features, such as papillae and saliva (Noel & Hu, 2018a). Interactions between the tongue and its target are dependent on the epithelial tissue found at the tongue's surface. In some animals, this epithelial surface is covered in keratin microstructures known as papillae (Figure 16) (Noel & Hu, 2018a).
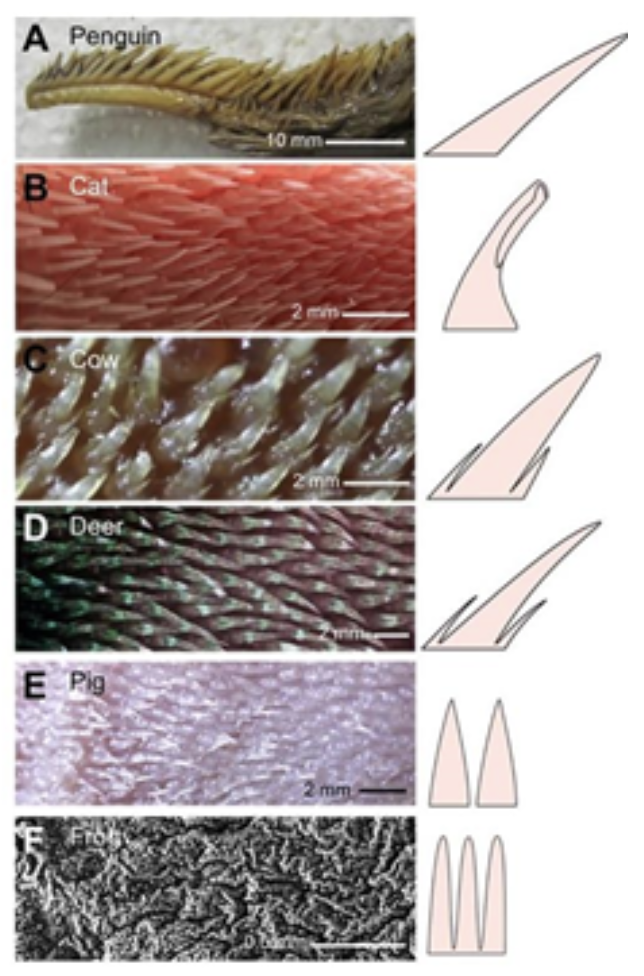
Fig. 16 Papillae photographs (left) and schematics (right) of (A) nestling penguin Aptenodytes fosteri, (B) domestic cat Felis catus, (C) cow Bos taurus, (D) deer Odocoileus virginianus, (E) pig Sus domesticus and (F) frog Lithobates catesbeianus. (Noel & Hu, 2018a).
In the animal kingdom, there are two dominant forms of papillae: fungiform and filiform. Fungiform papillae often take the shape of mushrooms, thus explaining their name. These structures are responsible for taste and contain nerve endings and taste buds (Noel & Hu, 2018a). However, fungiform papillae have no use regarding prey gripping. On the other hand, filiform papillae are cone-shaped structures that contain no taste buds and are imperative in helping animals grip food (Noel & Hu, 2018a).
The largest filiform papillae are found in birds, however they are also fairly abundant in felines (Noel & Hu, 2018a). In fact, the tongues of some penguins, geese and flamingos have papillae with length up to 1cm long to aid in pushing relatively slippery food down their throats (Noel & Hu, 2018a). Aptenodytes fosteri (emperor penguin) serves as an excellent example of the use of filiform papillae for prey gripping (Noel & Hu, 2018a). In order to survive in the wild, an emperor penguin must be able to effectively catch fish and maintain grip on the slippery prey between its jaws while swimming at high velocities (Figure 17).
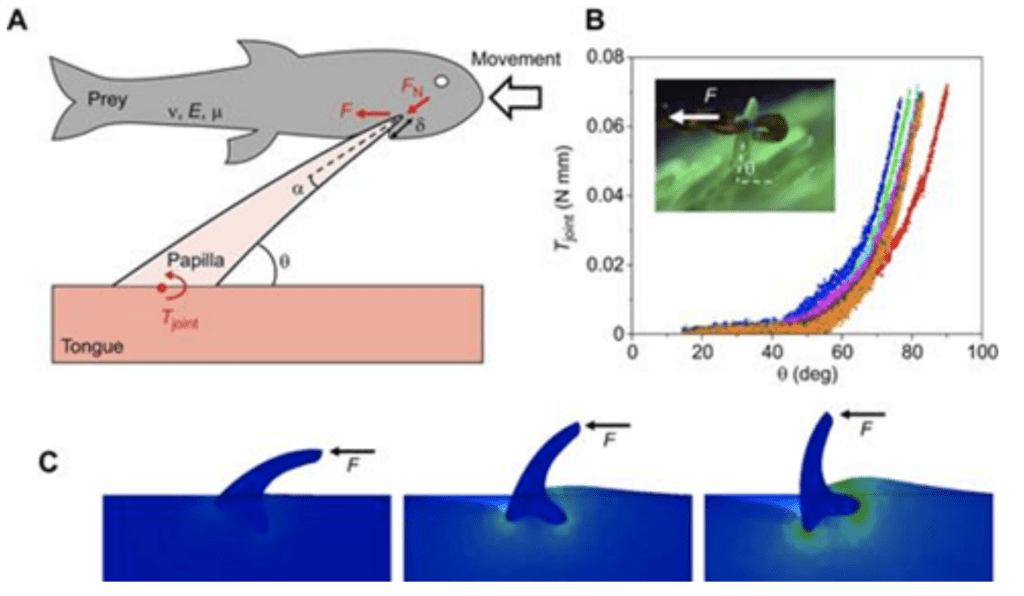
Fig. 17 (A) Schematic of a penguin papilla interacting with a fish, B) Graph showing resistive torque Tjoint of a cat papilla, C) Horizontal force applied on a rigid cat papilla (Noel & Hu, 2018a).
Sharp and rigid papillae enhance grip through their angled orientation (Noel & Hu, 2018a). In fact, the backward-positioning of the hundreds of papillae work like a one-way valve, allowing food to slide into but not out of the mouth. When a fish enters the penguin's mouth, the papillae pivot and slide out of their insertion point; however, when the prey tries to escape by moving out of the mouth, the papillae will be driven inversely and will be further inserted into the flesh of the prey (Figure 16A) (Noel & Hu, 2018a). Connective tissue at the papillae's base creates the resistance in rotation, which applies a resistive torque Tjoint (Noel & Hu, 2018a). Measurements have shown that there is an exponential growth of torque in relation to counter-clockwise angle θ (Figure 16B). This behavior further prevents prey from falling out of the mouth (Noel & Hu, 2018a).
Additionally, small, soft papillae aid in gripping saliva by the tongue (Noel & Hu, 2018a). Since it has now become clear that the role of saliva is essential for prey gripping, the role of these small papillae is undeniably important. Further, without saliva, food would be difficult to swallow and oral tissue would dry out, which constitutes a great risk for animals far from a water source. Short papillae make use of the surface tension of the saliva in order to keep the tongue surface wet and counteract the draining effects of gravity and evaporation (Figure 18) (Noel & Hu, 2018a).
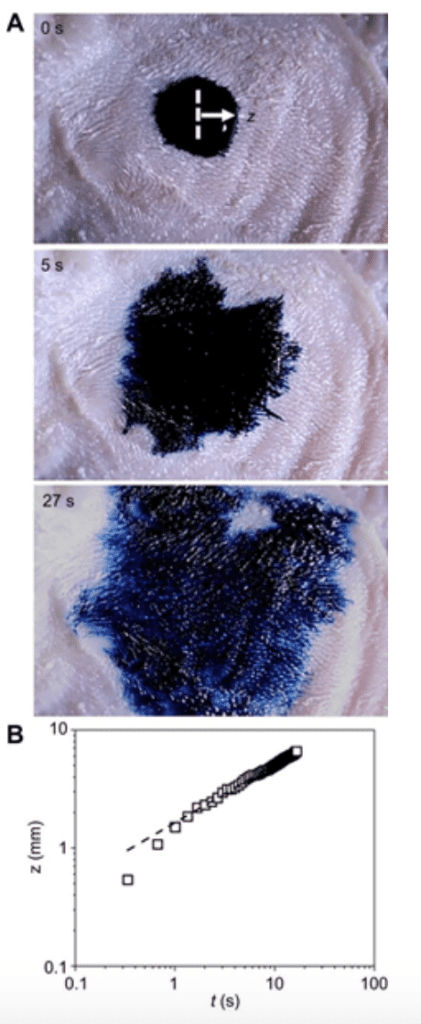
Fig. 18 Dyed water drop spreading on the tongue of a pig (Noel & Hu, 2018a).
An experiment was conducted on a pig's tongue where colored water droplets were deposited and the spreading of the color was observed (Figure 18) (Noel & Hu, 2018a). The motion of that liquid is called imbibition and helps the tongue wet itself again after eating dry foods or when water evaporates from it.
In addition to their function in prey gripping, there are important secondary uses of papillae, particularly in felines. Unlike the conical structure of papillae in most animals, felines distinguish themselves with a U-shaped cavity in their filiform papillae (Figure 19) (Noel & Hu, 2018b). These papillae, called cavo papillae, have unique structures to wick saliva and distribute it onto hairs in the grooming process (Figure 20) (Noel & Hu, 2018b). The saliva in the cavo papillae is wicked onto the hairs through surface tension (Fig.21). These papillae serve a range of purposes in felines, from detangling their hairs to cooling (Noel & Hu, 2018b).
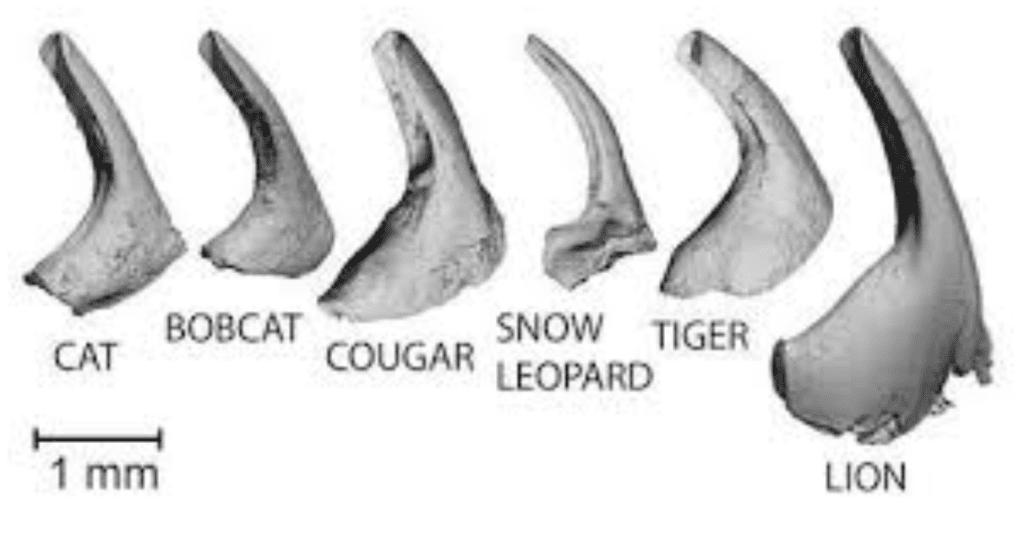
Fig. 19 Different feline papillae showing the characteristic U-shaped cavity (Noel & Hu, 2018b).
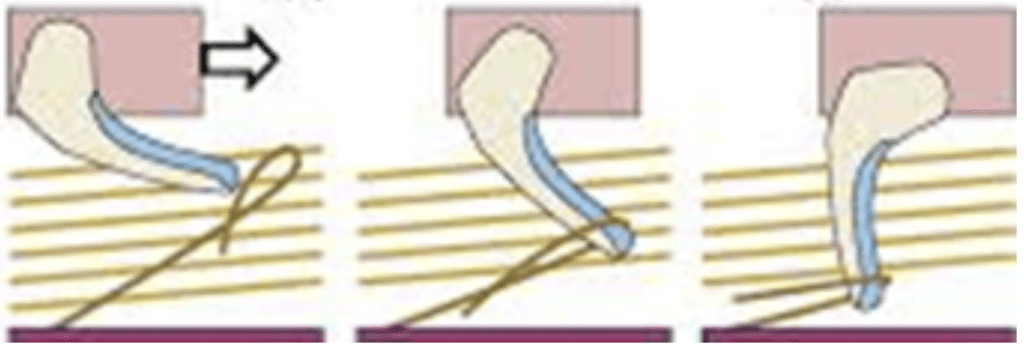
Fig. 20 Untangling of a feline's fur using filiform U-shaped papillae (Noel & Hu, 2018b).
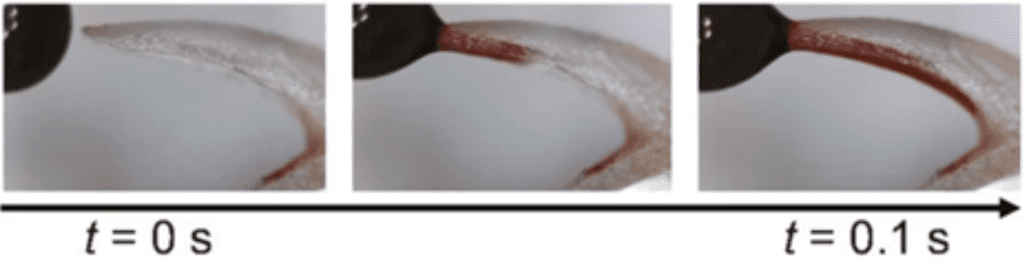
Fig. 21 Absorption of a colored droplet of water showing how a U-shaped papilla can transport saliva in its cavity (Noel & Hu, 2018b).
Biomimetic designs inspired by the physics of animal tongues
As discussed in this paper, animal tongues possess impressive mechanical properties and abilities which can serve as inspiration for the development of useful biomimetic designs. Such devices aim to replicate the useful biomechanical functions of various animal tongues for the purpose of improving the efficiency of engineering designs.
The rapid accelerations achieved by elastic recoil during chameleon tongue projection have inspired the development of similar structures in robotic arms, in order to increase speed and simplify the design (Zheng et al., 2018). In order to replicate the ballistic projection of chameleon tongues, engineers have constructed networks of concentrically stacked springs and sleeves (Figure 22). These springs attempt to simulate the network of intralingual sheaths responsible for the storage and release of elastic energy in chameleon tongues and allow the arm to reach objects at far distances very rapidly (Zheng et al., 2018).
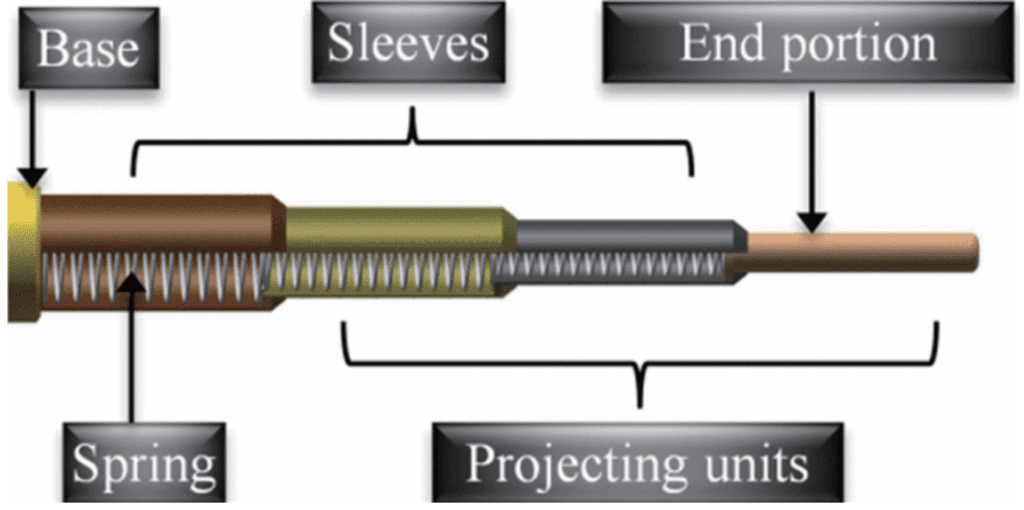
Fig. 22 Elastic design of a robotic arm inspired by a chameleon tongue (Zheng et al., 2018).
The fast, efficient fluid trapping strategy of hummingbirds has also inspired a lot of applications in the field of engineering or design (Hoff, 2021). For example, it inspired faster and more efficient ways of cleaning oil spills or other spilled liquids, which would have great environmental benefits (Hoff, 2021). These properties of hummingbird tongues can also inspire the development of faster and more efficient systems for the transport of fluids through pipelines (Hoff, 2021). Essentially, innovations based on hummingbird nectar extraction can significantly change our perception of the transportation of a thin-layer fluid.
Conclusion
In the animal kingdom, tongues of different species have evolved to perform impressive and unique mechanical functions. This paper outlines and analyzes how these functions can aid in the survival of a species, particularly in terms of prey-catching mechanisms. Animals that rely on the ability to capture fast-moving prey for survival, such as chameleons and frogs, have evolved to facilitate this process. The unique tongue structures of chameleons allow for rapid accelerations and large projection distances through elastic recoil mechanisms. Additionally, the non-Newtonian properties of a frog's saliva allow for efficient adhesion to prey once the tongue has been projected. Further, animals dependent on a certain food source for survival have adapted to more effectively access this food from their environments. Notably, hummingbird tongues have evolved to efficiently extract nectar from flowers due to their unique shape and ability to store elastic energy. Additionally, many animal tongues are lined with keratin microstructures known as papillae, in order to effectively grip the slippery or agile prey on which they rely for survival.
The study of animal tongue morphology and biomechanics, such as the examples discussed in this paper, can be applied to various engineering design problems and inspire the development of biomimetic devices, such as robotic arms and efficient fluid transport systems. By taking inspiration from biological systems, engineers can design unique and efficient devices to improve the human quality of life and understanding of the world.
References
Anderson, C. V., & Deban, S. M. (2010). Ballistic tongue projection in chameleons maintains high performance at low temperature. Proceedings of the National Academy of Sciences, 107(12), 5495–5499. https://doi.org/10.1073/pnas.0910778107
Anderson, C. V., & Deban, S. M. (2012). Thermal effects on motor control and in vitro muscle dynamics of the ballistic tongue apparatus in chameleons. Journal of Experimental Biology, 215(24), 4345–4357. https://doi.org/10.1242/jeb.078881
Anderson, C. V. (2016). Off like a shot: scaling of ballistic tongue projection reveals extremely high performance in small chameleons. Scientific Reports, 6(1). https://doi.org/10.1038/srep18625
Debray, A. (2011). Manipulators inspired by the tongue of the chameleon. Bioinspiration & Biomimetics, 6(2), 026002. https://doi.org/10.1088/1748-3182/6/2/026002
Franco, J. M., & Partal, P. (2010). The Newtonian Fluid. Rheology, 1, 76–95. https://books.google.ca/books?hl=en&lr=&id=zjw7CwAAQBAJ&oi=fnd&pg=PA74&dq=newton%27s+law+of+viscosity&ots=huuzTqYVh1&sig=cVAGkL9nUoYQHxwskhmFdvwx_2E#v=onepage&q=newton's%20law%20of%20viscosity&f=false
Galdi, G. P., & Necas, J. (1993). Recent Developments in Theoretical Fluid Mechanics: Winter School, Paseky, 1992. In Google Books. CRC Press. https://books.google.ca/bookshl=fr&lr=&id=vKxG_xOVVvYC&oi=fnd&pg=PA129&dq =papers+on+non+newtonian+fluids&ots=l59amUgz4C&sig=P2PxIrMd0RHkzMTCDEJl6b0DK5A
George, H. F., & Qureshi, F. (2013). Newton's Law of Viscosity, Newtonian and Non-Newtonian Fluids. Encyclopedia of Tribology, 2416–2420. https://doi.org/10.1007/978-0- 387-92897-5_143
de Groot, J. H., & van Leeuwen, J. L. (2004). Evidence for an elastic projection mechanism in the chameleon tongue. Proceedings of the Royal Society of London. Series B: Biological Sciences, 271(1540), 761–770. https://doi.org/10.1098/rspb.2003.2637
Herrel, A., Meyers, J. J., Nishikawa, K. C., & De Vree, F. (2001). Morphology and histochemistry of the hyolingual apparatus in chameleons. Journal of Morphology, 249(2), 154–170. https://doi.org/10.1002/jmor.1047
Hoff, M. (2016, August 18). Shape-shifting Tongue Is the Key to a Hummingbird's Nectar Gathering — Biological Strategy. Ask Nature. https://asknature.org/strategy/tongue-picks-up-liquid/#the-potential
Levine, R. P., Monroy, J. A., & Brainerd, E. L. (2004). Contribution of eye retraction to swallowing performance in the northern leopard frog, Rana pipiens. Journal of Experimental Biology, 207(8), 1361–1368. https://doi.org/10.1242/jeb.00885
Moulton, D. E., Lessinnes, T., O'Keeffe, S., Dorfmann, L., & Goriely, A. (2016). The elastic secrets of the chameleon tongue. Proceedings of the Royal Society A: Mathematical, Physical and Engineering Sciences, 472(2188). https://doi.org/10.1098/rspa.2016.0030
Noel, A. C., Guo, H.-Y., Mandica, M., & Hu, D. L. (2017). Frogs use a viscoelastic tongue and non-Newtonian saliva to catch prey. Journal of the Royal Society Interface, 14(127). https://doi.org/10.1098/rsif.2016.0764
Noel, A. C., & Hu, D. L. (2018a). The tongue as a gripper. The Journal of Experimental Biology, 221(7). https://doi.org/10.1242/jeb.176289
Noel, A. C., & Hu, D. L. (2018b). Cats use hollow papillae to wick saliva into fur. Proceedings of the National Academy of Sciences, 115(49), 12377–12382. https://doi.org/10.1073/pnas.1809544115
Rico-Guevara, A. (2017). Relating form to function in the hummingbird feeding apparatus. PeerJ, 5. https://doi.org/10.7717/peerj.3449
Rico-Guevara, A., & Rubega, M. A. (2011). The hummingbird tongue is a fluid trap, not a capillary tube. Proceedings of the National Academy of Sciences, 108(23), 9356–9360. https://doi.org/10.1073/pnas.1016944108
Rico-Guevara, A., Rubega, M. A., Hurme, K. J., & Dudley, R. (2019). Shifting Paradigms in the Mechanics of Nectar Extraction and Hummingbird Bill Morphology. Integrative Organismal Biology, 1(1). https://doi.org/10.1093/iob/oby006
Zheng, Z., Wang, K., Wu, X., Zhang, Q., & Li, K. (2018). Dynamic Analysis of Elastic Projecting Robot inspired by Chameleon Tongue. IEEE International Conference on Robotics and Biomimetics, 2088–2094. https://doi.org/10.1109/ROBIO.2018.8664861