A Chemical Review of Animal Tongue Functions
Shunyuan Xiao, Marc Srour, Sophie Allard
Abstract
In the animal kingdom, the evolution of tongue morphology has facilitated the survival of many species by providing advantages in foraging, avoiding predators, prey-catching and discriminating between food sources. Some species, notably the family of squamates, have developed impressive foraging strategies through the interpretation of chemical cues from their environment to locate prey, find mates and avoid threatening situations. These chemosensory searching abilities, relying on characteristics of the vomeronasal-lingual morphology and tongue-flicking behavior, are integral to the survival of many species of lizards and snakes. Other species, such as frogs, utilize the chemical properties of their tongue surfaces in order to adhere to the fast-moving and agile prey on which they rely for survival. Interactions between the mucins constituting the frog's saliva give the tongue impressive adhesive properties and enhance the prey-catching abilities of the species. While foraging and prey-catching abilities are critically important for the survival of some species, others depend more on the ability to discriminate between food through taste in order to avoid the consumption of harmful substances and to ensure consumption of the nutrients needed for survival. Such discrimination is achieved in mammals through the evolution of taste buds, which allow the transduction of taste information to the brain through chemoreceptors. Essentially, since the needs and environments of each species in the animal kingdom differ, the tongues of different species have evolved to perform specific and essential functions which can be understood through an analysis of chemical principles.
Introduction
The tongue is a complex muscular organ present in many species of the animal kingdom. In nature, the tongues of different species differ greatly in functions, morphologiesy and chemical properties. This impressive divergence illustrates nature's design solutions to various challenges faced by animals. While different species have different tongue functions, they most commonly serve to assist animals in foraging behavior, prey-catching and discrimination between food sources, which are essential to survival.
There are a few animals that possess particularly interesting tongue properties and functions. Many species of reptiles and snakes are tasked with the problem of searching for food in their environment. As such, strategic evolutions in their tongue morphology allow them to employ effective foraging strategies relying on the interpretation of chemical cues from their surroundings or from prey that have escaped their grasp. Similarly, a frog's diet consists mainly of fast-moving insects, and they are therefore reliant on an ability to rapidly and accurately catching their prey. The adhesive properties of their tongue surfaces due to the chemical composition of their saliva facilitates prey-catchingthis. Additionally, many mammals, notably cows, rely on the ability to discriminate between food sources for survival. As such, the evolution of mammalian taste buds is essential to the survival of many species. These chemical properties and functions of animal tongues illustrate some of the many ways species have adapted to their unique needs and environments, resulting in fascinating biodiversity.
Chemosensory searching in squamates
In nature, many animals rely on chemical cues in their environment to locate prey, avoid predators and find mates. Some species in particular rely heavily on chemoreception for foraging and survival; therefore, the organs involved in chemoreception, notably the tongue and the vomeronasal organ, have evolved rapidly in these species to facilitate this process. Squamates, a family of reptiles including lizards and snakes illustrated in figure 1, are a remarkable example of this (Baeckens et al., 2017).
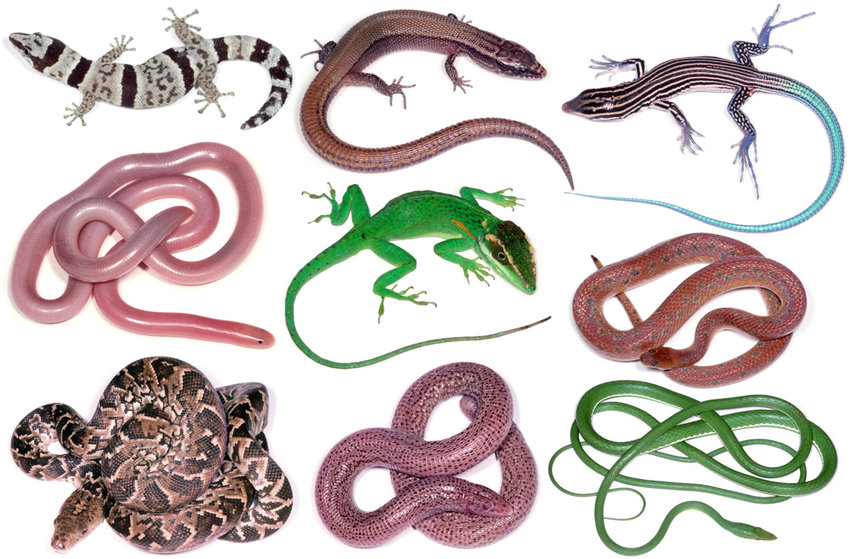
Fig. 1 Squamates (from top left to bottom right along each row: Sphaerodactylus cinereus, Cricosaura typica, Ameiva lineolata, Typhlops anousius, Anolis noblei, Tropidophis maculatus, Epicrates angulifer, Cadea blanoides, and Uromacer oxyrhynchus) (Vidal & Hedges, 2009).
Morphology and evolution of the vomeronasal-lingual organs
In order to explain the mechanisms through which lizards and snakes utilize chemoreception as a foraging strategy, the morphology of the different structures involved and how they have evolved in different species must be understood. There are two main organs involved in chemosensory searching in squamates: the tongue and the vomeronasal organ (VNO), Jacobson's organ (Ruiz-Monachesi et al., 2022). In general, the tongue of a lizard or a snake can be separated into three distinctive sections: the bifid apex, the body and the root (Abbate et al., 2010). To illustrate these components, the stereo micrographstereomicrograph of an Italian lizard tongue is presented below (Fig. 2).
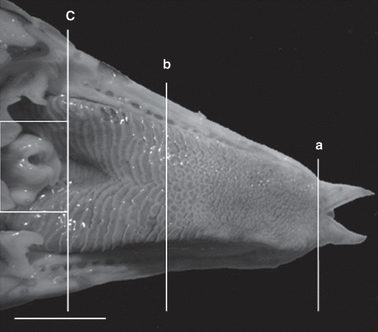
Fig. 2 Stereo micrograph of the dorsal surface of the tongue of an Italian lizard. a) End of bifid apex b) End of tongue body c) End of tongue root (Abbate et al., 2010).
For chemoreceptionary functions, the bifid apex of the tongue, which represents the forked tip to the right of (a) in figure 1, serves an essential role. In various species of lizards and snakes, the degree to which the tongue tip bifurcates differs considerably (Fig. 3). The forked tip of squamate tongues allows efficient sampling of chemical cues from the environment, due to the increased surface area for contact with the air during tongue protrusion. Additionally, their bifurcated tongues give them the ability to sample and sense signals from both sides of the body separately, increasing the efficiency of chemoreception in the search for prey or the avoidance of predators (Baeckens et al., 2017). Within the family of squamates, some species rely more heavily on chemoreception than others; more specifically, active foragers depend on chemical cues to locate prey, while sit-and-wait foragers rely more on visual cues (Baeckens, Van Damme, et al., 2017). As such, the evolution of active foragers has led to increasingly bifurcated tongues, suggesting a correlation between the degree of reliance of a species on chemoreception and the specialization of their lingual morphology to do so.
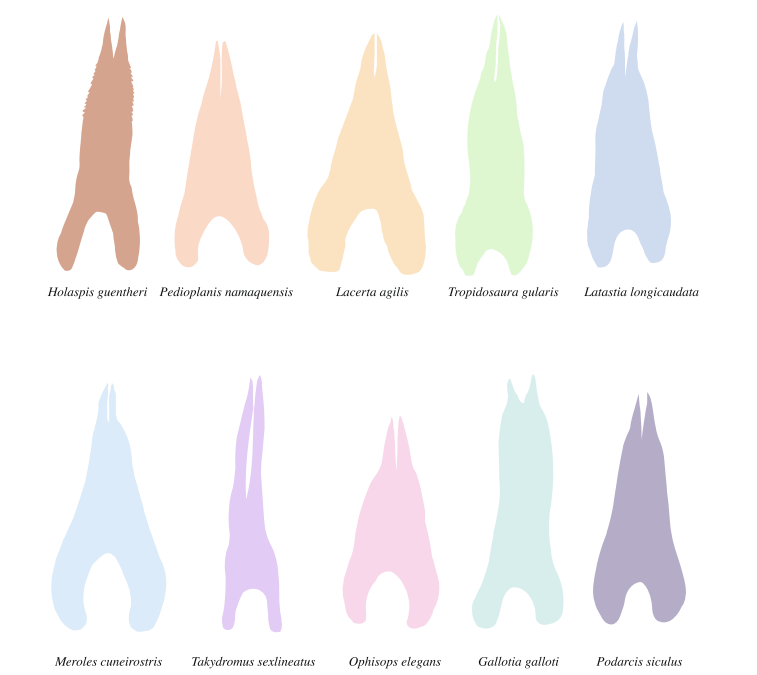
Fig. 3 Illustration of the tongue's shape in 10 lizard species (Baeckens et al., 2017).
For example, the species with the most bifurcated tongue in Ffigure 3 is the Takydromus sexlineatus, commonly known as the Japanese lacertid lizard (Fukudome & Yamawaki, 2016). Studies have shown that these lizards are active foragers, relying on visual cues as well as chemoreception to rapidly catch spiders and insects (Fukudome & Yamawaki, 2016). Adding on, one of the species with the least bifurcated tongues in Ffigure 3 is the Podarcis siculus, commonly known as the ruin lizard (Avery, 1991). Observations of these lizards have revealed that they spend much of their time immobile, observing their surroundings, and this behavior is consistent with that of sit-and-wait foragers (Avery, 1991). These two examples of the relationship between tongue forkedness and foraging strategies corroborate the above hypothesis, and many other similar examples can be found in nature.
Another important element of the chemosensory system in lizards and snakes is the vomeronasal organ (VNO). Located on the roof of the mouth above the tongue, the vomeronasal organ consists of a cartilaginous non-sensory body covered by a sensory epithelium (Ruiz-Monachesi et al., 2022). In order for the VNO to receive chemical signals to interpret, the palatal opening connects the oral cavity to the VNO (Ruiz-Monachesi et al., 2022). Much like the bifurcated tongues in squamates, patterns in the evolution of the VNO have also been observed. Morphological studies have revealed that a thicker epithelium is associated with an increased functionality of the VNO and an improved ability to discriminate between chemical cues from the environment (Baeckens et al., 2017). Essentially, species that are more reliant on chemical sensing for prey and predator recognition have evolved to have a thick VNO sensory epithelium and a highly forked tongue (Baeckens et al., 2017).
Mechanisms of chemoreception
Given an understanding of the vomeronasal-lingual morphology of squamates and the driving forces for the diversity of certain characteristics of these organs in different species, the mechanisms of chemoreception can be discussed. Overall, chemosensory searching involves the collection of chemical information from the environment and the transduction of this information such that it can be interpreted by the brain of the animal (Ruiz-Monachesi et al., 2022).
The process begins when sensory receptors in the nose are stimulated by volatile chemical components emitted from a food source (Schwenk, 2000). More specifically, these volatile compounds are characterized by their ability to evaporate easily at room temperature, generally due to weak intermolecular interactions (Anand et al., 2014). As such, these chemicals readily disperse in their environments and are easily collected by the advanced olfactory system in squamates. The binding of volatile compounds to chemoreceptors in the nose triggers tongue-flicking, a chemosensory process unique to squamates (Schwenk, 2000). In tongue-flicking, the tongue exits the oral cavity and oscillates through the air in order to capture the odor molecules emitted from a food source (El-Mansi et al., 2020). Following the collection of these chemicals on the tongue epithelium, squamates retract their tongues for chemical analysis (Fig. 4). The chemical scents collected, adhering mainly to the bifid apex of the tongue, are carried to the palatal opening by a hydraulic mechanism and then stimulate the chemoreceptor cells of the VNO epithelium, which sends the collected information to the brain via sensory neurons (Ruiz-Monachesi et al., 2022).
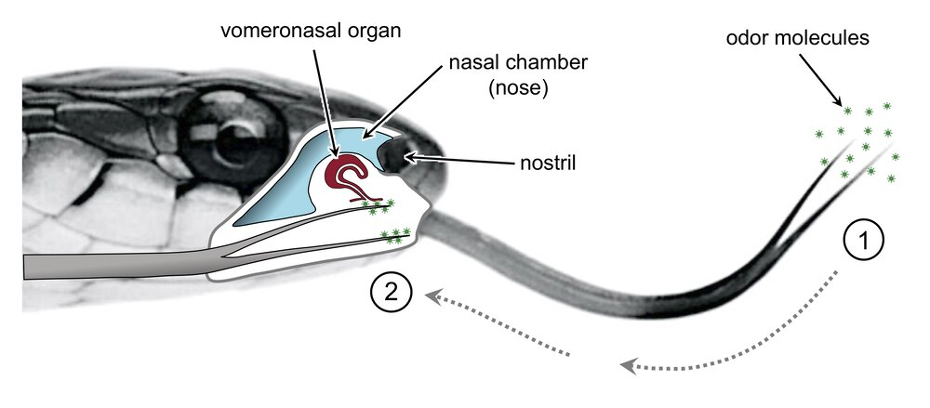
Fig. 4 Tongue-flicking behavior of a snake. 1) Collection of environmental odor molecules by the bifurcated tongue. 2) Retraction of the tongue and transportation of chemicals to the palatal opening and the VNO for interpretation (Schwenk, 2021).
While most squamates utilize similar tongue-flicking mechanisms for chemoreception, there are some interesting features unique to certain species that are worth noting. In particular, the striped sand snake (Psammophis siblians) has a unique tongue body allowing for efficient prey trailing using chemoreception (El-Mansi et al., 2020). The lingual body of these snakes is characterized by a central chamber lined with fascicles of odor receptor cells (Fig. 5). This chamber allows for more efficient sampling of chemical cues. Further, since the chamber is in contact with the lingual tip, studies suggest that it allows for the analysis and interpretation of chemical signal strength on either side of the body separately and simultaneously (El-Mansi et al., 2020).
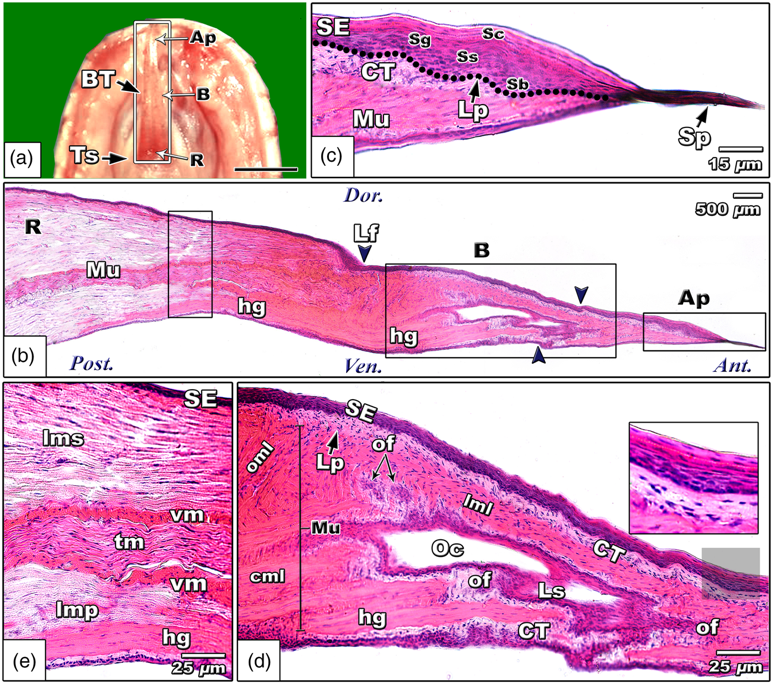
Fig. 5 Histological micrograph of the striped sand snake tongue showing the central olfactory chamber (Oc) (El-Mansi et al., 2020).
Strike-induced chemosensory searching
In addition to its use in general exploration, tongue-flicking is also employed in lizards and snakes in strike-induced chemosensory searching (SICS). This foraging strategy involves an increased rate of tongue-flicking following a prey strike. In fact, a study of SICS in ten different lizard species revealed that the rate of tongue-flicking increased by a factor of 10 after biting prey (Ballinger et al., 1992). This foraging strategy is exhibited by many lizards but is most common in venomous snakes. These snakes first strike their targeted prey, injecting it with venom. Following the initial strike, the tongue-flicking rate increases dramatically, suggesting it is no longer simply for exploratory purposes. Through the mechanisms of chemosensory searching outlined above, the snake is then able to collect and interpret odor molecules emitted from the prey, as well as its own venom, to locate the target now killed by the poisonous venom, and feed themselves (Teshera & Clark, 2021). Following an unsuccessful feeding attempt, the chemosensory abilities of squamates are heightened through an increased number of tongue flicks and therefore an increased collection of chemical cues. This strategy allows these foragers to efficiently locate their target with minimal delay.
Adhesive surface chemistry of frog saliva
Frogs, toads, chameleons and many other animals rely heavily on the physical, chemical, and biomechanical properties of their tongues to attack and capture their prey. The tongue aids the predator to quickly and effectively seize its meal. These amphibians mainly target insects, which are small, agile, and fast. Thus, the predator must be equipped with a high-velocity prey-gripping mechanism and evolution has facilitated the development of this mechanism. In particular, frogs can project and retract their tongue at impressive speeds (Noel et al.,2017). However, the greatest challenge faced by frogs in prey-catching is maintaining their grip on the prey, as forces attempt to slide it off the tongue. In order to overcome these difficulties, the surface chemistry of a frog's tongue has evolved to possess important adhesive properties.
Prey-gripping in frogs
An essential component of the adhesive prey-catching mechanism in frogs is the mucus coating their tongue (Fowler et al., 2018). Mucus has a wide variety of uses throughout the animal kingdom and does not always function as an adhesive. For example, some snails make use of two distinct mucuses, one adhesive and the other non-adhesive (Fowler et al., 2018). Other animals are characterized by the presence of mucus with adhesive but not significant mechanical properties. This reveals that mucus is a complicated chemical mixture with a broad range of properties and chemical compositions depending on the needs of the animal and the chemical composition of the mucus (Fowler et al., 2018). Therefore, the adhesion of an insect on a frog's tongue is a complicated process involving a highly specific mucus that has distinguished physical and chemical properties.
Chemical composition of frog saliva
In order to examine the adhesive properties of a frog's tongue, a study was conducted to analyze the superficial chemical configuration of the frog saliva after a tongue strike (Fowler et al., 2018). The study focused on evaluating the first few nanometers of the mucus coating the tongue to understand what makes this fluid so sticky on a chemical level. The study revealed that all mucus is primarily composed of mucins, regardless of adhesiveness. Mucins are glycoproteins that are rich in cysteine ends and have a serine, threonine, and proline rich core. They are often arranged with saccharide chains in a bottle-brush formation (Fig. 6) (Fowler et al., 2018). Mucins can form large tertiary structures and are often found in random coil clusters in solution (Fowler et al., 2018). These intra-molecular connections are notably due to the covalent disulfide bonds between two adjacent cysteine molecules (Fig. 6) (Fowler et al., 2018).
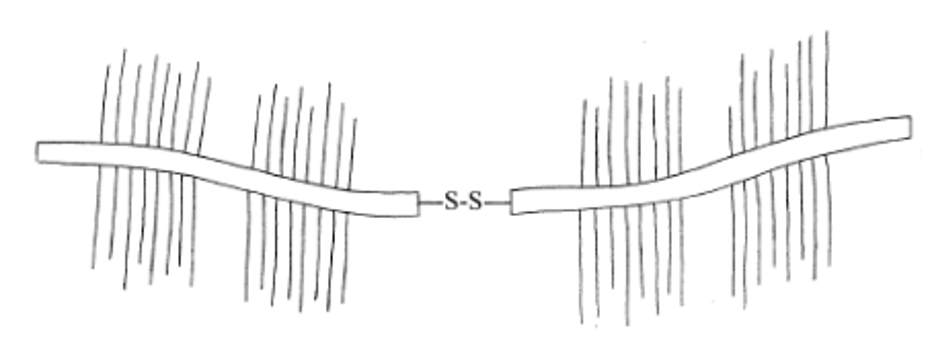
Fig. 6 Mucin bottle-brush formation and tertiary structure (Kubba et al., 2000).
Interestingly, the study revealed that a fibril formation within the mucus of a frog was present between the tongue and the surface when the animal retracts its tongue (Fig. 7) (Fowler et al., 2018). Since mucins form naturally disordered tertiary structures and coils, the presence of these fibrils illustrates that the chemical structure of the mucus has changed during tongue retraction. The fibril formations act as an essential component in the attack mechanism of the frog. In fact, they behave as shock absorbers in the retraction phase by connecting the surface of the prey to the tongue, thus creating responsive adhesive forces (Fowler et al., 2018).
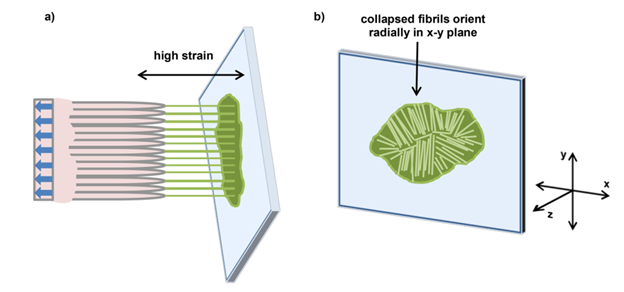
Fig. 7 (a) Mucus fibrillation between tongue and surface in a high strain scenario (b) Collapsed mucus fibrils and their orientation (Fowler et al., 2018).
Near edge X-ray absorption fine structure (NEXAFS) is an effective type of absorption spectroscopy that utilizes quantum physics principles such as photon absorption to obtain data on electronic structure, chemical composition, symmetry, and energy distribution on the surface of carbon-containing substances (Vlasov et al., 2012). In order to further understand the chemical composition of frog mucus, an experiment was carried out to measure the angle at which these mucins are oriented by following the change in intensity in a NEXAFS spectroscopy as the angle varies between 30° and 70° (Fowler et al., 2018). Further data from the NEXAFS experiment revealed the nature and distribution of the bonding orbitals in the mucus. C—C π*, C—OH σ*, C—C σ*, N—C—O π*, and N—CH2 σ* bonds were spread across the entire mucus sample characterizing proteins and peptide bonds (Fowler et al., 2018). The distribution of the bonds was relatively homogeneous throughout the sample, except for the edges that were especially rich in C—OH σ* bonds (Fig. 8) (Fowler et al., 2018). This can be explained by the presence of hydroxyl groups that comprise saccharide ring side chains, indicating a high concentration of glycoproteins (Fowler et al., 2018).
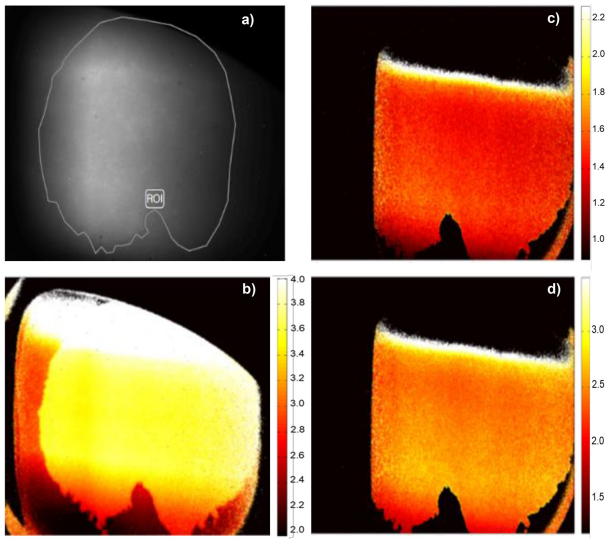
Fig. 8 NEXAFS measurements at a 30° angle showing (a) the selected region studied, C—OH σ* (b), N—C—O π* (c), and N—C σ* (d) photons. The brighter the color the higher the photons intensity (Fowler et al., 2018).
Moreover, there was a peak observed in the NEXAFS results near 289 eV corresponding to the C—OH σ* orbital (Fowler et al., 2018). This once again illustrates the presence of hydroxyl groups on the saccharide ring side chains, which are related to glycoproteins. Figure 9 illustrates the C1s and N1s K-edge spectra obtained through this experiment and shows the peak at 289 eV, as well as three other important features which correspond to important orbitals present in mucins (Fowler et al., 2018).
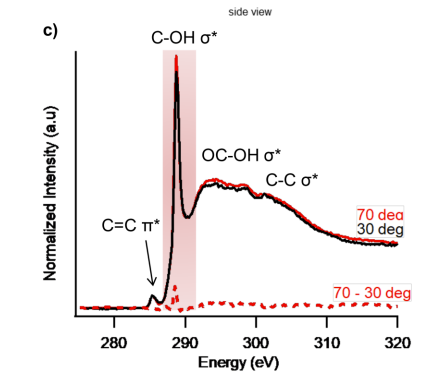
Fig. 9 C1s and N1s K-edge spectra obtained through NEXAFS illustrating 4 features corresponding to σ* and π* bonding orbitals in mucins (Fowler et al., 2018).
In NEXAFS, the angle of inclination of the studied polymer molecule plays an important role. The intensity was higher for images at 70° than for a 30° inclination, which suggests a certain degree of alignment of the hydroxyl groups (Fowler et al., 2018). Thus, the angle of alignment of the fibrils was determined to be 70° as shown in Figure 10. This result shows that the fibrils have a greater range of elongation granting them the elasticity they need to withstand the great forces of retraction of the tongue muscle. Another important conclusion drawn from these results is the alignment of the hydrophilic hydroxyl groups in this orientation. Since these groups are oriented away from the surface and toward neighboring mucin chains, Van der Waals interactions between these groups contribute to the adhesive properties of the mucus on a frog's tongue (Fowler et al., 2018).
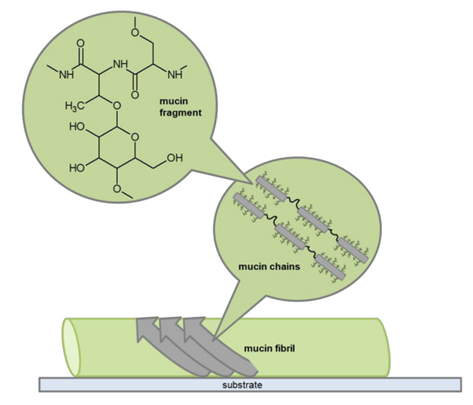
Fig. 10 Proposed molecular configuration of mucin on a frog's tongue in the retraction phase (Fowler et al., 2018).
The configuration of the mucins at a primary, secondary and tertiary structure gives the tongue its stickiness by forming fibrils that act as shock absorbers when the tongue is retracted (Fowler et al., 2018). This confirms the pressure-sensitive properties of the saliva, since the fibers formed by glycoproteins are present when the shear stress is at its lowest (when the tongue retracts). Without these formations, saliva would be highly ineffective since mucus would simply come off the tongue and provide no additional prey-adhesion (Fowler et al., 2018).
Mammalian tasting signal transduction
Mammals are one of the most abundant animal species on Earth given their large size, and their unique evolutionary path has allowed them to evolve one of the most specialized taste organs: the taste buds. Despite differences in the evolution of taste organs in different species, in general, mammalian taste buds share similar functions. In terms of taste perception, the taste buds, which are distributed on the tongue, serve as neurological receptive organs that perform the primary task of taste perception. Over the last ten years, the molecular mechanisms that taste buds use for chemosensory transmission have been the focus of many studies. As a result, the state-of-the- art is characterized by a high level of knowledgeresearchers currently have a lot of knowledge regarding the mechanisms underlying sweet, bitter, and umami tastes, and there isare making rapid progress regarding salty and sour transduction.
Essentially, taste buds receive molecular information and translate it into neural information. An excellent example through which to introduce these organs is the bovine tongue. Cows have a verythe most developed gustatory sensory system compared to otherof any mammals, with 14,975 to 21,691 taste buds distributed on the tongue of a six-year-old cow (Davies et al., 1979). These herbivores have evolved to possess such advanced chemosensory systems because it is essential for their survival to distinguish between safe and unsafe plants for consumption. In contrast, the tongue of an adult human has only about 10,000 taste buds in total, but only 2000-4000 taste buds are functioning (KidsHealth, 2019). To illustrate these taste organs, the dorsal surface of an ox tongue is illustrated in figure 11.
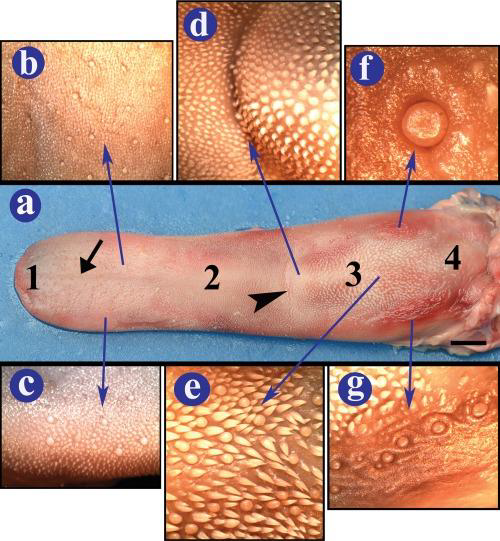
Fig. 11 (a) Dorsal view of an ox's tongue, (b-c) shows the filiform and fungiform papillae of the lingual body, (d-g) the pPapillae in other forms and different locations. All these papillae are types of taste buds on the ox's tongue (Erdoğan & Pérez, 2014).
Molecular transduction mechanism of acidic tasting stimuli
Sour tastes, which are developed through the presence of acids, may serve as a defense mechanism against absorbing too much dietary acid and upsetting an animal's essential acid-base balance. Additionally, sour tastes aid many species in avoiding rottenexpired meals and unripe fruit, which may threaten their survival (Roper, 2007). Therefore, it is essential for mammals to identify sour tastes within their food; molecular transduction mechanisms in the taste buds on their tongues facilitate this.
Acids are known to dissociate in water, forming a proton H+, which then combines with the surrounding water molecules to form H3O+. These extracellular ions can cross the cell membrane through ion channels, inducing an electric current (Roper, 2007). In this instance, H3O+ acts as a neurotransmitter, defined as a chemical that can create an electric potential between the outside and inside of the cytosol of a neuron. As such, a neuron adjacent to the taste cell would either polarize or depolarize. In this case, the adjacent neuron would polarize since H3O+ carries a positive charge. This current is an electric signal that tells the brain that something sour has been consumed by the animal. Consequently, the brain would provide a feedback signal (sour taste) to the rest of the organism's body, which it uses to determinetelling the animal whether to continue or ceasestop eating.
Given an understanding of the mechanisms through which acidic stimuli induces a sour taste response, varying degrees of stimuli can be investigated. A study by Roper investigated the following puzzle in taste transduction: it is perplexing that the degree of sourness in diluted solutions of various organic and inorganic acids is only sporadically associated with their pH (Roper, 2007). For example, acetic acid has a pH of 3.9 and induces sour taste in mammals, while HCl has the same pH and is comparatively tasteless. To investigate this, an experiment was conducted on rats (Roper, 2007). Recordings of the chorda tympani nerve after stimulating the tongue with an acetic acid solution at pH 5.5 ([H+] 3 M) and a hydrochloric acid solution at pH 3 ([H+] = 1 mM) showed the same response, despite the large difference in pH between the solutions (Roper, 2007). To understand this result, it should be noted that there are three nerves associated with tongue and taste: the facial nerve, the glossopharyngeal nerve, and the vagus nerve (Gibbons & Sadiq, 2020). The glossopharyngeal nerve is responsible for taste reception on the tongue, and the chorda tympani nerve belongs to this cranial branch (Gibbons & Sadiq, 2020). The results of this experiment revealed that sour taste is not solely related to the concentration of protons in the solution, as previously believed.
Extracellular protons can cross the cell membrane through ion channels and ion exchangers like the sodium-hydrogen exchanger pump and acidify the cytoplasm if they are present in high concentrations (low pH), which accounts for the sour taste of relatively concentrated solutions of HCl and other mineral acids (Roper, 2007). Additionally, the molecular form of organic acids, such as the protonated HAcetate in Ffigure 12, can cross the lipid plasma membrane, due to their neutral charge, and enter the cytosol. As HAcetate breaks down inside of the cell, H3O+ and acetate ions are released, acidifying the cytosol and lowering the pH (Roper, 2007). Organic acids are rich in carboxylic acid groups, –COOH. Because these acids have more oxygen atoms than inorganic acids and oxygen is highly electronegative, electrons can easily be separated from –COOH groups. As such, organic acids taste sourer than mineral acids because there would be more H+ ions dissociated from the molecule to acidify the cytosol (Difference between Organic Acid and Inorganic Acid | Definition, Structure, Properties, 2017).
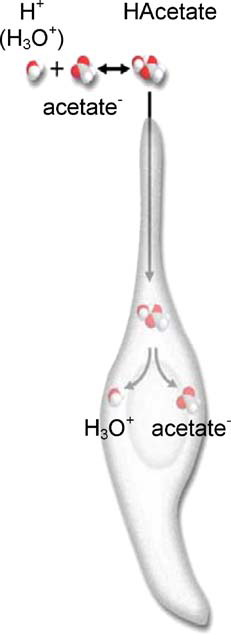
Fig. 12 Illustration of the permeation of an acid (HAcetate) into the cell and its dissociation within the cytoplasm (Roper, 2007).
In addition to the mechanisms discussed above, there are other pathways through which extracellular protons can enter the cell. For example, amiloride-sensitive epithelial sodium channels (ENaC) have been studied in hamster taste cells and were concluded to have proton permeability (Roper, 2007). In a study by Ugawa investigating rat taste cells and the sensitivity of acid-sensing ion channel-2a (ASIC2a) to acid solutions (altering extracellular pH), ASIC2a was revealed as a main contributor to sour taste identification in taste buds (Ugawa, 2003). A growing body of research suggests that there exist many other sour-taste channels. Since the study of sour taste genes has only just begun, additional research needs to be conductedis needed to further explain sour tastes in mammals.
Molecular transduction mechanism of salty taste
A common ion, Na+, is known to be the primary trigger for salty taste and table salt (NaCl) is most associated with this. The transduction of salty taste is valuable to many mammalian species, since Na++ and Cl– are important nutrients required to maintain blood osmolality, blood pressure, and body water regulation. If the solute concentration is not equal on both sides of the selectively permeable cell membrane, water flow is induced towards the side of higher solute concentration through osmosis. This process explains why high Na+ concentration could result in blood osmolality disorders, causing cells to expand or contract (Rasouli, 2016). These ions also account for neuron signal transmission, as the ion concentration gradient allows the transfer of electric signals between cells. Therefore, it is important to maintain the appropriate concentration gradient for the transmission of taste information to function properly (Rasouli, 2016). Further, since Cl— is important in maintaining acid-base balance, the ability to recognize salty foods through transduction mechanisms is essential to the survival of many mammalian species.
As mentioned above, Na++ and Cl— ions can have a significant impact on the cell metabolism and neuron signal transmission. Given their importance to mammals, the mechanisms through which taste buds recognize these ionsm through salty taste is essentialimportant to understand. Research has revealed that systemic conditions resulting in elevated levels of aldosterone change salt taste sensations (DeSimone & Lyall, 2006). This interesting result suggests that salt taste reception may involve one of the sodium transporters that is targeted by this hormone (DeSimone & Lyall, 2006). In fact, the epithelial-sodium channel (ENaC) is the mammalian Na+-specific taste receptor, as described below (Fig. 13).
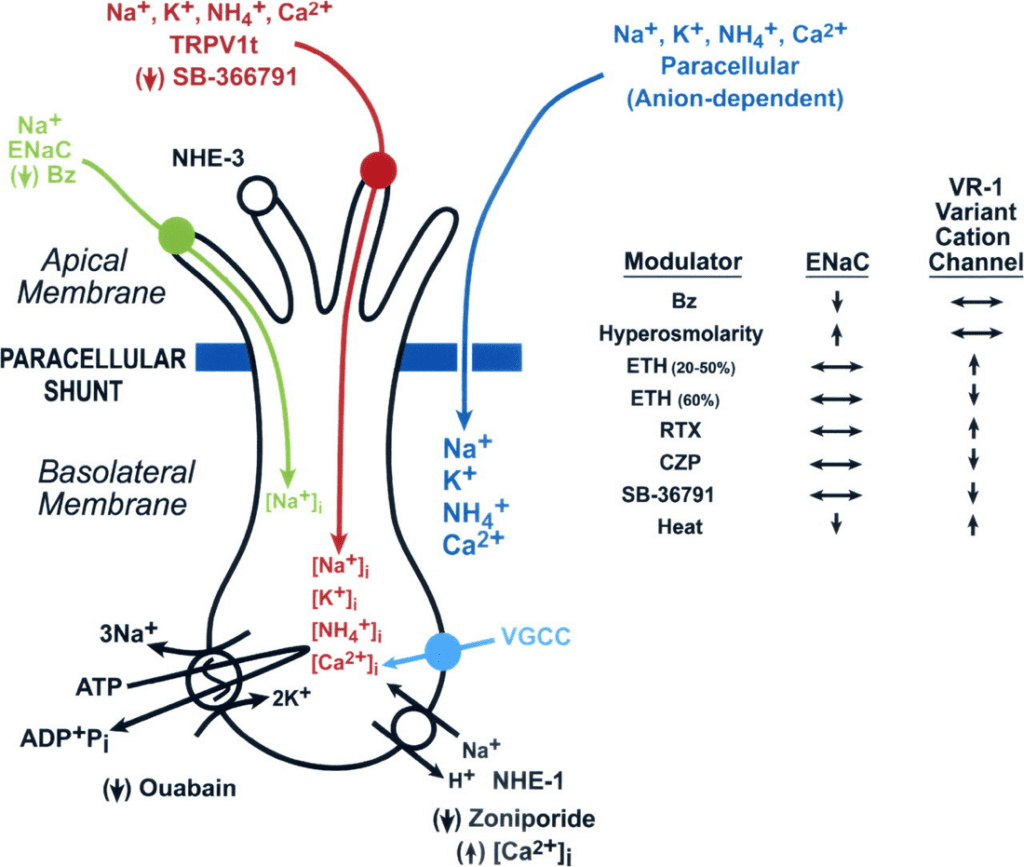
Fig. 13 Illustration of taste receptor cells, highlighting the ENaC Na+ channel (green) and the channel for reception of other cations (red) (DeSimone & Lyall, 2006).
In studies conducted on rat and hamster taste buds, amiloride, a type of drug, was found to inhibit Na++ current across taste cell membranes (DeSimone & Lyall, 2006). These findings allowed researchers to conclude that ENaC plays a function in Na++ taste reception, since amiloride or its more powerful analogue, benzamil, blocked taste nerve responses to NaCl in numerous species, but left other taste modalities unaffected (DeSimone & Lyall, 2006). Essentially, mammalian taste buds are characterized by two distinct receptors for the interpretation of salty taste: epithelial-sodium channels for responses to Na++ and another receptor for responses to other cations (DeSimone & Lyall, 2006). Both receptors are illustrated in Ffigure 13 and play an essential role in the discrimination between different tastes in mammals.
Conclusion
The tongue is a muscular organ present in many species of the animal kingdom. Through evolution, an impressive divergence in tongue morphology and functions between species has emerged. This diversity allows each species to adapt to its individual needs and environment, in order to facilitate survival through advantages in prey-catching, foraging and in the discrimination between food sources. This paper outlined various unique chemical features of animal tongues and their role as nature's design solution to the problems faced by animals.
Many animals, notably squamates, are faced with the difficult task of searching for food and avoiding predators. To facilitate this, they rely heavily on chemosensory searching through tongue flicking to collect and interpret chemical cues from their environment. By making use of their specialized vomeronasal-lingual morphology, these animals can effectively locate prey, avoid predators and find mates, all of which contribute to the survival of their species. Other modes of chemical interpretation through taste buds present on the tongue surface allow mammals to distinguish between sour, salty, bitter, sweet, and umami tastes during the consumption of food through chemical pathways. The ability to make these distinctions allows mammals to avoid harmful or poisonous foods which threaten their survival, as well as ensure that they obtain the proper nutrients to sustain them. In addition to the tongue's functions in processing and interpreting chemicals from the environment and from food, the surface chemistry of the tongue itself can have useful properties and applications for some animals. For example, since frogs are faced with the problem of catching fast-moving and agile prey, evolutionary adaptations to their tongue's surface have appeared as natural solutions to this conundrum. The unique chemical structure of the mucus coating a frog's tongue is responsible for the adhesive properties of the tongue and its efficacy in prey-catching.
Overall, through the evolution of tongue morphology in the animal kingdom, many species have adapted useful chemical advantages to solve problems unique to their specific needs and lifestyles. Further investigations into such features of animal tongues can serve as inspiration for unique biomimetic designs and allow us to better understand interactions between species and their reliance on chemistry.
References
Abbate, F., Guerrera, M. C., Montalbano, G., Zichichi, R., Germanà, A., & Ciriaco, E. (2010). Morphology of the Lingual Dorsal Surface and Oral Taste Buds in Italian Lizard (Podarcis sicula). Anatomia, Histologia, Embryologia, 39(2), 167–171. https://doi.org/10.1111/j.1439-0264.2010.00992.x
Anand, S. S., Philip, B. K., & Mehendale, H. M. (2014). Volatile Organic Compounds. Encyclopedia of Toxicology, 967–970. https://doi.org/10.1016/b978-0-12-386454-3.00358-4
Avery, R. A. (1991). Temporal dynamics of a vigilance posture in the ruin lizard Podarcis sicula. Amphibia-Reptilia, 12(3), 352–356. https://doi.org/10.1163/156853891×00509
Baeckens, S., Herrel, A., Broeckhoven, C., Vasilopoulou-Kampitsi, M., Huyghe, K., Goyens, J., & Van Damme, R. (2017). Evolutionary morphology of the lizard chemosensory system. Scientific Reports, 7(1), 10141. https://doi.org/10.1038/s41598-017-09415-7
Baeckens, S., Van Damme, R., & Cooper, W. E. (2017). How phylogeny and foraging ecology drive the level of chemosensory exploration in lizards and snakes. Journal of Evolutionary Biology, 30(3), 627–640. https://doi.org/10.1111/jeb.13032
Ballinger, R., Coady, N., Prokop, J., & Lemos-Espinal, J. (1992). Strike-Induced Chemosensory Searching: Variation Among Lizards. Transactions of the Nebraska Academy of Sciences and Affiliated Societies, 128. https://digitalcommons.unl.edu/tnas/128/?utm_source=digitalcommons.unl.edu%2Ftnas%2F128&utm_medium=PDF&utm_campaign=PDFCoverPages
Davies, R. O., Kare, M. R., & Cagan, R. H. (1979). Distribution of taste buds on fungiform and circumvallate papillae of bovine tongue. The Anatomical Record, 195(3), 443–446. https://doi.org/10.1002/ar.1091950304
DeSimone, J. A., & Lyall, V. (2006). Taste Receptors in the Gastrointestinal Tract III. Salty and sour taste: sensing of sodium and protons by the tongue. American Journal of Physiology-Gastrointestinal and Liver Physiology, 291(6), G1005–G1010. https://doi.org/10.1152/ajpgi.00235.2006
Difference Between Organic Acid and Inorganic Acid | Definition, Structure, Properties. (2017, June 17). Pediaa.com. https://pediaa.com/difference-between-organic-acid-and-inorganic-acid/
El-Mansi, A. A., Al-Kahtani, M. A., Abumandour, M. M. A., & Ahmed, A. E. (2020). Structural and Functional Characterization of the Tongue and Digestive Tract of Psammophis sibilans (Squamata, Lamprophiidae): Adaptive Strategies for Foraging and Feeding Behaviors. Microscopy and Microanalysis, 26(3), 524–541. https://doi.org/10.1017/s1431927620001312
Erdoğan, S., & Pérez, W. (2014). Anatomical and scanning electron microscopic characteristics of the oropharyngeal cavity (tongue, palate and laryngeal entrance) in the southern lapwing (Charadriidae:Vanellus chilensis,Molina 1782). Acta Zoologica, 96(2), 264–272. https://doi.org/10.1111/azo.12075
Gibbons, J. R., & Sadiq, N. M. (2020). Neuroanatomy, Neural Taste Pathway. PubMed; StatPearls Publishing. https://www.ncbi.nlm.nih.gov/books/NBK545236/
Fowler, J. E., Kleinteich, T., Franz, J., Jaye, C., Fischer, D. A., Gorb, S. N., Weidner, T., & Baio, J. E. (2018). Surface chemistry of the frog sticky-tongue mechanism. Biointerphases, 13(6), 06E408. https://doi.org/10.1116/1.5052651
Fukudome, M., & Yamawaki, Y. (2016). Behavioural interactions between the lizard Takydromus tachydromoides and the praying mantis Tenodera aridifolia suggest reciprocal predation between them. Journal of Ethology, 34(3), 231–241. https://doi.org/10.1007/s10164-016-0468-6
Ishimaru, Y., Inada, H., Kubota, M., Zhuang, H., Tominaga, M., & Matsunami, H. (2006). Transient receptor potential family members PKD1L3 and PKD2L1 form a candidate sour taste receptor. Proceedings of the National Academy of Sciences, 103(33), 12569–12574. https://doi.org/10.1073/pnas.0602702103
KidsHealth. (2019). What Are Taste Buds? (for Kids) – KidsHealth. Kidshealth.org. https://kidshealth.org/en/kids/taste-buds.html
Rasouli, M. (2016). Basic concepts and practical equations on osmolality: Biochemical approach. Clinical Biochemistry, 49(12), 936–941. https://doi.org/10.1016/j.clinbiochem.2016.06.001
Kubba, H., Pearson, J. P., & Birchall, J. P. (2000). The aetiology of otitis media with effusion: a review. Clinical Otolaryngology and Allied Sciences, 25(3), 181–194. https://doi.org/10.1046/j.1365-2273.2000.00350.x
Noel, A. C., Guo, H.-Y., Mandica, M., & Hu, D. L. (2017). Frogs use a viscoelastic tongue and non-Newtonian saliva to catch prey. Journal of the Royal Society Interface, 14(127). https://doi.org/10.1098/rsif.2016.0764
Roper, S. D. (2007). Signal transduction and information processing in mammalian taste buds. Pflugers Archiv : European Journal of Physiology, 454(5), 759–776. https://doi.org/10.1007/s00424-007-0247-x
Ruiz-Monachesi, M. R., Abdala, C. S., & Cruz, F. B. (2022). Allometry and morphological integration shape the chemical detection system in Liolaemus lizards (Squamata, Iguania). Zoologischer Anzeiger, 299, 221–233. https://doi.org/10.1016/j.jcz.2022.06.005
Schwenk, K. (2000). Feeding in Lepidosaurs. In Feeding: Form, Function, and Evolution in Tetrapod Vertebrates (pp. 175–291). Academic Press. https://doi.org/10.1016/b978-012632590-4/50009-5
Schwenk, K. (2021, June 17). Smelling in stereo: The real reason snakes have flicking, forked tongues. Phys.org. https://phys.org/news/2021-06-stereo-real-snakes-flicking-forked.html
Teshera, M. S., & Clark, R. W. (2021). Strike-Induced Chemosensory Searching in Reptiles: A Review. Herpetological Monographs, 35(1). https://doi.org/10.1655/0733-1347-35.1.28
Ugawa, S. (2003). Identification of sour-taste receptor genes. Anatomical Science International, 78(4), 205–210. https://doi.org/10.1046/j.0022-7722.2003.00062.x
Vidal, N., & Hedges, S. B. (2009). The molecular evolutionary tree of lizards, snakes, and amphisbaenians. Comptes Rendus Biologies, 332(2-3), 129–139. https://doi.org/10.1016/j.crvi.2008.07.010
Vlasov, I. I., Turner, S., Van Tendeloo, G., & Shiryaev, A. A. (2012). Chapter 9 – Recent Results on Characterization of Detonation Nanodiamonds. In O. A. Shenderova & D. M. Gruen (Eds.), ScienceDirect (pp. 291–326). William Andrew Publishing. https://www.sciencedirect.com/science/article/pii/B9781437734652000098