Fancy Footwork: A Morphological Study of the Role of the Hoof in Ungulate Locomotion in Habitats Around the World
Yitian Zhang, Claire Driedger, Marshall Moussavi, Emmanuel Menacho Tardieu
Abstract
In many mammals around the world, nature has designed a variable and complex structure placed at the tips of limbs called a hoof. Hoofed mammals, also called ungulates, are divided into two orders: even- and odd-toed ungulates. The divergence of these mammals from a common ancestor has led to the mechanical and morphological specialization of hooves within different living environments and habitats. This report offers a physical examination of the hoof, outlining how eight animals' hooves help circumvent challenges associated with their habitats. The hippopotamus's mechanical control of their even-toed hooves reflects their semi-aquatic lifestyle. In African savannahs, both the zebra's and giraffe's hooves help these animals migrate efficiently over long ranges. Moose hooves allow these ungulates to cope with the unique climatological conditions posed by their snowy environment. Like the hippopotamus, the dexterity of the mountain goat's hooves is linked to their cliffside habitat. Horse hooves are often protected by horseshoes, which prevent their attrition during the repeated impact of the hoof on the ground. Finally, in the desert, the camel's footpads fulfill a function which is like that of hooves. In short, hooves solve both universal physical challenges, such as supporting the weight of the animal and providing traction, and challenges unique to the environment inhabited by specific animals.
Introduction
Mammal habitats around the world vary greatly, from deserts to mountains. To meet the environmental needs of ungulate mammals, evolution has created an elegant and intricate morphological feature: the hoof, which is found at the tip of the foot. The hoof wall consists of a thick, hard nail made of keratin that surrounds the toes of a mammal. The hoof can be of varying shapes, but is generally rounded, oval, or slightly pointed at the tip. The weight of the mammal is passed down through the long pastern bone to the short pastern bone and finally into the coffin bone, where the force is distributed through the sole corium onto the hoof wall. Figure 1 illustrates this anatomy.
.
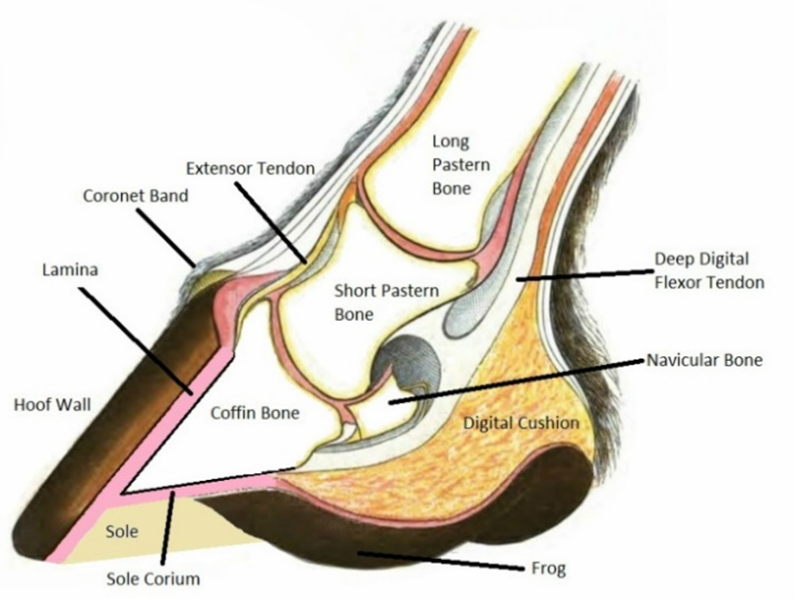
Fig. 1 The anatomy of the equine hoof (Okanagan School of Natural Hoof Care, retrieved from Alderman).
Today, all ungulates fall within two orders: perissodactyls – even-toed ungulates – who bear their weight on an even number of toes, and artiodactyls – odd-toed ungulates – who bear their weight on an odd number of toes (Groves & Grubb, 2011). During the early Eocene period, all hoofed mammals distributed their weight over five toes (Universitat Basal, 2014). However, 54 million years ago, the side toes of some hoofed marsh-dwellers dwindled significantly in size or completely disappeared, creating the order of even-toed ungulates (British Museum of Natural History, 1906). Still, even-toed mammals were forced to survive on the fringes of the ecosystems, in environments which only offered cellulose-rich plant foods. This environmental constraint initiated the diversification of even-toed ungulates, who would go on to develop multiple stomachs to facilitate the digestion of plant material (Klappenbach, 2020). When the global climate shifted during the Miocene period (15 MYA) towards grasslands-dominated environments, even-toed mammals began to outnumber odd-toed mammals.
Despite the differences between odd-toed and even-toed ungulates, the hooves of these mammals solve similar physical challenges. All hooves dissipate the energy created by the foot's impact with the ground during locomotion. Similarly, this tough, keratin-filled nail supports the weight of the animal as it stands. Hooves also solve the design challenge of protecting the delicate tissues and bones within the mammal's foot.
Macroscopically, there are also unique mechanical adaptations of hooves that can be observed in various mammals – from hippopotami to mountain goats – which solve specific challenges according to that animal's habitat. Along with evolutionary history, environmental and lifestyle factors like surface conditions, migratory patterns, and natural predatory pressures determine the structure of hooves. This paper will study the hooves of eight different mammals to reveal the diversity in the morphological and mechanical features of hooves in the natural world.
Hippopotamus (Hippopotamus amphibius)
The common hippopotamus (Hippopotamus amphibius) is the second largest land animal besides elephants and rhinoceros (Clauss et al., 2003). The common hippopotamus and the pygmy hippopotamus are the only two extant species in the family of Hippopotamidae (Taxonomy, 2020). Taxonomically, hippopotamuses are classified as even-toed ungulates (Keller et al., 2009). Unlike many other even-toed ungulates, the hippopotamus is a semi-aquatic animal that lives in lakes, rivers and mangrove swamps. It is widely distributed in the grasslands to the south of the Sahara Desert in Africa (Nowak & Figueiredo, 1999). The transition from land to water can be challenging for mammals who are adapted to life on land. In order to survive and adapt to their aquatic environment, land mammals must evolve cardiovascular systems that allow them to breathe in water and the corresponding body structures, such as enlarged webs or flat tails (Reidenberg, 2007). For example, platypus evolved enlarged webs which help them swim, and muskrats evolved flat tails, which help them balance while walking on the ground and swimming in the water. However, hippopotamuses never evolved these specializations to paddle in the water. Even though they cannot breathe in water, they can float and swim in the water at the speed of 8 km/h thanks to their hooves (Owen-Smith & Chafota, 2012). In general, the opening and closing state of the hippopotamus' hooves helps them paddle in water and supports their body weight, respectively. Figures 2 and Figure 3 illustrate the opening and closing state of the right forefoot in hippopotamuses, respectively.

Fig. 2 Closing state of the right forefoot (Endo et al., 2018).

Fig. 3 Opening state of the right forefoot (Endo et al., 2018).
Note: a) Dorsal; b) Medio-dorsal; c) Planter; d) Latero-dorsal for Figures 2 and 3.
This adaptation allows the hippopotamus to be semi-aquatic which means it can walk on rigid surfaces and live in aquatic environments. The semi-aquatic adaptation is achieved by the transition between the mediolateral opening state and the closing state of the hippopotamus' hooves (Raji et al., 2008). Based on Endo et al.'s CT examination, the opening and closing state of hippopotamus hooves are performed by the adduction and abduction of the most medial and lateral phalanges (Endo et al., 2018). These opening and closing movements enlarge the width of the hippopotamus' hooves and thus change the hooves' shape. Among many even-toed ungulates, only the central two digits touch the ground. The lateral digits are usually shorter and only touch the soft ground (Perich, 2003). These lateral digits are called “false hooves” or “dewclaws.” The false hooves contribute to reducing shock and preventing slipping when animals jump. This morphology is frequently observed among animals that live in mountain or cliff habitats, such as mountain goats (Lewinson & Stefanyshy, 2016). Even though hippopotamuses do not have false hooves, their second and fifth digits are shorter than the third and fourth digits under the opening state, as shown in Figure 3. In the resting state, hippopotamus hooves are opened, and the arrangement of the carpals, metacarpals, tarsals, metatarsals, and phalanges is mediolaterally enlarged. A mediolaterally widened foot may be required to support the weight ranges of 1500kg to 3200kg of hippopotamuses when they stand on rigid ground (Marshall & Sayer, 1976). Also, the opening state of digits allows a more even distribution of the hippopotamus's weight on their hooves than the closed state (Roland et al., 2003). This stability helps hippopotamuses migrate upstream during the dry season. In contrast, the closed state of hooves reduces water resistance so that hippopotamuses can swim in water (Endo et al., 2018). Occasionally, hippopotamuses open their feet to paddle in deep water where they cannot touch the ground.
Pygmy hippopotamuses share similar characteristics of hooves with the common hippopotamus. However, the resting state of their hooves maintains an opening state, even in water (Endo et al., 2018). This might be due to lighter body weight. As such, their weight can be evenly distributed on all four toes.
Unlike most even-toed ungulates, such as mountain goats and giraffes, the angle of the hippopotamus' hooves is nearly 90˚ between the dorsal edge of the line and the plantar heel to shoe line, and the diameter of their hooves is about the same as the diameter of their ankles. The vertical and cylindrical shape of the hooves makes the hippo more stable on land, but it also limits their mobility since a smaller angle could reduce the work needed to lift the leg and minimize impact force upon landing. However, hippopotamuses do not need to walk or stand for long periods of time. They spend most of their day in aquatic or semi-aquatic environments. The vertical morphology also supports their body weight.
Zebra (Equus)
Zebras are widely distributed in grasslands and savannas in Eastern and Southern Africa. While living in grasslands and woods with no shelter, zebras have adapted many of their features to avoid predators, such as lions and hyenas (Naidoo et al., 2016). Zebras' black and white stripes are effective at confusing enemies that only have black and white vision. Zebras are in the same family as horses – the equine family; thus, these two animals share similar morphologies and physical appearances. The zebra's hoof wall is more rigid than a horse's, and the frog angle is wider in zebras than in horses. These two main differences between zebras and horses are due to artificial selection. Modern domesticated breeds of horses evolved around 3500 BC, but zebras have never been domesticated by humans (Librado et al., 2021). Zebra hooves are specialized to migrate over long distances and to avoid predators in the wild

Fig. 4 The modified lateral and dorsal view of a Zebra's hooves (Davies, 2002). The angle is between line (A) from the proximal limit to the distal limits of the dorsal hoof wall at the weight-bearing border with the line drawn from the palmar margin of the heel and the shoe, and the most dorsal margin of the toe and the shoe.
In general, the hoof consists of four exterior layers. From the outside, an individual hoof consists of the stratum externum, the stratum medium, the stratum internum and the dermis parietes (Douglas & Thomason, 2000). Since the stratum externum is very thin, it is difficult to distinguish between stratum medium and stratum externum. The stratum medium is located in the middle, and it is the thickest part of the hooves. The plantar of a zebra's hooves is covered with a thick, rough keratin structure that can be compared to a racehorse's horseshoe. This thick layer of keratin protects the soft interior structure of the horse's hooves capsule and prevents abrasion from long-distance migration (Roland et al., 2003). The wide frog of the zebra's hooves helps the zebra maintain stability while traveling through grasslands. The wide angle distributes the animal's weight more on the dorsal side of hooves than on the plantar side. This specialization is widely found among wild equines, since they require high mobility and vigilance (Tacchio et al., 2010). Distributing body weight on the front side of hooves helps equines mobilize quickly, which is a vital survival skill in the wild environment. During migration season, zebras usually migrate from the Ngorongoro area in southern Tanzania to the Masai Mara in lower Kenya around the Serengeti National Park, and return annually with the rain as shown in Figure 5 (Mijele et al., 2016).
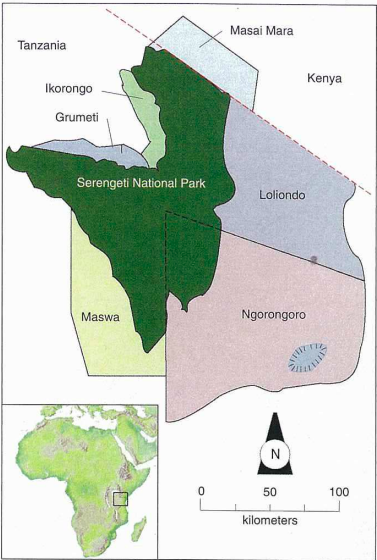
Fig. 5 The modified migration map of zebras based on Migele et al.'s migration path and Wolanski et al.'s map (Adapted from Mijele et al., 2016; Wolanski et al., 1999).
Turner's equation investigates the relationship between body mass and the hoof area (Anderson et al., 2021) and this is shown in Equation 1. This equation is utilized to analyze the balance of hooves and the probability of lameness among equines. The balance of hooves is an essential indicator to quantify the health of equines' hooves.
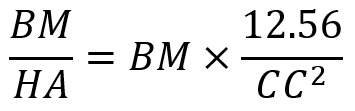
Equation 1 Turner's equation, where BM stands for body mass; HA stands for surface area of hooves; CC stands for coronet circumference.
The HA is calculated by πr2, r is the radius of hooves. CC is calculated by 2πr. Even though zebras are in the same family as the horse, the zebra's hooves are smaller than the horse's due to its smaller body size. The average shoulder height of a horse is 1.57 m compared with the average shoulder height of 1.35 m of a zebra (Jant & Neumann, 1998). Based on Turner's equation, the ratio of 1.65 kg/cm2 and 1.1 kg/cm2 for the fore- and hind-foot, respectively, would give an ideal result for the size of hooves and body mass (Souza & Souza Junio, 2021). However, the ratio for zebras is smaller than the given value among all equines. This demonstrates that zebras have relatively larger hooves than their body size suggests. The relatively large hooves maintain the balance of zebras better and dissipate more energy for them.
Giraffes (Giraffa)
While the long neck of the giraffe may be its most recognizable adaptation, the giraffe sports another evolutionary feature: its hooves. As seen in Figure 6, giraffes are even-toed ungulates with cloven hooves, and they walk on their tip toes (Encyclopedia Britannica, nd.). A closer examination of the giraffe's hooves reveals the incredible physical link between a giraffe's habitat and its behavior.

Fig. 6 Model of left hind giraffe foot obtained from a CT scan (Adapted from Stieger-Vanegas, 2015).
Considering that the average shoulder height of giraffes is 274 cm, and that their average stride length is 247 cm, giraffes have a proportionally small mean hoof area of 189.6 cm2 (Cumming, 2003). Further examination reveals that the larger the body mass of the giraffe, the larger the interface of the giraffe hooves with the ground. The relationship between the pressure exerted by a force over a given area, or in this case, by the weight of the giraffe over the interface of the hoof with the ground, respectively, is defined by Equation 2, with p = pressure (Pa), m = mass (kg), g = gravity (m/s2), and a = area (m2).
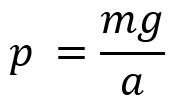
Equation 2 The pressure exerted on the hoof is proportional to the mass of the giraffe.
Not only does this equation hold true for giraffes, but the pressure exerted per unit area on the ground remains identical across many large African ungulates , as plotted in Figure 7 (Cumming, 2003).

Fig. 7 Ordinary least squares regression showing the relationship between the adult female mass of African large mammal herbivores and hoof area (Adapted from Cumming 2003).
Surprisingly, however, the contact points of the healthy giraffe's hoof with the ground are not over the whole hoof, but rather are limited to the periphery of the foot. As seen in Figure 8, the giraffe hoof is in the shape of a concave oval, with turquoise paint marking the hoof's contact points with the ground.

Fig. 8 The solar surface of the giraffe's foot is concave with hoof ground contact points around the periphery of the foot including the hoof wall, heel, and parts of the sole (Dadone, 2021).
The dynamic between a giraffe's hooves and their environment is intricate. To begin, the home of the giraffe is sub-Saharan Africa, where it inhabits the semi-arid savanna biome and travels through grasslands. Also, the climate of the sub-Saharan desert consists of scarce water availability and extreme temperatures (Bergstrom & Skarpe, 1999).
The habitat of the wild giraffe directly affects the health of the giraffe's hoof. Specifically, hoof diseases and overgrowth are less prevalent in wild giraffes compared to captive giraffes (Dadone & Foxworth, 2021). In wild giraffes, the rough substrate of sub-Saharan terrain causes friction against the hoof, wearing down on it and preventing overgrowth. Furthermore, the dry climate of the semi-arid savannah maintains giraffe hoof health since moist conditions soften the hoof and present the opportunity for the penetration of bacteria and debris into the hoof (deMaar, 2000). Not only are the hooves of a giraffe perfectly adapted to their environment, but their hooves also necessarily dictate the nature of this mammal's interactions with its environment (Klappenbach, 2018).
Healthy giraffe hooves power their quadrupedal locomotion which is necessary for their survival. Firstly, fast locomotion is of vital importance for these ungulates to escape predators (Owen-Smith & Mills, 2008; Sinclair et al., 2003). In order to survive within the open grasslands of Africa, giraffes have evolved the ability to run up to 60 km/h, and it is the hoof which powers this biomechanical movement by lengthening the leg (New World Encyclopedia, 2009). In Equation 3, speed is written as a function of the shoulder height of giraffes, where u = speed (ms-1), Fr = Froude number, h = shoulder height (m) and g = acceleration due to gravity 9.81 ms2. Froude number is a dimensionless value that describes the ratio between inertial and gravitational forces.
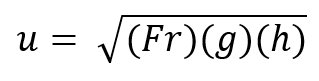
Equation 3 Dimensionless speed increases with increasing ungulate shoulder height (Basu et al., 2019).
The equation above demonstrates that, as the shoulder height of the giraffe increases, so does its speed. Giraffe hooves can reach heights of up to 15 cm in males and 10 cm in females (Dagg & Foster, 1976). Therefore, hooves help giraffes survive by increasing the length of their legs, and thus by increasing their speed, such that they can survive the predatory challenges posed by their environment.
On the other hand, the mechanical and morphological features of the giraffe's hoof permit these creatures to execute a slower, more sustained speed over greater distances without injury. At one point, giraffes were odd-toed ungulates and had five-digited hooves. Eventually, the third and fourth digits of these hooves began to bear more weight while the other digits bore less, creating the giraffe's cloven hooves (Shorrocks, 2016). This transfer of weight to only two digits improves their movement over grassy terrain and the dexterity of their limbs (Shorrocks, 2016). Also, giraffes have double-pulley astragali, bones in their ankle joints which prevent the hoof from rolling side to side yet increases forward-to-back foot flexibility and springiness (Prothero, 2009). According to the Encyclopedia Britannica (nd.), the astragalus increases the “propulsive thrust at the ankle and thus enables a quicker recovery at the end of a stride before starting the next one”. The giraffe's astragalus ankle bone can be seen in Figure 9, and its location within the giraffe's lower leg can be seen in Figure 10.
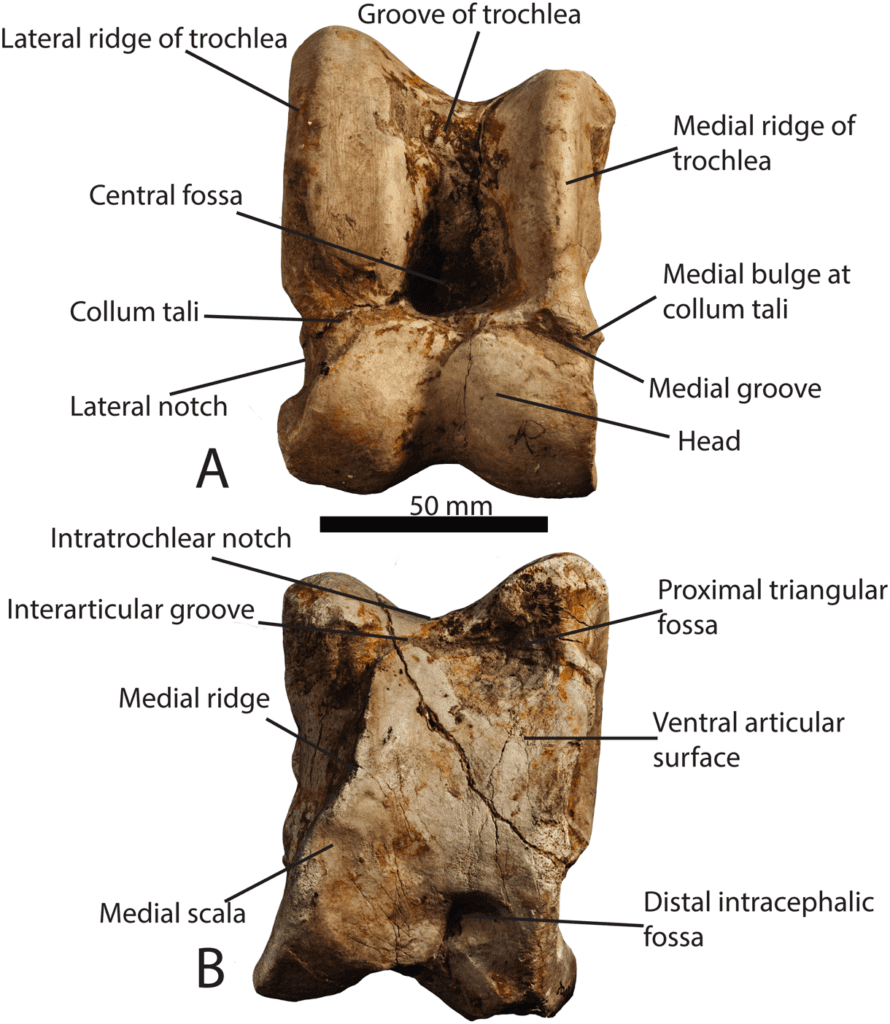
Fig. 9 Photograph of a Samotherium major astragalus in dorsal view, with representative terminology (Solounias 2016). The scale bar represents 50 mm.

Fig. 10 Right hind leg of a Masai giraffe at San Diego Zoo. The giraffe is an even-toed ungulate with double-pulley astragali (Adapted from Burnett, 2007).
Giraffes occupy large home ranges when searching for food and water. As such, sustained locomotion is necessary for the giraffe because it increases its “foraging efficiency where food sources are scarce” (Shorrocks, 2016). For example, on the individual level, giraffe habitats can span up to 654 km2 during the dry season (Grzimek's Animal Life Encyclopedia). For another, the daily average area covered in Nairobi National Park by bulls is 5.9 km, by cows it is 2.9 km, and by calves it is 3.3 km per day (Shorrocks, 2016). It is the durability and dexterity of the cloven hoof of the giraffe which permits them to escape predation and to travel without injury
Moose (Alces alces)
The moose is the largest member of the deer family. Characteristically, moose inhabit forests which receive snowfall. More specifically, the mean snow depth received by moose habitats ranges from 47-77 cm (Hundertmark et al., 1990). The moose is well-suited for life in these areas thanks to its cloven hooves, as shown in Figure 11. This adaptation, partially caused by predatory pressure, increases individual ungulate survival in snow by “permitting use of food resources over a greater proportion of the species' home range” (Telfer & Kelsall, 1984).
First, it is important to consider the structure of moose hooves. Moose have cloven hooves, whose two main digits correspond to the vestigial third and fourth digit, along with two small posterolateral declaws, corresponding to the vestigial second and fifth digits. For a bull, the front hoof width is 12.5 cm, for a cow, it is 10 cm, and for a calf, it is just under 7.5 cm (Department of Inland Fisheries & Wildlife Maine). However, this artiodactyl's third and fourth digits are asymmetrical. In fact, the mean total length of the fourth digit is longer than the third digit in 73% to 100% of specimens, and it is also broader than the third digit. This asymmetry and the anatomy of the moose hoof can be visualized in Figure 11 below. Anna Keller (2009) of the University of Zurich, says that this asymmetry in digits “suggests a different function during stance and weight bearing” and that this could be as a result of selective evolution favoring “locomotion on soft ground.”

Fig. 11 A bull moose' front foot (Adapted from Peziol, 2017). There is wear on the declaws and the toes are asymmetrical.
The substrate of the moose's natural terrain is quite soft. In the summer, moose can be found wading along forest-edged shallow lakes and streams, foraging for mosses, lichens, and submerged aquatic vegetation (Geist, 2022). In the winter, moose trample in snow, as seen in Figure 12.
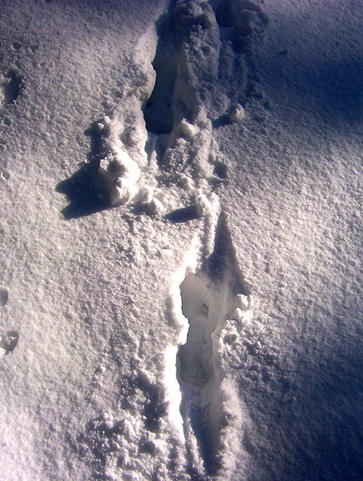
Fig. 12 Moose tracks in deep snow, Alaska (Beeblebrox, 2003). Long legs and hooves allow moose to wade easily through deep water or snow.
The nature of the substrates found in the moose's environments, namely mud, soft soil, and snow, affect the health of the moose's hooves. For example, in the case of a captive wild European moose, walking on flooring, which is much “harder than the soil in natural moose habitat” caused chronic hoof overgrowth and changes in phalangeal bone structure (Clauss, 2009). Therefore, the habitat of the moose plays a direct role in the morphology of the moose's hoof.
Similarly, hooves play a role in ensuring that moose can survive within their habitat. For one, the declaws of a moose further increase the surface area of their hooves when they are in contact with the ground. For another, these ungulates reduce the weight-load of their body on ground by spreading the toes of their hooves, which is useful when walking in muddy or marshy terrain, or over soft snow. More specifically, it was experimentally recorded that the surface area of a moose's track was increased by approximately 10 percent when the toes were splayed (Kelsall, 1969). As such, the moose's hooves act like snowshoes, supporting this animal in its environment. It follows that the weight-load-on-track can be calculated by dividing the weight of the animal by its total track area. This measurement can be compared to snow hardness. If the snow hardness exceeds the weight-load-on-track, it can be predicted that the moose will be able to walk on top of the snow, or only sink into it minimally. As seen in Figure 13, the amount of snow left under the moose's footprint is proportional to the initial snow on the ground.

Fig. 13 The amount of snow left under the moose footprint in relation to snow depth (Lundmark & Ball, 2018). The regression line shows the cut-off point when shallow snow may start to affect sinking depths of moose, as viewed by the intercept at 20.13 cm of snow.
The depth of the moose's footprint is important because if moose sink up to chest height into soft snow, plowing through this snow will cost energy, and could threaten their survival (Kelsall, 1969). To cope with this challenge, moose may proceed by trampling a “moose yard,” a system of trails in deep snow (Geist, 2022). Therefore, not only do the hooves help the animal during quadrupedal locomotion, but also during their daily behavioral movements.
Hooves play a role in helping moose achieve their nutritional requirements. During the summer, the moose's cloven hooves act like paddles, propelling this large mammal forward for long distances, and over 5 meters underwater, where they can find and eat vegetation (Ames, 2019). During the winter, these ruminants will scrape away the snow to reveal mosses and lichens (National Geographic).
The moose's cloven feet help them escape predators by enabling them to trot at high speeds (Geist, 2022), and they try to avoid predators altogether by avoiding areas with little to no snow cover, since these areas pose an increased risk for predation (Telfer & Kelsall, 1984). The moose has evolved more morphological adaptations to snow than its predators, namely the wolf, the coyote, and the wolverine (Telfer & Kelsall, 1984). Therefore, since moose are more well adapted to snow than their predators thanks to their hooves, they can avoid or escape predation and source food.
Mountain goat (Oreamnos americanus)
The hoof plays an important role in enabling the formidable climbing ability of the mountain goat. Since the hoof interacts with a uniquely rocky substrate, and a simple mistake or slip on a cliff can result in a goat's death, it is morphologically well-adapted. Mountain goats are even-toed ungulates, belonging to the order Artiodactyla in the family Bovidae, which comprise cloven-hooved mammals. Endemic to western Canada and USA, they occupy subalpine and alpine ecosystems. Most of their time is spent foraging in escape terrain, termed so because the steep, rocky slopes allow the goats to escape from less well-adapted predators (Hjeljlord, 1973; Rideout, 1977). This ability to outclimb predators is enabled by their front-heavy musculature. As a result, mountain goats have a muscle-bound plodding appearance, and they rarely run or jump (Casebeer et al., 1950). Mountain goats are generalist herbivores and tend to eat what is available, tending to prefer locations near escape terrain. This makes sense as predation from wolves, cougars and grizzly bears is the most common cause of mortality (Festa-Bianchet et al., 1994).
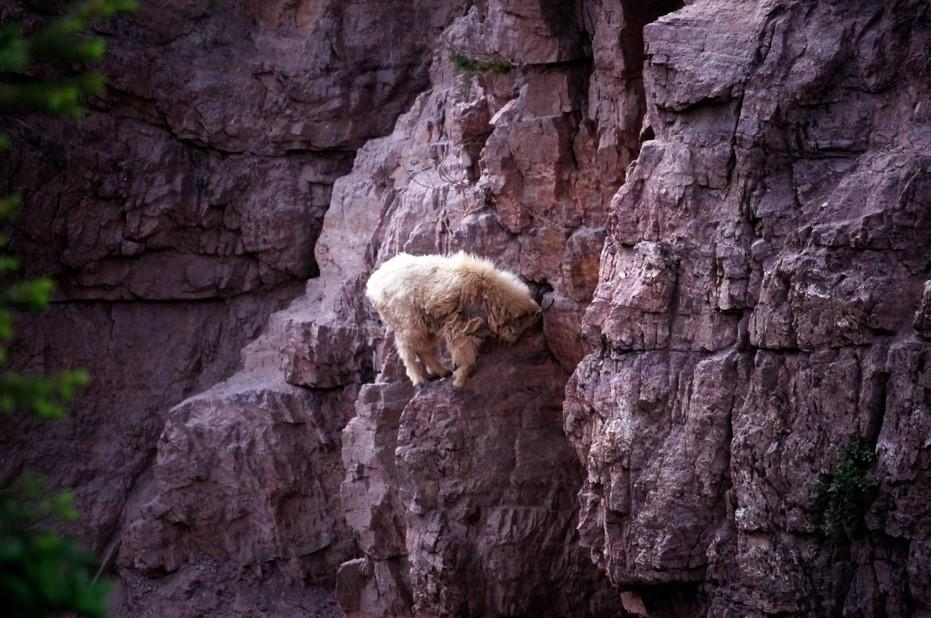
Fig. 14 This image illustrates the need for specialized hooves so that mountain goats do not fall. From Mountain goat climbing (SurprisePally, 2008).
Mountain goat hooves consist of two digits as seen in Figure 15 below. Both these digits have a hard keratin edge and a soft traction pad that together provide the most effective grip. Irregularities in the rocky terrain push up into the softer sole, which conforms. The bump that is created is then trapped by the hard edge, and if there is loose dirt or rocks, it accumulates under the hooves providing more grip. Even on smooth ice and wet rock, the soft pad provides a considerable amount of extra friction. The individual toes also improve grip: they can spread apart when placed or squeezed together for secure placement. When going downhill, the digits will spread apart in a V shape.

Fig. 15 This figure shows how the digits of mountain goat hooves can spread apart in a V shape (Adapted from Basic Care, 2022 and Garman, 2021).
The hoof accumulates material if the goat begins to slide forwards, in order to increase friction. Furthermore, a vector of force that is orthogonal to the goat's weight is produced from its weight. This is like creating a V with one's index finger and middle finger and pushing down and forwards on a surface. Since part of the goat's weight is directed sideways, it decreases the force that opposes friction directed downhill. This increases the force that is required to overcome friction and make the goat slip. Additionally, dewclaws provide traction downhill (Chadwick, 1983).
Morphological adaptations of the hooves also depend on the extent of daily movements, which vary based on season and sex. Movement distances increase with group size as adult males tend to be solitary. In contrast, it is hypothesized that nursery groups comprising adult females and vulnerable 1-2-year-olds travel more as an antipredator strategy: larger displacements result in less predictability of their location (Risenhoover & Baley, 1985). Males tend to move less than one kilometer a day, and females between two and five (Singer and Doherty, 1985). In terms of seasonal movements, mountain goats in different locations may or may not migrate depending on the extent of seasonal weather changes. However, minerals are sparse in alpine vegetation and consumption of new-growth vegetation reduces sodium retention (Herbert & Cowan, 1971). Therefore, mountain goats may travel long distances to reach salt licks, through rivers or forests (Feldhamer et al., 2003). It seems that there are no specialist adaptations to the hooves due to their seasonal movements.
Behavioral traits in mountain goats are influenced by their hooves as well. Goats will dig away superficial loose surfaces and deliberately place their hoof in a secure spot when climbing. Mountain goats use their dexterous hooves with a lip to increase their energy intake when foraging in the winter. They have been observed to create craters in snow, enlarging them and pawing to expose moss to eat. Another hoof adaptation applicable to winter results in a decreased body mass track density. Mountain goats achieve this by spreading their rudimentary digits. A large surface area of hooves of mountain goats relative to their mass provides an energetic advantage for locomotion in snow, as they walk more easily on snow crusts. Since dexterous appendages are so important, there is an increased blood flow and circulation to maintain muscular function in their toes in extremely cold temperatures. The consequence of the physiological morphological adaptation is the no-run behavioral trait. High speed locomotion is severely limited due to the higher fat content required for insulation (Dailey, 1991).
An interesting study related to mountain goat hooves was a numerical analysis of a replica. A study of this replica indicated that stiff roll combined with compliant yaw and pitch in certain joints increased the energy needed to slide the hoof by ~20% as compared to the baseline hoof which was stiff at all the joints. The researchers found that the compliant pitch and roll allowed the hoof replica to respond to irregularities in the rough terrain. The researchers likened this to an antilock braking system behavior (Abad et al., 2019). See Figures 16 and 17 below for clarification.

Fig. 16 Anatomy of a mountain goat hoof (Abad et al., 2019).

Fig. 17 Clarification of roll, pitch and yaw of hoof.
Mule deer (Odocoileus hemionus)
Mule deer are native to northwestern North America and live in environments with predominantly rock and dirt surfaces with early-stage vegetation. In this environment, their hooves must be able to withstand the forces incurred when mule deer run to evade predators, chase during the mating season, and jump over obstacles. As seen in Figure 18 below, their hooves have hard, sheeted keratin nails on the outside of their toes to withstand such forces. The keratin nails are also sharp, and mule deer have been observed to kick and stomp on coyotes in self-defense. The inside of the hooves is softer and provides traction on smooth surfaces. The separation between the two digits of each hoof provides space for interdigital scent glands to be placed. These scent glands continuously release scent that rubs onto the ground as mule deer walk. Other deer can follow these scent tracks to locate the deer who secreted them without the need to bleat, which can alert predators. Furthermore, the cloven hoof allows the deer to push off and turn sharply by providing some compliance in fitting in with rough substrates (Ness et al., 2022).
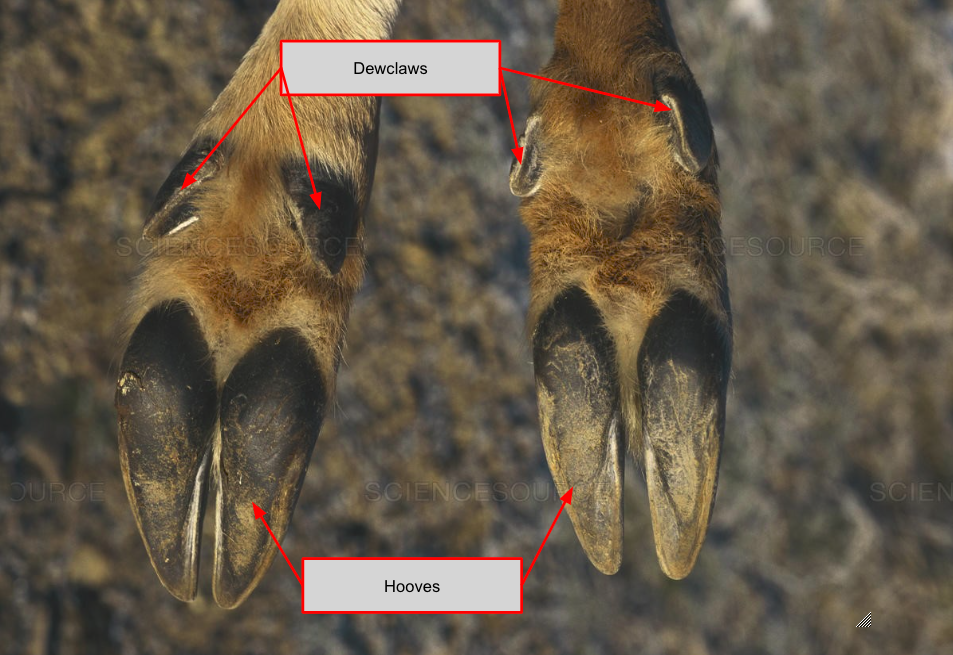
Fig. 18 Mule deer hooves (Adapted from Krasseman, 1997).

Fig. 19 “A Black-tailed deer stotting across a winter field” (Allen, 2016).
Adult male bucks can weigh up to 110kg and females can weigh between 55kg and 80kg (Link, 2004). This variation results in differing antipredator strategies. Whereas most mule deer will flee rather than fight, males are more capable of fighting. The escape gait of Mule deer is the stott as seen in Figures 19 and 20. Striding with its four legs simultaneously, a mule deer can reach high speeds in a biomechanically efficient manner, as energy is conserved between strides (Lingle, 1992). This allows it to outrun predators or chase mates while expending little energy.

Fig. 20 Illustration of a deer stotting (Lingle, 1992).
Thanks to the simultaneous landing of the front and back limbs, stotting mule deer conserve a large proportion of their momentum between strides. This becomes more apparent considering the level position of their bodies during limb contact, paired with a trajectory that is significantly more horizontal than vertical as seen in Figure 20 (Lingle, 1992). Stotting is enabled by the hooves' secure grip on the ground.
Dewclaw track marks are typically seen only if the deer are traveling through mud or snow, and the additional surface area means that the pressure exerted by the deer's weight decreases, thereby making the deer's hooves sink less. Because they sink less, the gait is more efficient as less energy is expended creating a path in viscous substrates. Furthermore, on slick surfaces like mud, snow or dirt the hooves can spread into a V shape preventing the deer from sliding forward (Link, 2004).
Horses (Equus caballus)
This case study will be to understand the importance of the horse's hooves. This is going to be done by understanding how the hooves may affect the horse performance in a horse race. The primary function of the hoof in horses is to support the weight of the animal and protect the sensitive parts of the animal's foot. The expansive and elastic nature of the hoof allows it to prevent concussions on the animal's foot and to distribute the weight of the horse (Hodgson, 2010). The hoof is a type of horn that is always growing, however, the rate of growth depends on many factors, such as temperature, evaporation of moisture, and humidity of the environment (Hodgson, 2010). Nevertheless, the hoof is also being worn at a very different rate than the secretion rate. The attrition rate depends on different factors such as the weight and behaviors of the animal, the nature of the ground (hard or soft, smooth or irregular), the time and distance the animal walks (Hodgson, 2010). All this information tells us that racehorses need to have a long enough hoof to withstand the forces and weights while running on the track. People involved in the race business developed many techniques to keep the hoof in the best condition possible. One of the techniques is to use the horseshoe, which is created by applying iron to only the bottom part of the hoof that contacts the ground. This protective layer of iron protects the hoof (Hodgson, 2010). All racehorses and domestic horses use horseshoes to keep the hoof in good condition. The horseshoes also prevent infections and other types of diseases (Kummer, 2006).
Apart from distributing the horse's weight, the hoof also provides traction to advance. A good condition of the hoof allows the horse to have more traction, hence producing more force and allowing the animal to move forward quicker (Hodgson, 2010).
Firstly, the height of the hoof is important. The hoof is the last part of the horse's toe. Before the hoof, there is a fetlock joint that dictates the movement of the toe. Research has shown that there is an important correlation between the angle at which the hoof is found with respect to the fetlock joint (Taylor, 2022). The angle between the joint and the hoof must be 50 degrees to distribute the animal's weight in the appropriate way. If the angle is higher or lower than 50 degrees, the fetlock joint will be found higher or lower on the leg of the animal. This causes damage to tissues. Such damage is caused because pressure and strain are put on those tissues instead of the hoof, the latter of which is designed to endure such conditions (Taylor, 2022). Horses have four legs, and it is extremely important that the hoof on each leg has the same dimensions, with the appropriate angle. A minimal difference of the length of each hoof would cause an unequal distribution of the animal's weight, putting more strain and pressure on one leg over the other, causing damage over the long term. The importance of the angle between the fetlock and the hoof comes from the fact that the fetlock joint works as a suspension mechanism. Figure 21 is provided to better understand this concept.
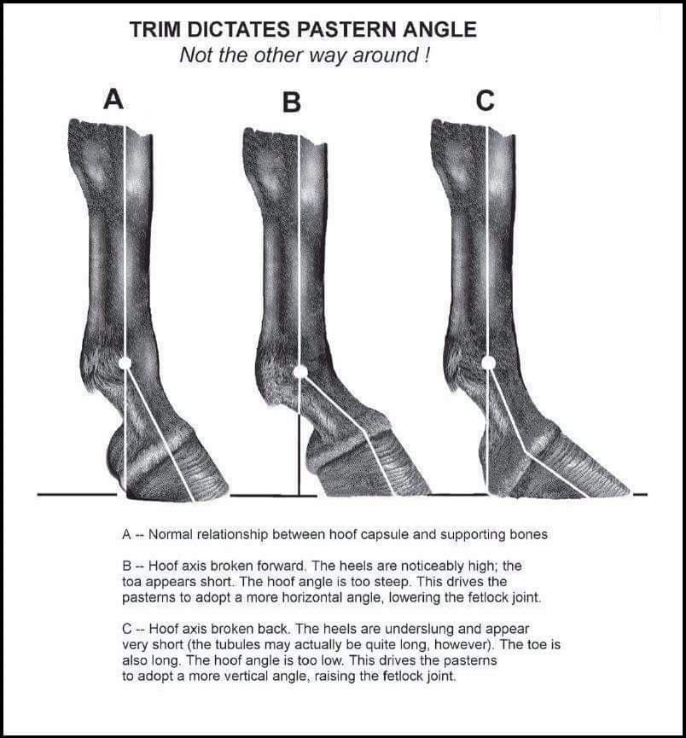
Fig. 21 Importance of the angle between the hoof and the leg (Taylor, 2022).
The second thing to understand is the mechanics behind the stance of the horse, which can be divided in five sections. The first one is the pre-impact phase, which is just immediately before the hoof touches the ground (Thomason et al., 2008). The second phase is the primary impact, where the hoof collides with the ground. Usually, the lateral part of the hoof hits the ground first and then the center contacts the ground, distributing the force of impact over the whole hoof (Thomason et al., 2008). The third phase is the secondary impact, where the weight of the horse starts being distributed over the whole leg and hoof of the horse. At this step, the leg is firmly planted on the ground and the force of impact and weight of the horse are distributed over the hoof and leg. The fourth phase is called the support. In this phase the horse moves to the leg to make a 90-degree angle with the ground. The last phase is the breakover. At this point all forces have almost reached zero, and the horse starts to accelerate in the horizontal direction. Once the hoof leaves the ground the cycle is completed, and on the next step the horse takes, the exact same mechanisms occur (Thomason et al., 2008). Figure 22 is posted below to better understand the mechanism of movement.
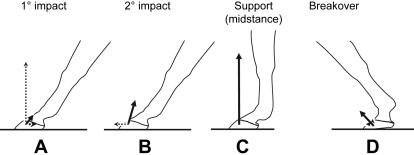
Fig. 22 Mechanism of a horse's stance (Thomason et al., 2008).
A group of scientists have measured the forces and acceleration at distinct stages of this mechanism. This was a direct method to confirm observations and quantify those involved forces, as seen in Figures 23 to 25. However, since these measurements were done in a laboratory, they are not truly representatives of the forces that the hoof receives on racetracks or in natural habitats (Thomason et al., 2008).
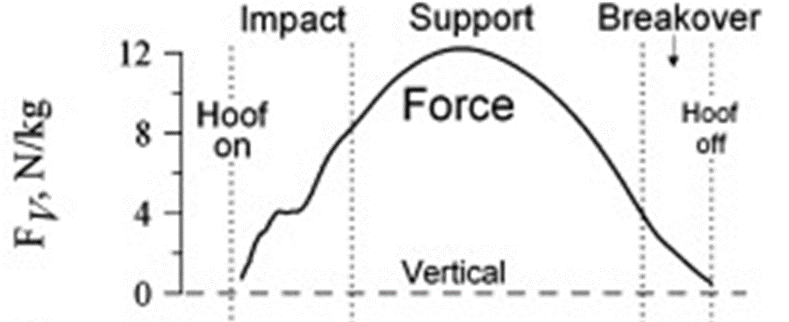
Fig. 23 Force of impact on stance mechanism (Thomason et al., 2008).
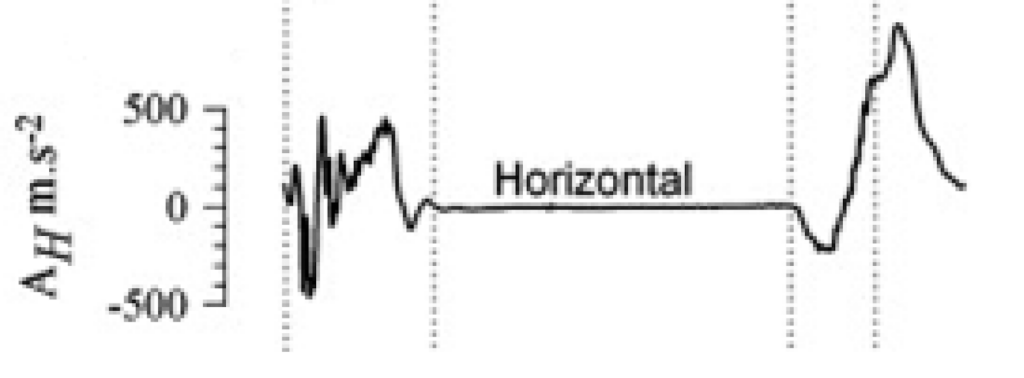
Fig. 24 Horizontal acceleration exerted by the hoof (Thomason et al., 2008).

Fig. 25 Hoof strain on stance mechanisms (Thomason et al., 2008).
In summary, the hoof plays a significant role in the mechanism of movement and stance of the horse. The length and condition of the hoof also impacts the movement and forces the horse can exert. Thus, one method that racehorse owners can maximize their horse's performance is by maintaining the hooves.
Camel (Camelus)
The camel is an animal that lives in one of the harshest environments in the world. The animal is originally from the dry Sahara Desert in North Africa. A photo of a camel's foot is shown below in Figure 26.
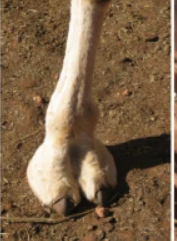
Fig. 26 A camel foot (Clemente et al, 2020).
It can be observed that the camel has two toes on its foot, each toe with a nail. From this image and all other case studies examined on this paper, it is natural to assume that camels also have hooves. However, camels, in fact, do not have hooves (Clemente et al., 2020). Nevertheless, this case study examines how adaptive evolution works and describes how the anatomy of an animal's foot changes based on their habitat, specifically the type of ground encountered.
Camels do not have hooves, but they have fat pad under their feet, as shown in Figure 27.
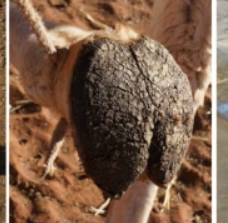
Fig. 27 Camel fat pad (Clemente et al, 2020).
The mechanism of stance of the camel is remarkably like that of the horse. The main difference is that instead of hooves, the fat pads are present. Both fat pads and hooves have the same function of dissipating and reducing mechanical forces such as the force of impact and weight of the animal. However, the main difference is that fat pads provide a greater surface area with the ground (Clemente et al., 2020). Since camels live in the desert, they walk and run in the sand. The fat pads allow the camel's feet to have a higher surface area, dissipating the weight over more area which prevents the camel from sinking into the sandy soil. Also, the higher surface area allows the foot of the camel to be in contact with more sand particles, producing more force once it decides to approach the breakout phase of the stance mechanism (Clemente et al., 2020). Interestingly, when a camel increases its velocity, there is a decrease in the foot pressure (Clemente et al., 2020). A possible explanation is that the fat pad takes advantage of the sand and uses it as a secondary suspension method to dissipate the force and weight of the animal. Note that the response of the fat pad is completely different to that of the hoof, even if the mechanisms are the same.
Knowledge about the mechanisms of hooves can lead to a better understanding of other types of feet, such as the camel's foot pads. In a similar way, this biological and physical knowledge also provides inspiration for developing biomimetic devices and technologies. For instance, a bioinspired robotic hoof design profits off of the “combination of hard and soft materials” of the hoof to increase the traction and versatility of an artificial hoof (Johnson et al, 2018). Hoof properties and mechanics can also allow for technological advancements in prosthetics.
Conclusion
Hooves, whether they are big or small, represent an important part of an animal's body. This portfolio demonstrates that a difference in habitat, especially in the composition of the ground, affects the adaptation of the animal. For the hippopotamus, which is both an aquatic and a terrestrial mammal, the ability to splay its outer digits while swimming or walking in mud increases its fitness in its environment. All hooves protect the tissues and bones within the feet, and for zebras, this protection and the wide frog of their hooves allows them to migrate over long distances in semi-arid savannahs. Giraffes, who also inhabit and migrate around sub-Saharan Africa, have hooves which lengthen their legs, and they have a double pulley astragalus which prevents side-to-side movement of the hoof. Some ungulates, such as moose and mountain goats, have hooves and declaws which can splay under the weight of the animal. In the case of moose, this allows the animal to travel over varied substrates such as mud and snow. For mountain goats, the splaying of their hooves in a V-shape modifies the direction of force and prevents them from slipping on the rocks in their habitat. For mule deer, stotting is a mechanically advantageous form of quadruped movement that is enabled by their sharp, cloven hooves. Clearly, all ungulate hooves provide frictional force so that the animal can walk, but this friction can also wear on the hoof. For horses, horseshoes which are placed under the hoof protect their hooves from attrition. The form and function of hooves can also be understood from the perspective of camels, who do not have hooves, but instead have fat pads. These fat pads act similarly to hooves in that they increase the area interface between the hoof and sand.
Regardless of the substrate upon which the mammal walks, hooves provide some similar physical benefits to all ungulates. At the same time, hooves have different shapes and forms depending on the habitat of the animal, demonstrating the adaptive evolution and vast diversity found in biology. This link means that knowing the habitat, living conditions, and certain physical characteristics, such as the weight, of an ungulate can provide insight into the structure and form of their hooves, and vice-versa. Bioinspired hooves draw from these parallels to deliver innovative technology which can solve challenges in biomimetics and biodevices.
References
Abad, S.-A., Herzig, N., Sadati, S. M. H., & Nanayakkara, T. (2019). Significance of the Compliance of the Joints on the Dynamic Slip Resistance of a Bioinspired Hoof. IEEE Transactions on Robotics, 35(6), 1450–1463. https://doi.org/10.1109/tro.2019.2930864
Alderman, L. Background: Anatomy of a Hoof. Retrieved October 3, 2022, from https://www.irongateequine.com/education/laminitis
Allen, M. (2016). A Black-tailed Deer stotting across a winter field [Review of A Black-tailed Deer stotting across a winter field]. https://www.alamy.com/a-black-tailed-deer-stotting-across-a-winter-field-image235857060.html
Ames, H. (2019). What Are Moose Adaptations? Sciencing. Retrieved September 27, 2022, from https://sciencing.com/moose-adaptations-8521173.html
Artiodactyl (n.d.). Encyclopedia Britannica. Retrieved September 26, 2022, from https://www.britannica.com/animal/artiodactyl/Form-and-function
Basic Care. (n.d.). The Art of Goat Care. Retrieved October 20, 2022, from https://theartofgoatcare.weebly.com/basic-care.html
Baskins, D. 1997. Well-shod: A horse shoeing guide for owners and farriers. Colorado Springs: Western Horseman Magazine.
Basu, C.K., Deacon, F., Hutchinson, J.R., & Wilson, A.M. (2019). The running kinematics of free-roaming giraffes, measured using a low cost unmanned aerial vehicule (UAV). PubMed Central. https://doi.org/10.7717%2Fpeerj.6312
Beeblebrox. (2003). moose tracks in deep snow, Alaska. Retrieved September 27, 2022, from https://commons.wikimedia.org/wiki/File:Tracksdeepsnow.JPG#/media/File:Tracksdeepsnow.JPG
Bergström, Roger & Skarpe, C. (1999). The abundance of large wild herbivores in a semi-arid savanna in relation to seasons pans and livestock. African Journal of Ecology 12–26.
Burnett, C. (2007 05 29). The right-rear foot of a Masai Giraffe. https://commons.wikimedia.org/wiki/File:Masai_Giraffe_right-rear_foot.jpg
Casebeer, R. L., Rognrud, M. J., & Brandborg, S. (1950). The Rocky Mountain goat in Montana. Montana Fish and Game Commission.
Chadwick, D. (1983). A Beast the Color of Winter: The Mountain Goat Observed (p. 51) [Review of A Beast the Color of Winter: The Mountain Goat Observed]. Bison Books.
Clauss, M., Keller, A., Peemöller, A., Nygrén, K., Hatt, J.-M., & Nuss, K. (2009). Postmortal radiographic diagnosis of laminitis in a captive European moose (Alces alces). Schweizer Archiv Fur Tierheilkunde, 151(11), 545–549. https://doi-org.cegep-heritage.idm.oclc.org/10.1024/0036-7281.151.11.545
Clauss, M., Frey, R., Kiefer, B., Lechner-Doll, M., Loehlein, W., Polster, C., Rossner, G. E., & Streich, W. J. (2003). The maximum attainable body size of herbivorous mammals: morphophysiological constraints on foregut, and adaptations of hindgut fermenters. Oecologia, 136(1), 14–27. https://doi.org/10.1007/s00442-003-1254-z
Clemente, C. J., Dick, T. J. M., Glen, C. L., & Panagiotopoulou, O. (2020, March 2). Biomechanical insights into the role of foot pads during locomotion in camelid species. Nature News. Retrieved October 3, 2022, from https://www.nature.com/articles/s41598-020-60795-9
Cumming, David H.M., & Cumming, G. S. (2003). Ungulate Community Structure and Ecological Processes: Body Size, Hoof Area and Trampling in African Savannas. Oecologia, 134(4), 560–568. http://www.jstor.org/stable/4223543
Dadone, L., Foxworth, S., Aruho, R., Schilz, A., Joyet, A., et al. (2021). Foot shape and radiographs of free-ranging Nubian giraffe in Uganda. PLOS ONE 16(12): e0252929. https://doi.org/10.1371/journal.pone.0252929
Dagg A. I. & Foster J. B. (1976) The Giraffe. Its Biology, Behavior and Ecology. Van Nostrand Reinholt, New York.
Dailey, T. (1991). Ecological energetics of mountain goats and bighorn sheep: locomotion and thermoregulation [Review of Ecological energetics of mountain goats and bighorn sheep: locomotion and thermoregulation] https://www.proquest.com/docview/303934278?pq-origsite=gscholar&fromopenview=true
deMaar, T. W. (2000). Normal Hoof Angles and Other Parameters of Selected African Ungualtes. Veterinary Information Network. https://www.vin.com/apputil/content/defaultadv1.aspx?pId=11125&catId=29140&id=3981982
Douglas, J. E., & Thomason, J. J. (2000). Shape, Orientation and Spacing of the Primary Epidermal Laminae in the Hooves of Neonatal and Adult Horses (Equus caballus). Cells Tissues Organs, 166(3), 304–318. https://doi.org/10.1159/000016744
Eltringham, S. K. (1999). The Hippos: Natural History and Conservation. United Kingdom: Academic Press.
Endo, H., Yoshida, M., Nguyen, T. S., Akiba, Y., Takeda, M., & Kudo, K. (2018). Three-dimensional CT examination of the forefoot and hindfoot of the hippopotamus and tapir during a semiaquatic walking. Anatomia, Histologia, Embryologia, 48(1), 3–11. https://doi.org/10.1111/ahe.12405
Feldhamer, G. A., Bruce Carlyle Thompson, & Chapman, J. A. (2003). Wild mammals of North America : biology, management, and conservation. Johns Hopkins University Press.
Festa-Bianchet, M., Urquhart, M., & Smith, K. G. (1994). Mountain goat recruitment: kid production and survival to breeding age. Canadian Journal of Zoology, 72(1), 22–27. https://doi.org/10.1139/z94-004
Even vs. Odd toed Ungulates.png – UBC Wiki. (2012). Wiki.ubc.ca. https://wiki.ubc.ca/File:Even_vs._Odd_toed_Ungulates.png
Garman, A. : J. (2021, September 3). How to Prevent Foot Rot in Goats. Backyard Goats. https://backyardgoats.iamcountryside.com/health/how-to-prevent-foot-rot-in-goats/
Geist. V. (2022). Moose. Encyclopedia Britannica. https://www.britannica.com/animal/moose-mammal
Groves, C. P., & Grubb, P. (2011). Ungulate taxonomy. Johns Hopkins University Press.
Grzimek's Animal Life Encyclopedia. (n.d.). Okapis and Giraffes (Giraffidae). https://www.encyclopedia.com/environment/encyclopedias-almanacs-transcripts-and-maps/okapis-and-giraffes-giraffidae
Hebert, D., & Cowan, I. M. (1971). Natural salt licks as a part of the ecology of the mountain goat. Canadian Journal of Zoology, 49(5), 605–610. https://doi.org/10.1139/z71-097
Hjeljord, O. (1973). Mountain Goat Forage and Habitat Preference in Alaska. The Journal of Wildlife Management, 37(3), 353. https://doi.org/10.2307/3800126
Hodgson, J. T. (2010). Art of preserving and defending the foot of the horse: Deduced mathematically from the .. Nabu Press.
Hundertmark, K., Eberhardt, W.L., & Ball, R.E. (1990). Alces. Vol 26. pp. 108-114. https://www.adfg.alaska.gov/static/home/library/pdfs/wildlife/research_pdfs/alces/6023.pdf
Jantz, B., & Neumann, D. (1998). Growth and reproductive cycle of the zebra mussel in the River Rhine as studied in a river bypass. Oecologia, 114(2), 213–225. https://doi.org/10.1007/s004420050439
Jantz, B., & Neumann, D. (1998). Growth and reproductive cycle of the zebra mussel in the River Rhine as studied in a river bypass. Oecologia, 114(2), 213–225. https://doi.org/10.1007/s004420050439
Johnson, A., Singhasaneh, N., Bays, P. (2018). Bioinspired Hoof Design. Retrieved on October 23, 2022 from https://www.andrew.cmu.edu/user/amj1/papers/DW2018_Hoof.pdf.
Keller, A., Clauss, M., Muggli, E., & Nuss, K. (2009). Even-toed but uneven in length: the digits of artiodactyls. Zoology (Jena, Germany), 112(4), 270–278. https://doi.org/10.1016/j.zool.2008.11.001
Kelsall, J. P. (1969). Structural Adaptations of Moose and Deer for Snow. Journal of Mammalogy, 50(2), 302–310. https://doi.org/10.2307/1378347
Klappenbach, Laura. (2020, August 25). Even-Toed Hoofed Mammals. Retrieved from https://www.thoughtco.com/even-toed-hoofed-mammals-130019
Krassemann, S. J. (1997). Mule deer hooves (Odocoileus hemionus), Flathead National Forest, Montana. [Review of Mule deer hooves (Odocoileus hemionus), Flathead National Forest, Montana.].
Kummer, M., Geyer, H., Imboden, I., Auer, J., & Lischer, C. (2006). The effect of hoof trimming on radiographic measurements of the front feet of normal warmblood horses. The Veterinary Journal, 172(1), 58–66. https://doi.org/10.1016/j.tvjl.2005.03.008
Leśniak, K., Whittington, L., Mapletoft, S., Mitchell, J., Hancox, K., Draper, S., & Williams, J. (2019). The Influence of Body Mass and Height on Equine Hoof Conformation and Symmetry. Journal of Equine Veterinary Science, 77, 43–49. https://doi.org/10.1016/j.jevs.2019.02.013
Lewinson, R. T., & Stefanyshyn, D. J. (2016). A descriptive analysis of the climbing mechanics of a mountain goat (Oreamnos americanus). Zoology, 119(6), 541–546. https://doi.org/10.1016/j.zool.2016.06.001
Librado, P., Khan, N., Fages, A., Kusliy, M. A., Suchan, T., Tonasso-Calvière, L., Schiavinato, S., Alioglu, D., Fromentier, A., Perdereau, A., Aury, J.-M., Gaunitz, C., Chauvey, L., Seguin-Orlando, A., Der Sarkissian, C., Southon, J., Shapiro, B., Tishkin, A. A., Kovalev, A. A., & Alquraishi, S. (2021). The origins and spread of domestic horses from the Western Eurasian steppes. Nature, 598(7882), 634–640. https://doi.org/10.1038/s41586-021-04018-9
Lingle, S. (1992). Escape Gaits of White-Tailed Deer, Mule Deer and Their Hybrids: Gaits Observed and Patterns of Limb Coordination. Behaviour, 122(3-4), 153–181. https://doi.org/10.1163/156853992×00499
Lundmark, C. & Ball, J.P. (2008). Living in Snowy Environments: Quantifying The Influence of Snow on Moose Behavior, Arctic, Antarctic, and Alpine Research. 40:1, 111-118, https://doi.org/10.1657/1523-0430(06-103)[LUNDMARK]2.0.CO;2
Marshall, P. J., & Sayer, J. A. (1976). Population Ecology and Response to Cropping of a Hippopotamus Population in Eastern Zambia. Journal of Applied Ecology, 13(2), 391–403. https://doi.org/10.2307/2401788
McHorse, B. K., Biewener, A. A., & Pierce, S. E. (2017). Mechanics of evolutionary digit reduction in fossil horses (Equidae). Proceedings of the Royal Society B: Biological Sciences, 284(1861), 20171174.
Mijele, D., Iwaki, T., Chiyo, P. I., Otiende, M., Obanda, V., Rossi, L., Soriguer, R., & Angelone-Alasaad, S. (2016). Influence of Massive and Long Distance Migration on Parasite Epidemiology: Lessons from the Great Wildebeest Migration. EcoHealth, 13(4), 708–719. https://doi.org/10.1007/s10393-016-1156-2
Moose. (n.d.). Department of Inland Fisheries & Wildlife. Retrieved September 27, 2022, from https://www.maine.gov/ifw/fish-wildlife/wildlife/species-information/mammals/moose.html#:~:text=The%20total%20length%20of%20a,3%20inches%20for%20a%20calf
Moose. (n.d.). National Geographic. Retrieved September 27, 2022 from https://www.nationalgeographic.com/animals/mammals/facts/moose
Mule Deer | National Wildlife Federation. (2019). National Wildlife Federation. https://www.nwf.org/Educational-Resources/Wildlife-Guide/Mammals/Mule-Deer
Naidoo, R., Chase, M., Landen, K., Beytell, P., du Preez, P., Stuart-Hill, G., & Taylor, R. (2016). Namibia and Botswana's zebra migration: still the longest, but for how long? A reply to Schapira et al. Oryx, 51(1), 20–20. https://doi.org/10.1017/s0030605316000867
Ness, A., Jacob, A., Saboraki, K., Otero, A., Gushue, D., Martinez Moreno, D., de Peña, M., Tang, X., Aiken, J., Lingle, S., & McKenzie, D. (2022). Cellular prion protein distribution in the vomeronasal organ, parotid, and scent glands of white-tailed deer and mule deer. Prion, 16(1), 40–57. https://doi.org/10.1080/19336896.2022.2079888
Novellie, P. A. (1994). Monitoring the condition of mountain zebra habitat in the Mountain Zebra National Park. Koedoe, 37(1). https://doi.org/10.4102/koedoe.v37i1.324
Nowak, R. D., & Figueiredo, M. A. (1999, October). Unsupervised progressive parsing of Poisson fields using minimum description length criteria. In Proceedings 1999 International Conference on Image Processing (Cat. 99CH36348) (Vol. 2, pp. 26-30).
Outdoors, N. (2018, August 10). Deer Hooves… More Than Meets The Eye. N1 Outdoors®. https://n1outdoors.com/deer-hooves/
Owen-Smith, N., Mills, M.G.L. (2008). Predatory-prey size relationships in an African large-mammal food web. Journal of Animal Ecology. http://dx.doi.org/10.1111/j.1365-2656.2007.01314.x
Owen-Smith, N., & Chafota, J. (2012). Selective feeding by a megaherbivore, the African elephant (Loxodonta africana). Journal of Mammalogy, 93(3), 698–705. https://doi.org/10.1644/11-mamm-a-350.1
Perich, Shawn; Furman, Michael (2003). Whitetail Hunting: Top-notch Strategies for Hunting North America's Most Popular Big-Game Animal. Creative Publishing international. pp. 8, 9
Peziol, M. (2017). A bull moose front foot. There is wear on the declaws and the toes are asymmetrical. Retrieved on October 23, 2022 and adapted from https://www.wildernesscollege.com/moose-habitat.html.
Prothero, D.R. (2009). Evolutionary Transitions in the Fossil Record of Terrestrial Hoofed Mammals. Evo Edu Outreach, https://doi.org/10.1007/s12052-009-0136-1
Raji, A. R., Sardari, K., & Mohammadi, H. R. (2008). Normal Cross – Sectional Anatomy of the Bovine Digit: Comparison of Computed Tomography and Limb Anatomy. Anatomia, Histologia, Embryologia: Journal of Veterinary Medicine Series C, 37(3), 188–191. https://doi.org/10.1111/j.1439-0264.2007.00825.x
Reidenberg, J. S. (2007). Anatomical adaptations of aquatic mammals. The Anatomical Record: Advances in Integrative Anatomy and Evolutionary Biology, 290(6), 507–513. https://doi.org/10.1002/ar.20541
Risenhoover, K. L., & Bailey, J. A. (1985). Relationships between group size, feeding time, and agonistic behavior of mountain goats. Canadian Journal of Zoology, 63(11), 2501–2506. https://doi.org/10.1139/z85-370
Roland, E., Stover, S. M., Hull, M. L., & Dorsch, K. (2003). Geometric symmetry of the solar surface of hooves of Thoroughbred racehorses. American Journal of Veterinary Research, 64(8), 1030–1039. https://doi.org/10.2460/ajvr.2003.64.1030
Samuel, B., & Macgregor, W. G. (). Proceedings of the first International Mountain Goat Symposium, Kalispell, Montana, February 19, 1977. Fish and Wildlife Branch.
Shorrocks, B. (2016). The Giraffe: Biology, ecology, evolution, and behaviour. John Wiley & Sons, Ltd. https://doi.org/10.1002/9781118587430
Singer, F. J., & Doherty, J. L. (1985). Movements and habitat use in an unhunted population of mountain goats, Oreamnos americanus. [Ottawa Field-Naturalists' Club].
Solounias N, Danowitz M (2016) Astragalar Morphology of Selected Giraffidae. PLoS ONE 11(3): e0151310. https://doi.org/10.1371/journal.pone.0151310
Stieger-Vanegas, S. (2015). 3D model from a set of CT images obtained using a 64-slice CT scanner of the disarticulated extremities of a 22 year old. https://www.youtube.com/watch?v=Kg175vUv-rY&ab_channel=Andr%C3%A9sAlejandroCastroCort%C3%A9s
SurprisePally. (2008). Mountain goat climbing [Review of Mountain goat climbing]. https://www.flickr.com/photos/31417716@N00/2690921109
Tacchio, G., Davies, H.M.S., Morgante, M. & Bernardini, D. (2010). A radiographic technique to assess the longitudinal balance in front hooves. Equine Veterinary Journal, 34(S34), 368–372. https://doi.org/10.1111/j.2042-3306.2002.tb05450.x
taxonomy. (2020). Taxonomy browser (Hexaprotodon liberiensis). Nih.gov. https://www.ncbi.nlm.nih.gov/Taxonomy/Browser/wwwtax.cgi?id=56798
Taylor Fabus, (2022, January 21). What your horse's hoof angle may be telling you. Michigan State University Extension. Retrieved October 3, 2022, from https://www.canr.msu.edu/news/what-your-horse-s-hoof-angle-may-be-telling-you
Telfer, E. S., & Kelsall, J. P. (1984). Adaptation of Some Large North American Mammals for Survival In Snow. Ecology, 65(6), 1828–1834. https://doi.org/10.2307/1937779
Thomason, J. J., & Peterson, M. L. (2008). Biomechanical and mechanical investigations of the hoof-track interface in racing horses. Veterinary Clinics of North America: Equine Practice, 24(1), 53–77. https://doi.org/10.1016/j.cveq.2007.11.007
Ungualte. (2009). New World Encyclopedia. https://www.newworldencyclopedia.org/p/index.php?title=Ungulate&oldid=918634
Universität Basel. (2014, June 18). Evolutionary biology: Why cattle, pigs are even-toed. ScienceDaily. Retrieved September 22, 2022 from www.sciencedaily.com/releases/2014/06/140618131927.html
Wolanski, E., Gereta, E., Borner, M., & Mduma, S. (1999). Water, migration and the Serengeti ecosystem. American Scientist, 87, 526-533.