Chemical Mechanisms of Resonating Devices in Animals for Communication and Information Reception
Archinlin Wang, Henry Stephenson, Syphax Ramdani, Zachary Flynn
Abstract
Acoustic resonance is a highly effective method of communication and information reception in the animal kingdom, pervasively employed by creatures as small as the 4mm Nuctenea sclopetaria spider and as large as the colossal Orcinus orca (killer whale). Resonance is often achieved either via specialized organs or an extended phenotype. These biologically engineered machines are known as resonating devices. Their functions are diverse, ranging from ultrasonic whistles for communication and navigation in orcas to mating calls in cicadas. In general, the functions of resonance can be summarized as methods to provide species with a greater awareness of their surrounding environment through vibratory sensory modes. This allows animals to rapidly adapt to variable conditions, granting them survival advantage. This analysis aims to describe resonating devices through a chemical lens, demonstrating the biochemical, protein, mechanical, and biomaterial engineering techniques that have been developed through evolution in four unique animal examples. This review opens with a general discussion of the organ of Corti common to most mammals, then explores more specific examples, such as ultrasonic whistles and their reception in killer whales and echolocation in bats. Thereafter, examples of resonance in arthropods are examined, including the chemical mechanism for cicada mating calls and protein crystallization modulation in spider silk. The significance and prevalence of resonating devices in coordination, hunting, reproduction, and sociality for several major phyla of the animal kingdom are demonstrated.
Introduction
This review concentrates on resonating devices within animals from a chemical perspective, particularly on the microscopic interactions of cells, proteins, amino acids, and nucleotides, demonstrating the often hierarchical nature of chemical systems. There are two primary focuses. First, a background on mammalian uses of resonance for auditory sensory modes is provided and unique examples are considered. Second, the uses of resonance in the arthropod phylum are discussed. To begin, the cochlea is explored as a crucial auditory organ which serves as the primary tool for converting sound waves into information. Mechanotransduction is examined at the cellular level, including ion transport, synaptic transmission, cochlear amplification, and adaptability. Additionally, the paper touches on why the structure of the prestin protein is key to auditory sensing. Following that, specific examples in aquatic mammals and bats are considered. The paper demonstrates that toothed whales and bats have comparable evolution of echolocation, indicated by their similar genetic developments. Furthermore, there is evidence that this evolutionary convergence has also occurred between laryngeal echolocating bats and dolphins.
In the latter half of the review, the chemical mechanisms of resonating devices in arthropods are discussed. Cicadas use chitin, chitosan, and resilin to create a biocomposite which induces mechanical changes that optimize resonance in their mating calls. This demonstrates how evolution has enabled cicadas to become exceptional biomaterial engineers. In the Araneae class, many web-weaving spiders have been shown to use resonance to hunt, perform courtship rituals, and evade predation. Spiders have fine-tuned protein processing capacities; they create an ideal silk material using pH, strain rate, and ion concentrations in their specialized silk glands in order to amplify certain biologically relevant vibrations and attenuate others. Spiders manipulate these parameters to synthesize different silks depending on the intended use and current environmental conditions. The examples in this review elucidate the frequent convergence of evolution towards the most optimal solution, but also the beauty of natural selection in engineering simple yet elegant biological machines.
Mechanotransduction in the mammalian organ of corti
The mechanisms employed by mammals to hear sounds are complex and have been a popular subject for scientific investigation. Until sound reaches the organ of Corti, these mechanisms are fairly well understood. However, there are still many questions regarding the principle of mechanotransduction (MT), or the conversion of a mechanical signal to a biochemical signal, which occurs in the organ of Corti (Brown, 2013). After an introduction to ear anatomy and the journey of sound to the organ of Corti, this section gives a detailed look into mechano-transduction at the cellular level. Particularly, ion transport, synaptic transmission, cochlear amplification, and adaptation will be explored. It is important to note that much of this research is recent and ongoing.
Ear anatomy and sound transmission: a brief overview
The organ of Corti is situated in the cochlea within the inner ear. Before sound can reach this organ, it must pass through various structures (illustrated in Figures 1 and 2). The ear canal leads to the tympanum, which lies at the border between the outer and middle ear. The middle ear has three auditory ossicles: the malleus, incus, and stapes. The stapes is connected via the oval window to the cochlea, an inner ear structure separated into three chambers (the scala vestibuli at the top, scala media in the middle, and scala tympani at the bottom). Together, the chambers are arranged in a tube which coils in a snail-like fashion. The scala vestibuli and scala tympani are linked at the apex by the helicotrema and both contain a liquid called perilymph. The scala media contains the organ of Corti and is filled with a liquid known as endolymph. A particularly important structure is the basilar membrane separating the scala tympani and scala media; the organ of Corti lies on this membrane (Brown, 2013).
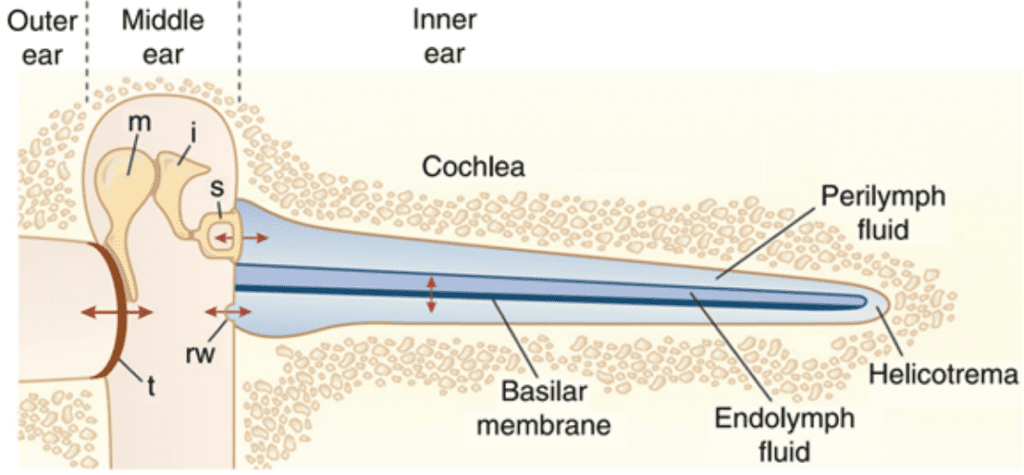
Fig. 1 Representation of ear transmission from the ear cannal to the cochlea. The cochlea is represented unwound. Arrows show oscillatory motion at different steps of transmission. t: tympanum, m: malleus, i: incus, s: stapes, rw: round window (Fettiplace, 2017).
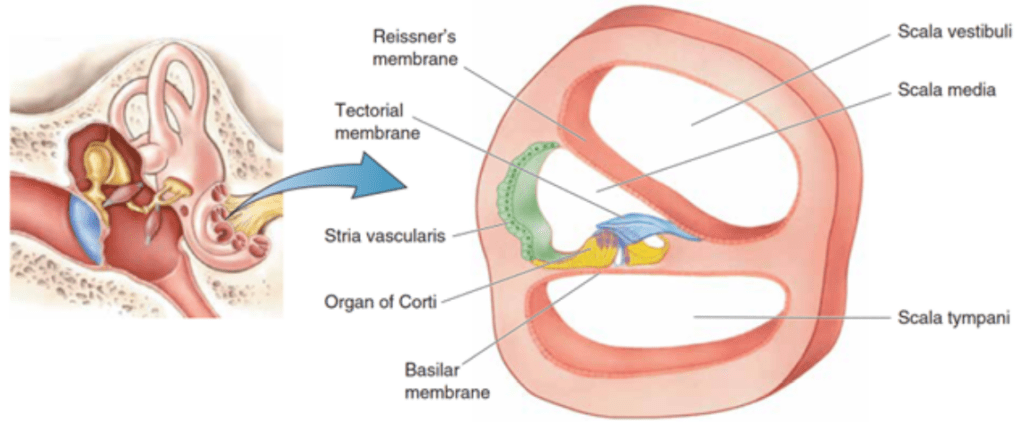
Fig. 2 Cross-section of the three scalae of the cochlea and some structures within (Bear et al., 2015).
Sound waves travel through the ear canal and reach the tympanum, causing it to vibrate. These vibrations cause oscillatory motion of the auditory ossicles, which causes the stapes to act as a piston on the oval window. This piston-like motion produces sound waves in the perilymph, which causes the basilar membrane to vibrate. The basilar membrane's resonant frequency changes along its length such that each frequency causes maximal vibrations only at some position along the membrane. Each frequency is thus coded to a specific region of the cochlea (Brown, 2013).
A model for mechanotransduction
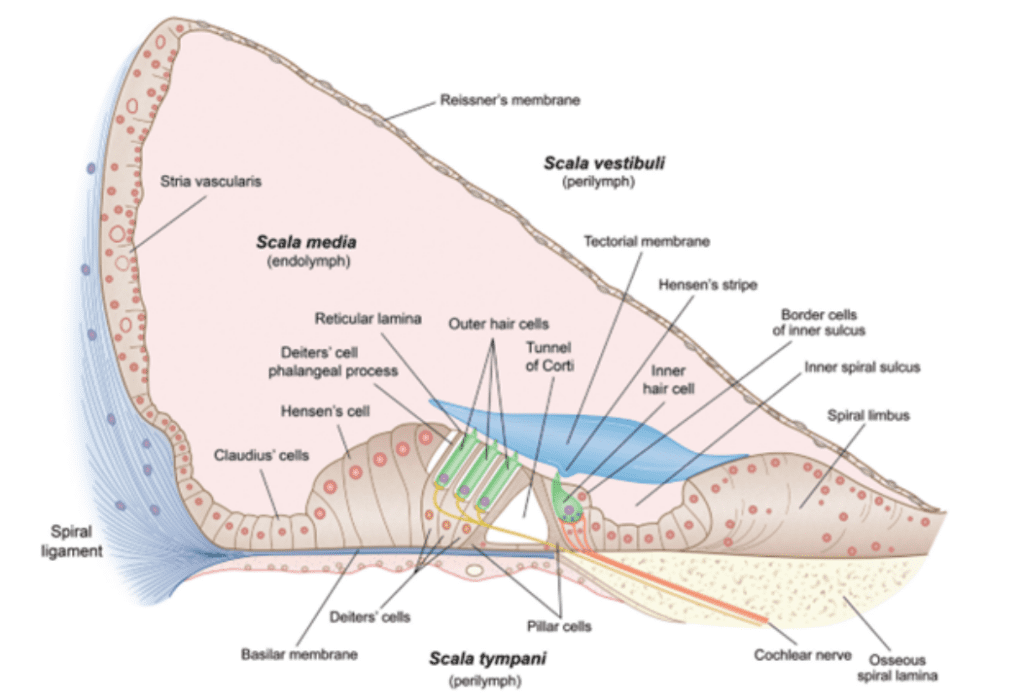
Fig. 3 Cross-section of scala media and organ of Corti (Fettiplace, 2017).
The organ of Corti lies at the bottom of the scala media (see Figure 3). It contains multiple rows of outer hair cells (OHCs) and inner hair cells (IHCs), as well as many supporting cells. The hair cells (HCs) are named after the hair-like stereocilia which lie on their apical (top) side and are crucial to MT. Figure 4 shows a typical mammalian HC. Interestingly, IHCs receive over 90% of the afferent (going to the brain) innervation, while OHCs receive around 90% of the efferent (coming from the brain) innervation. This leads to the theory that IHCs are the cells responsible for MT, while OHCs are responsible for cochlear amplification (Fettiplace, 2017; McPherson, 2018).
The ionic composition in and around the HC is integral to the MT process. The HC is exposed to two different fluids: the endolymph on the apical side, and the perilymph on the basolateral (bottom and sides) side. The typical ionic composition of the endolymph, perilymph, and HC cytosol are summarized in Table 1. Hair cells are moderately depolarized at -40 to -60 mV, and the endolymph is about 80-100 mV more positive than the perilymph (Fettiplace, 2017; McPherson, 2018).
Endolymph | HC Cytosol | Perilymph | |
K+ | 150 | 125-140 | 4 |
Ca2+ | 0.02 | negligible | 1.3 |
Na+ | 1 | 10-15 | 150 |
Cl– | 130 | 1.5-4 | 120 |
Transduction starts at the tips of the stereocilia of the HCs. Stereocilia are organized in rows of increasing height and the tips of shorter stereocilia are connected by tip links to their taller neighbours. The stereocilia tips also contain ion channels which open as tension in the tip links increases (Fettiplace, 2017; McPherson, 2018; Xu, 2018). This channel, referred to as the MT channel, has yet to be conclusively identified, but the best candidate is currently TMC1 (McPherson, 2018). It is a large, nonspecific cation channel with unusually large conductance compared to other cation channels (over 100 pS, as compared to 30 pS for a common cation channel like the acetylcholine receptor). When open, it mostly transports potassium ions and some calcium ions, which move down a concentration and voltage gradient from the endolymph to the cytosol. Potassium ions can then move down a concentration gradient from the cytosol to the perilymph, which means that the HC does not have to expend energy to move ions, making this process faster. Instead, the ionic concentrations in the endolymph and perilymph are maintained by the stria vascularis on the border of the scala media (Fettiplace, 2017).
When there are no sound waves, stereocilia stand upright and the resting tension of tip links causes only some channels to open. When sound waves move stereocilia in the direction of longer stereocilia, tension increases in the tip links, increasing the number of open channels. The influx of positive ions causes depolarization of the cell. When stereocilia are moved the opposite way, however, tension decreases and more channels are closed, leading to hyperpolarization (Xu, 2018). PIEZO2, a key protein in cutaneous mechanoreceptors, also creates a current when stereocilia move the opposite way (Beurg & Fettiplace, 2017). PIEZO2's function in hearing is still unknown (McPherson, 2018).
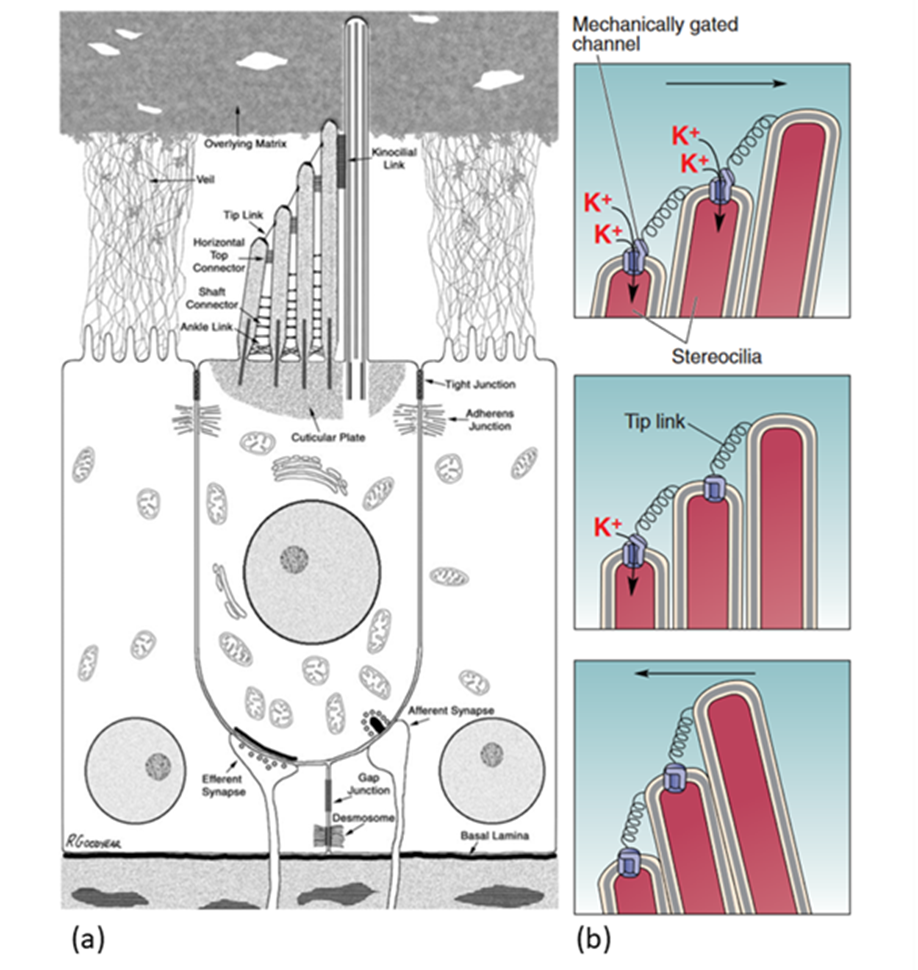
Fig. 4 (a) Diagram of a stereotypical mammalian hair cell. The kinocilium (rightmost hair-like structure on the apical side of the hair cell) is not present in mature cochlear hair cells. (b) Model of MT channel opening and closing. The probability of channel opening depends on the movement of stereocilia (Adapted from Bear et al., 2015; McPherson, 2018).
Synaptic transmission at the ribbon synapse
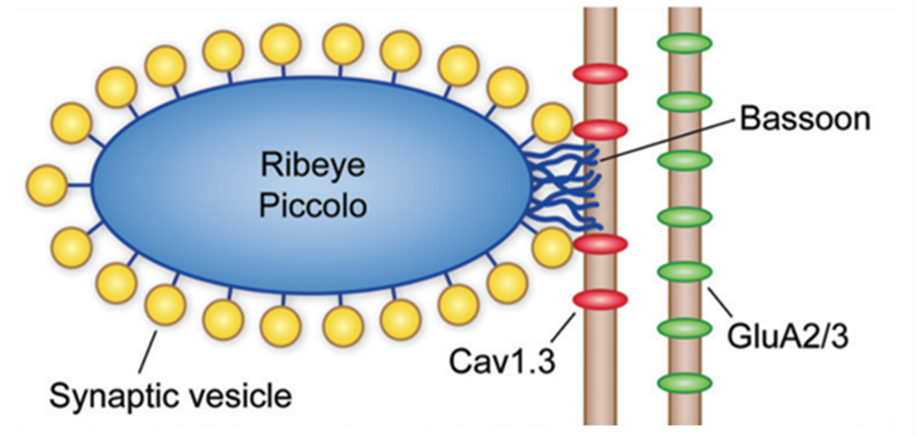
Fig. 5 Schematic of IHC ribbon synapse. Ribeye, piccolo, and bassoon are proteins known to play a part in the structure and function of the ribbon synapse. Cav1.3 channels are positioned close to the synaptic ribbon, and AMPA (GluA2/3) receptors are present on the afferent neuron (Fettiplace, 2017).
Afferent synapses in the IHCs, called ribbon synapses (see Figure 5), must rapidly send a large volume of signals. To achieve this, a specialized electron-dense ovoid surrounded by synaptic vesicles, called synaptic ribbons, is visible on the basal side of IHCs. Cav1.3 voltage-gated calcium ion channels positioned close to the ribbon open as the cell depolarizes. The relatively high calcium concentration in the perilymph allows for an influx of calcium ions which interact with the ribbon to cause exocytosis of the glutamate neurotransmitter contained within the synaptic vesicles. This neurotransmitter travels to AMPA receptors on the afferent neuron, triggering action potentials. The mechanism for glutamate release is not well understood but is possibly mediated by the calcium-binding protein otoferlin. The ribbon synapse has several characteristics which distinguish it from other neuronal synapses. To start, release of glutamate is not caused by an action potential but by a graded change in membrane potential. As the cell gets depolarized, more and more glutamate is released. Additionally, glutamate is constantly released even at resting potential, causing spontaneous neuron activation. Depolarization only increases the release of glutamate. Finally, the synapse is said to be indefatigable, as it can maintain neurotransmitter release for sustained stimuli without depression (Fettiplace, 2017; McPherson, 2018).
Cochlear amplification
As previously stated, it is believed that OHCs do not send signals to the brain. Indeed, they receive mostly efferent innervation. Additionally, they can lengthen and shorten at frequencies of up to 80 kHz when their membrane potential changes. This ability has been named electromotility. Because of this, it is believed that OHCs vibrate in response to incoming sound waves, matching them and thereby amplifying them. Otoacoustic emissions, sounds which are emitted by the ear in response to incoming sounds, provide strong evidence for this (Brown, 2013; Fettiplace, 2017).
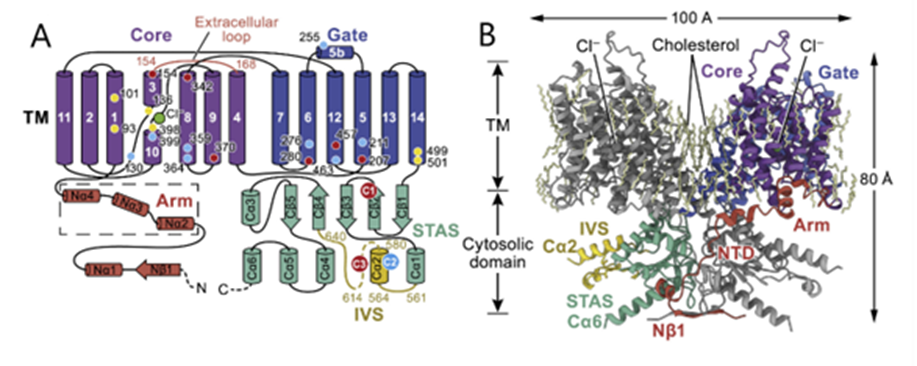
Fig. 6 Schematic (A) and structural model (B) of prestin. Key domains are colored on one protomer, and residues affecting prestin electromotility are shown with a yellow, blue, or red dot and numbered in (A). TM indicates the transmembrane portion (Adapted from Ge et al., 2021).
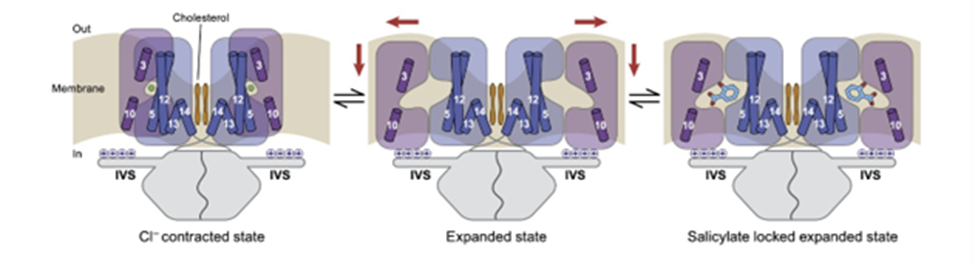
Fig. 7 Mechanism of prestin electromotility. Chloride causes prestin to shift into a contracted state. When chloride is unbound, the protein expands. Salicylate can be used in experiments to lock prestin into its expanded state (Ge et al., 2021).
Prestin, a dimeric protein embedded in the lateral section of the OHC plasma membrane, has been identified as being responsible for the electromotility of the OHCs (see Figures 6 and 7 on prestin). Recent studies point towards a possible mechanism through which prestin senses voltage and changes conformation, causing movement. Each prestin monomer contains an anion-binding pocket, to which chloride ions are bound when the cell is at rest. Binding of chloride ions causes the protein to be in its most compact conformation. Prestin contains intrinsic voltage-sensing residues. When the cell is depolarized, the residues cause unbinding of chloride ions and a change of conformation of prestin to an expanded state. The high concentration of prestin in the OHC membrane leads to elongation of the membrane (Ge et al., 2021). Notably, prestin is also sensitive to membrane tension. Increased tension may cause decreased affinity for chloride in the anion-binding pocket, which may act as a regulation mechanism (Homma & Dallos, 2010).
Efferent innervation
OHCs possess nonselective cation channels which open when bound to the acetylcholine neurotransmitter and have particularly high permeability for calcium ions. When efferent neurons release acetylcholine, calcium ions travel inside the cell and bind to calcium-dependent potassium channels, which allows for an outward flow of potassium ions, hyperpolarizing the cell. This hyperpolarization inhibits prestin action and thus reduces cochlear amplification. It has been proposed that this mechanism exists to protect OHCs from damage caused by particularly loud sounds (Fettiplace, 2017; McPherson, 2018).
Adaptation
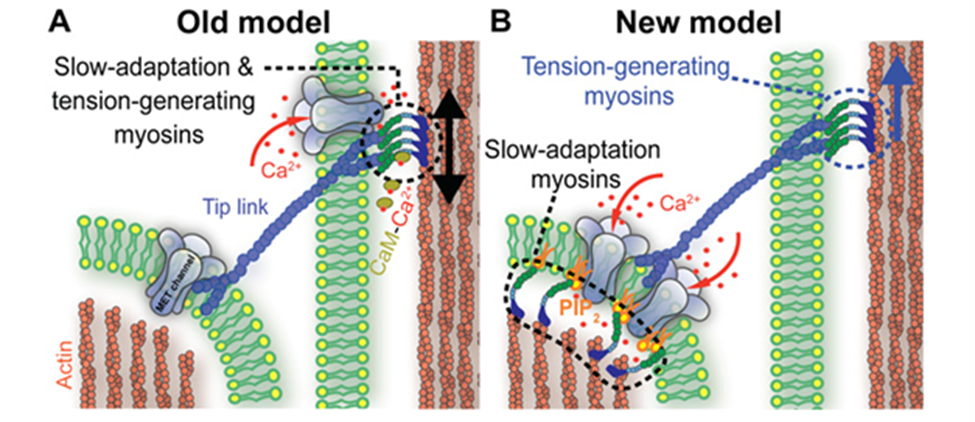
Fig. 8 Old (A) and new (B) models of slow adaptation. In (A), MT channels appear at both ends of the tip links. Myosin motors on the taller stereocilia generate tip link tension and interact with calcium to slide along it. In (B), myosin motors on both ends of the tip links are different. On the lower end, they interact with PIP2 to inhibit MT channels. There is no consensus as to whether either model is correct (Caprara et al., 2020).
As in other sensory receptors, a key role of HCs is adaptation, or the attenuation of sustained, constant stimuli to allow for greater sensitivity to changes in the environment. It is believed that two models underpin adaptation in HCs: fast adaptation, which activates in one millisecond or sub-millisecond time scales, and slow adaptation, which works in time scales of tens to hundreds of milliseconds (Fettiplace, 2018). Both models are dependent on calcium ion entry through the MT channel. In fast adaptation, calcium ions directly interact with the MT channel or a connected protein, which inhibits its function (Peng, 2013). In slow adaptation, calcium ions cause myosin movement in the upper part of the tip link, allowing it to slide down to reduce tension (Caprara et al., 2020). However, these models are still controversial, especially for mammals, and the details of their mechanisms are still unknown (Caprara et al., 2020; Fettiplace, 2018; Peng, 2013). A more recent model for slow adaptation proposes that the process occurs close to the lower end of the tip link, where myosin works in conjunction with phosphatidylinositol 4,5-bisphosphate (PIP2) to inhibit the MT channel. The old model is compared with the new model in Figure 8. PIP2 was shown to decrease MT channel conductance, providing some proof for this model (Caprara et al., 2020). Other mechanisms for slow and fast adaptation, as well as alternatives beyond slow and fast adaptation, have been proposed as well (Caprara et al., 2020; Peng, 2013).
Summary
Mechanotransduction in mammalian hair cells is a complex phenomenon, and this section gave a broad overview of some of its processes. The stereocilia of HCs are connected by tip links. The tension in these tip links opens MT channels at the ends of the stereocilia, which creates an inward flow of potassium and calcium ions, depolarizing the cells. In IHCs, depolarization causes the opening of voltage-gated calcium ion channels, which strengthen the release of neurotransmitters by the synaptic ribbon to the afferent neuron. In OHCs, depolarization is detected by intrinsic voltage sensors in the membrane-bound prestin molecules, causing its expansion, and therefore amplification of the sound. Multiple models for adaptation have been proposed, the most prominent of which are fast and slow adaptation, which rely on calcium ions to inhibit MT channel activity and reduce tip link tension, respectively. Though significant progress has been made in our understanding of cochlear mechanotransduction, many questions remain, including the identity and characteristics of the MT channel.
Convergent evolution of echolocation phenomena in bats and toothed whales
In terms of size, the flying bat and diving toothed whale (Odontoceti), or dolphin, are mammalian polar opposites, yet both make use of echolocation as a method of communication, navigation, and most importantly predation. Thus, the genetic convergence that determines their echolocation is an intriguing topic. The echolocation abilities of bats and dolphins are a famous example of parallel development through natural selection. Two genera of bats—Yangochiroptera and Rhinolophidae— as well as Odontoceti are capable of echolocation by the use of ultrasound. This intricate bio-sonar system helps in navigation and feeding for these mammals (Shen et al., 2012).
Some discrepancies in echolocation behavior and signal structure between toothed whales and bats are a result of variances in the medium density in which they operate. Water is denser than air, which means that sound travels five times faster in water and attenuates much slower (loses energy at a decreased rate). This section discusses the remarkable genetic similarities in echolocation behavior between bats and toothed whales, despite their distinct habitats. The transition to the current lifestyles of bats and toothed whales necessitated significant modifications in movement patterns, possibly coincident with the evolution of echolocation (Fenton et al., 2014).
As mentioned in the section on Mechanotransduction in Mammalian Organ of Corti, the Organ of Corti has been the focus of most echolocation-related molecular studies, and the prestin motor protein plays a crucial role in voltage motility in this organ (Fettiplace, 2017). Prestin is a member of the anion transporting SLC26 family and is largely located on the membrane of cochlear outer hair cells (OHCs). It has intracellular amino and carboxyl termini, approximately ten transmembrane domains, and several intracellular and extracellular loops (Navaratnam et al., 2005). Prestin supplies the electromobility of OHCs believed to be important for cochlear amplification, an active process that confers sensitivity and frequency selectivity on the auditory system of mammals (Dallos, 2008; Li et al., 2010).
Prestin amino acid sequence convergence appears to have occurred between bats and dolphins, as well as within laryngeal echolocating bats. In addition, the voltage-gated potassium (K+) channel gene KCNQ4 evolved similarly in all echolocating bats (Liu et al., 2011). Besides voltage motility, mammalian audition also requires hair bundle motility, which is executed by outer hair cells in the cochlea. The proteins encoded by the genes Cdh23 and Pcdh15 are essential for hair bundle motility (Di Palma et al., 2001). Homodimers of Cdh23 and Pcdh15 directly link to one another via their amino termini; they form the upper and lower portions of tip-links, respectively (shown in Figure 9), which are located between stereocilia within the hair bundle. The auditory system entails the perception and amplification of sound signals as well as the conversion of mechanical signals to ion fluxes in inner hair cells (Kazmierczak et al., 2007). A series of nerve channel openings are responsible for the management of electrical signals to the brain. Otoferlin may serve as the primary Ca2+ sensor that initiates membrane fusion at the ribbon synapse of the inner hair cell of the ear. Even though the aforementioned functions are involved in the conversion of sound signals to electrical impulses in the inner ear, otoferlin is also expressed in neurons and nerve fibers in the brain (Schug et al., 2006).
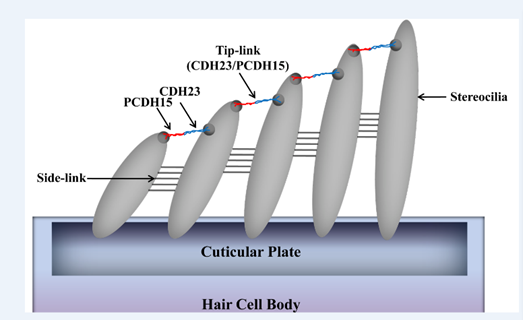
Fig. 9 Model of Cdh23 and Pcdh15 localization at tip-links (Kazmierczak et al., 2007).
The investigations of Ying Li reveal that the high-frequency acoustic sensitivities and selectivities of bat and whale echolocation are dependent on a shared molecular structure of prestin (Li et al., 2010). A functional examination of the parallel amino acid changes revealed here may provide insight into the structure-function link of prestin and the molecular basis of the acoustic adaptations in echolocation. It might also explain why the prestin of the bottlenose dolphin is so similar to those of the Rhinolophidae and Hipposideridae bat families (shown in Figure 10) (Li et al., 2010), even though the last common ancestor of bats and dolphins has not existed for at least 60 million years (Barber, 2013).
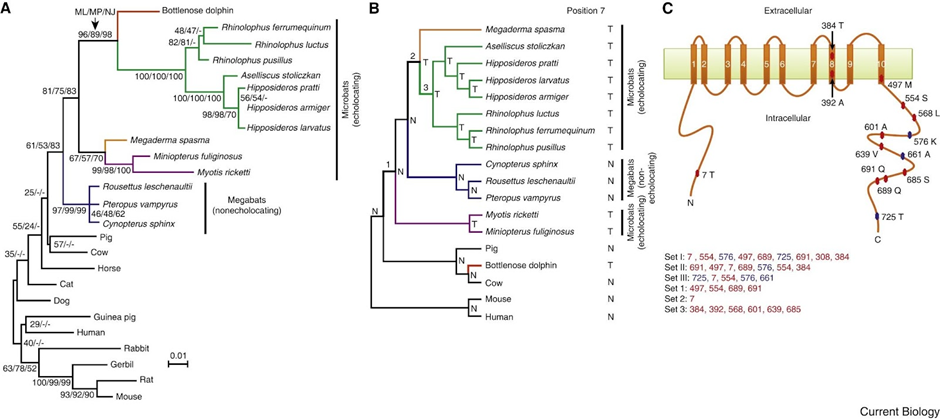
Fig. 10 Parallel evolution of prestins of echolocating bats and bottlenose dolphins (Li et al., 2010). (A) Maximum-likelihood (ML) tree reconstructed using the prestin protein sequences of 25 mammals, under the model of JTT-f with a gamma distribution of substitution rate variation among sites (shape parameter = 0.14). Numbers on interior branches are bootstrap percentages from ML, maximum-parsimony (MP), and neighbor-joining (NJ) analyses. The bootstrap value from an analysis is indicated as “-” when the branch does not exist in that analysis. (B) The species tree of 18 mammals. Tree branches are not drawn to scale. Parallel substitutions were examined between the red branch and the branches labeled 1, 2, and 3. The amino acid (N: Asn; T: Thr) at position 7 of prestin is shown for each interior and exterior node. (C) Locations of evolutionarily interesting amino acid sites in the structural model of prestin with ten transmembrane domains. Numbers associated with colored circles are the amino acid positions in the dolphin prestin sequence with the residues observed in dolphin indicated. Sets I, II, and III are the most important sites responsible for the misplacement of both dolphin and the two purple-labeled microbats in panel A, misplacement of dolphin, and misplacement of the two microbats, respectively, and are listed in the order of their support of the gene tree relative to the species tree (from high to low). Sets 1, 2, and 3 are sites that have experienced parallel amino acid substitutions between the dolphin branch (red in panel B) and branches 1, 2, and 3, respectively. Parallel evolution sites are shown in red, while the other sites are shown in blue.
To determine whether convergent changes in bat Prestin genes have also occurred in echolocating whales, the researchers sequenced the whole gene in a variety of echolocating toothed whales, non-echolocating baleen whales, and additional bats.
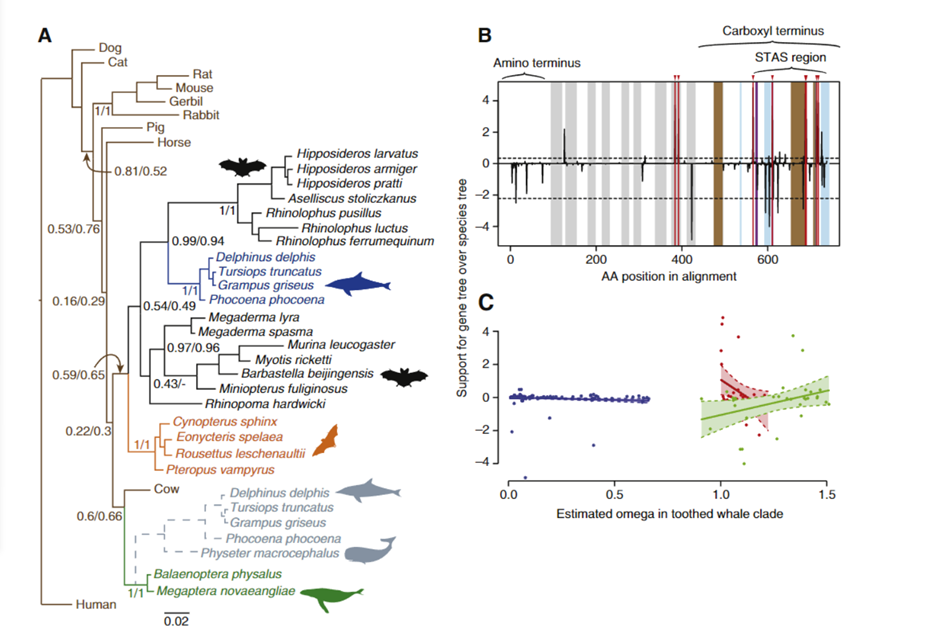
Fig. 11 Evidence of sequence convergence in the Prestin gene between dolphins and bats (Liu et al., 2009). (A) Bayesian phylogeny of Prestin based on amino acid data. Values at important nodes are posterior probabilities under two different models. Colors and drawings indicate the groups (baleen whales, toothed whales, fruit bats, and echolocating bats). The gray broken lines indicate the alternative position of toothed whales when the sperm whale (Physeter macrocephalus) was included in the analysis. (B) Relative support for gene tree and species tree topologies for different sites along the Prestin gene sequence. Values are the difference between site-wise negative log likelihood scores for the constrained tree minus the site-wise negative log likelihood scores for the convergent tree. Positive values indicate more support for the gene tree than the constrained tree, negative values indicate more support for the constrained tree. Red lines with arrows indicate the 8 sites showing statistically significant convergence based on simulations. Domains of the Prestin protein are indicated: in addition to those labeled on the figure, gray bands indicate transmembrane domains, blue bands coil domains, brown bands α-helices, and purple bands charge clusters. The first α-helix is the transmembrane. (C) Relationship between ω and support for the convergent topology (as in B above) for sites in three categories — blue points are sites under purifying selection across the tree, red those evolving neutrally across the tree, and green those with different omega values in the toothed whale clade than in other parts of the tree. Shaded regions are 95% confidence intervals for the regression lines.
The recognized species tree topology was strongly supported by trees based on nucleotide alignments from this larger dataset (shown in Figure 11), notwithstanding the previously observed clustering of echolocating bats. However, in amino acid sequence-based phylogenetic trees constructed using a number of phylogenetic techniques, echolocating dolphins currently form a well-supported clade with echolocating horseshoe and bottlenose dolphins (Liu et al., 2009).
In conclusion, these results indicate that the observed sequence convergence between dolphins and CF bats in prestin was due to the adaptive evolution of the dolphin gene. Currently, no substantial anatomical similarities have been made between these groups, with the possible exception of the precise tuning of the cochlear basal membrane in the porpoise (Phocoena). However, comprehensive studies of OHC length and fine-scale structure in cetaceans and bats are lacking and could provide insight given the high frequency hearing thresholds documented in dolphins and particular CF bats. Regardless, the observation of adaptive sequence convergence between two extremely distinct groups that share a complicated phenotype is unprecedented and suggests sequence convergence may be more frequent than previously thought.
Composite biomaterials in the cicada's main resonating device
The genus Cicada is made up of a variety of different species that have developed singing as a method for attracting nearby mates. While the exact mechanism differs in individual species, most cicadas utilize a system of tandem resonators to create a distinct and impressively loud song (Young & Bennet-Clark, 1995). The first resonator consists of a set of ribs and the tymbal plate; the tymbal plate deforms the ribs and as they return to their equilibrium position, a loud click is made (Young & Bennet-Clark, 1995). The tymbal plate's high frequency matches that of the resulting sound waves and acts as a resonator to amplify these clicks (Young & Bennet-Clark, 1995, Fahrunnida et al., 2022). The second resonating device acts as a Helmholtz resonator, a hollow and air-filled structure which amplifies incoming sound signals, which in the case of the cicada is composed of an air sac and the tympana (Young & Bennet-Clark, 1995). Only specific frequencies from the tymbal resonator are amplified, while surrounding frequencies are damped by standing waves within the air sac (Wolfe, 2005). For more information about Helmholtz resonance in cicadas, refer to the paper Resonating Devices in Nature for Communication and Information Reception found within this hyperbook. In general, this system enables cicadas to create songs of great vibrational magnitude (volume) while expanding relatively small amounts of energy. This is crucial from an evolutionary standpoint, as it allows male cicadas to attract a larger pool of potential mates. Not only does this enlarge the gene pool, but it also contributes to natural selection because cicadas who have louder and more efficient resonators are more apt to pass on their genes.
While the mechanical properties of these resonators are impressive, they only scrape the surface in terms of how they function. The chemical structures within the materials of the tymbal are the true microscopic controls for these resonating devices, as their chemical properties allow for resonance on a macroscopic scale. In this section, the chemical structures within the tymbal resonator will be analyzed, focusing on the characteristics of the composite materials which make up the tymbal plate and the surrounding ribs. To begin, the chemical nature of chitin and chitosan will be analyzed, as these are the primary components within the tymbal. Next, the resilin protein will be discussed as its rubber-like properties are crucial in high-frequency operations such as resonance. Finally, this section will conclude by explaining how these three materials form a highly flexible yet rigid composite biomaterial crucial to the tymbal resonator's functionality.
Chitin and chitosan: optimally tough biopolymers
Chitin is a natural polymer found in a variety of life forms. Most commonly, chitin is found within the cell walls of fungi, in the exoskeletons of crustaceans and most importantly for this section, in the exoskeletons of arthropods (Hahn et al., 2020, Sajomsang & Gonil, 2010). Chitin is a rigid and inelastic polysaccharide. Polysaccharides are carbohydrates made up of two or more sugar rings connected by a glycosidic bond, which in chitin's case, is two glucose rings. More specifically, the two glucose rings are 2-amino-D-glucose and N-acetylated-D-glucosamine (Hahn et al., 2020). This means that each has an additional amine and acetyl group as well as carbon-oxygen rings, as seen in Figure 12. These functional groups allow for hydrogen bonding not only within but also between chitin molecules (Hahn, 2020). Therefore, the chitin tends to clump tightly together to form three unique types: α-chitin, β-chitin and γ-chitin (Hahn et al., 2020, Sajomsang & Gonil, 2010). As seen in Figure 13, α-chitin molecules run in antiparallel directions. This exact structure allows for the most rigid form of chitin, which likely explains why it is the most stable and abundant form found in nature (Hahn et. al., 2020, Sajomsang & Gonil, 2010). Shown in Figure 13 are β-chitin molecules which run in parallel directions, creating weaker intermolecular forces and producing monoclinic crystals, which are not ideal for the formation of tough polymers (Hahn et al., 2020). Finally, γ-chitin has a combination of parallel and antiparallel chains. It is a comfortable middle ground between α-chitin and β-chitin (Hahn et al., 2020). However, α-chitin is often more useful because of its aforementioned toughness and rigidity.
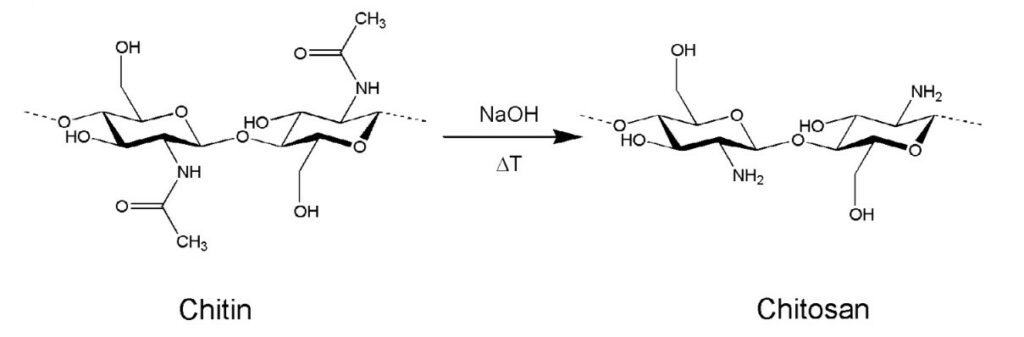
Fig. 12 On the left is chitin, a polysaccharide made up of glucose rings with added amine and acetyl groups. The right molecule is chitosan, the deactivated form of chitin (the acetyl group is removed) (Hanh et al., 2020).
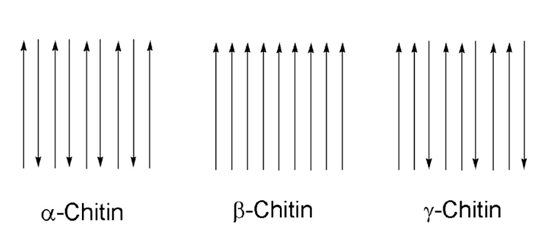
Fig 13 The different configurations of chitin, according to their chain directions. α-chitin has antiparallel chains while β-chitin has parallel chains. γ-chitin has a combination of both parallel and antiparallel chains (Hanh et al., 2020).
Another property of chitin is that it is largely insoluble in water-based solutions due to its large molecular weight and overall low polarity (Hahn et al., 2020). Chitosan is described as the deactivated form of chitin (Hahn et al., 2020). Through a simple deacetylation reaction of chitin, where the acetyl groups are removed, the amine groups are more freely expressed within the molecule, allowing chitosan to be more soluble due to decreased molecular weight and increased polarity of the molecule (Hahn et al., 2020). More specifically, chitosan can be dissolved in slightly acidic solutions such as those found within animals or fungi (Hahn et al., 2020). This makes chitosan a versatile molecule, as it still exhibits high polymerization while being available in water-solvent reactions. While chitosan is not particularly used for resonance in cicadas, it is pertinent to the discussion as it is gaining notoriety in the field of tissue and materials engineering, which uses biomaterials as inspiration.
Resilin: the organic rubber substitute
Resilin is an elastomeric protein commonly found in insects and crustaceans. For example, it exists in the ribs of cicadas and in the legs of fleas (Su et al., 2013). “Elastomeric” means that the protein has low stiffness yet high strain, or in other words, it has a low Young's modulus. Young's modulus is simply the ratio between the tensile strain that is exerted on a material (σ) and how much tensile stress it experiences as a result (ε). The expression for Young's modulus is shown in Formula 1 below:
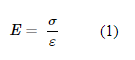
The relationship between stress and strain for resilin is exemplified in Figure 14. Materials that have a high Young's modulus are considered brittle, since under little strain they shear apart easily. Materials with low moduli are considered highly pliant as the molecules within rearrange in a way which decreases internal stress (Su et al., 2013, Thermodynamics, 2022). As a result, elastomeric materials have natural resilience, as they can survive millions of strong force interactions without being worn out by high tensile stresses (Su et al., 2013). For example, resilin can effortlessly return to its original shape even after several weeks of continuous strain (Su et al., 2013). It is important to note that the material characteristics of resilin are not the same as those of other materials. Plasticity refers to how malleable the resilin is, while elasticity is its ability to return to its original shape. Its resilience is a natural consequence of the previous two properties; it is a tough and durable material comparable to rubber. Furthermore, resilin is insoluble and heat resistant, allowing it to maintain its functionality despite the temperatures achieved during the cicada's song (Su et al., 2013).
Proteins are long chains of amino acids which have been folded in three dimensions and have a specific function based on their structure. Chitin, chitosan, and resilin are all proteins which are folded and kept in place by interactions between the backbone of the amino acids as well as their varying side groups. Researchers believe that the high content of polar side groups within resilin's chain of amino acids gives resilin elastic properties (Su et al., 2013). Furthermore, the high count of the amino acids proline and glycine contributes to the protein's elastomeric characteristics (Su et al., 2013). This is because proline has a large side chain; it is too bulky to form tertiary structures. Therefore, it can only conform to secondary structures like α-helixes or β-pleated sheets, such as the PPII conformation (a left-handed helix found in many elastomeric proteins) (Su et al., 2013). In contrast, glycine has a very small side group, allowing the amino acid backbone to be more flexible as there are fewer interactions with other portions of the polypeptide chain. The combination of these two amino acids has led to researchers theorizing that resilin acts like an entropic spring (Su et al., 2013).
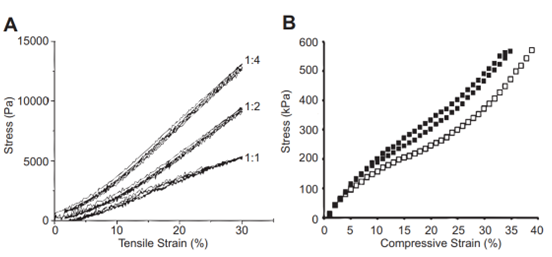
Fig. 14 Graphs of resilin modulus which serve as examples. Young's modulus is the amount of stress in a material as a function of either tensile or compressive strain. (A) The tensile modulus of a Resilin-based protein (RLP12) found within a tested hydrogel. The ratios represent the number of crosslinking sites to the crosslinker. (B) The compressive modulus of RZ10. Resilin-based proteins, much like natural resilin, have remarkably low tensile moduli (Su et al., 2013).
Entropic springs are common in modern life. For example, a simple rubber band can be modeled as an entropic spring. This can serve as an analogy for the resilin protein, as the same principles of thermodynamics and entropy act on the protein like they do on the rubber band. When the rubber band is at equilibrium, it is at a point where it is neither stretched nor compressed. As the band stretches or compresses, kinetic energy is stored within the band as potential and thermal energy. However, the net change in energy within the spring is negligible, since instead of stretching the molecular bonds within the band, they are simply rearranging themselves by rotating or bending around their equilibrium positions (Thermodynamics, 2022). Instead of increasing the enthalpy of the band, the entropy of the band is lowered. That is, the band goes from a higher state of probability to a lower state of probability (Su et al., 2013, Thermodynamics, 2022). In simple terms, entropy is a measure of molecular disorder, or how much molecular chaos is found within a system. As per the Second Law of Thermodynamics, processes in which the entropy in the universe increases are favoured and considered spontaneous. Given that the rubber band has lower entropy in the stretched or compressed state, a retractive or elastic force will spontaneously act on it to restore it to its equilibrium position (Su et al., 2013, Thermodynamics, 2022). As the change in entropy is the cause of the retractive force and not a change in internal energy, the rubber band is considered to be an entropic spring. The same principles apply to the resilin molecule, however, on a microscopic scale. This is advantageous for high-frequency environments such as the tymbal resonator. Entropic processes are efficient ways of achieving motion as the work done in non-spontaneous processes will naturally flow in the other direction without intervention or energy.
To conclude, resilin has several useful mechanical properties all due to its chemical nature. It is highly resilient, extensible and has a long lifetime, which makes it worthy of being described as an organic form of synthetic rubber.
Chitin and resilin: an ideal composite material for resonance
Now that the properties of chitin, chitosan and resilin have been discussed, we can piece together all three to see how these materials and their characteristics serve the cicada's resonating device. These materials form a composite which is used in the ribs that produce the audible clicks which, at high frequencies, create the cicada's song. The ribs are perpendicular to the tymbal's translation motion; as the tymbal moves backwards, it exerts a force on the ribs. However, these ribs are fixed in resilin at each end, and therefore, the individual rib deflects at its weakest point. As the tymbal retracts, the rib returns to its original position and a sound wave is produced (Young & Bennet-Clark, 1995).
The ribs themselves are composed of chitin, which explains how they manage to buckle hundreds of times per second without shattering (Fahrunnida et al., 2022). As mentioned before, chitin is an extremely rigid polymer which resists the internal and external forces applied by the tymbal plate onto the ribs (Hahn et al., 2020). The ribs are fixed at their ends to a resilin-chitin composite. Within this resilin, the chitin acts as a support structure, as it forms a polygonal mesh through protuberant bands, as seen in Figure 15 (Fahrunnida et al., 2022). These meshes are presumed to support the soft resilin as well as help it regain its shape faster after the ribs return to their original position (Fahrunnida et al., 2022). It is also important to note that thanks to the high thermal stability of both chitin and resilin, the composite material functions normally at the temperatures achieved by the resonator. After all, the resonator operates at a high frequency and while it is an efficient process, by sheer magnitude the resonator itself can reach up to 37 °C (Fahrunnida et al., 2022). A similar composite material is observed within the tymbal plate itself, however, the relationship seems to be the opposite. The tymbal is a membrane mostly made up of chitin, allowing it to be rigid, however, some resilin is present so it can remain flexible (Fahrunnida et al., 2022). This is useful, as the tymbal exerts significant forces onto the ribs, but it must remain flexible so that it can translate backwards in the first place.
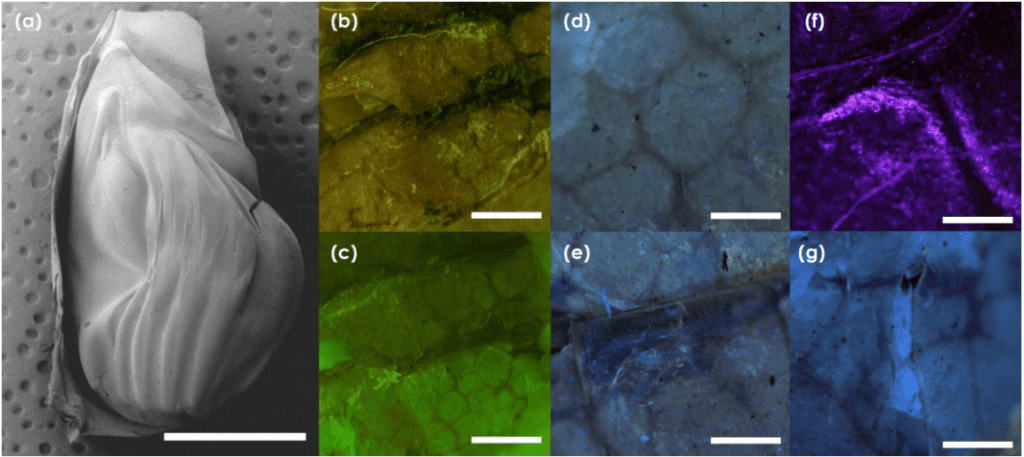
Fig. 15 a) The tymbal is pictured through a scanning electron microscope. b),c),d) Auto fluorescent images of chitin support structures and polygonal chitin structures. e) resilin autofluoresence around chitin ribs f) resilin autofluoresence around chitin joints g) large patches of resilin between chitin supports (Fahrunnida, 2022).
The chemical nature of the resilin-chitin composite structure is fascinating. Resilin proteins form a composite interface with chitin at the protein's chitin binding domain, which creates dityrosine bridges as the two materials polymerize (Fahrunnida et al., 2022). This creates a functional link between the molecules, as the chitin rich regions within the material delocalize the high stresses at the chitin-resilin interface, which decreases the chance of interfacial failure (Fahrunnida et al., 2022). Finally, when resilin interfaces with chitin, it tends to develop β-structures (secondary structures such as β-pleated sheets and β-turns), which in turn create a more ordered resilin structure with decreased compliance (Fahrunnida et al., 2022). While the resulting composite may have decreased malleability, it obtains higher rigidity while retaining the same benefits as resilin (such as high strain). This allows the resilin to push the ribs back into place during resonance, while resisting the high-frequency forces acted upon it, increasing efficiency, and lowering damage to the surrounding tissue and itself. There are other benefits in terms of resonance to this composite material. It has improved energy storage and fatigue resistance compared to natural resilin, which is crucial in high frequency operations (Fahrunnida, 2022).
Summary
The composite material made up of chitin and resilin has been engineered by natural selection and serves the function of resonance within a cicada. Chitin and its deactivated form chitosan are both rigid, tough polymers which provide structure and strength to a given system. They achieve this through their chemistry; chitin is a polysaccharide which links together tightly through antiparallel chains. Resilin is an elastomeric protein which resembles rubber. Even at high strain rates, the tensile stress felt by resilin is very low, making it an ideal elastic protein. Furthermore, it acts like an entropic spring by arranging its molecules in a way that the laws of thermodynamics ensure it returns to its equilibrium position. This is due to its chemical makeup, as its different amino acids contribute to specific characteristics that ensure elasticity. Putting these materials together results in an extremely versatile composite, in which the ratio between the materials dictates its flexibility versus strength. These serve as fantastic natural examples of biomaterial design considering these materials have a lot of promise in the fields of tissue engineering and drug delivery (Su et al., 2018).
Spider silk chemical modulation for specific mechanical results
Spider silk is a hierarchical biopolymer highly adaptable to a variety of environmental conditions (Sponner et al., 2007). The primary method of adaptation is chemical modulation of silk threads by the spider, which represents an example of extraordinary biomaterial engineering in nature; some species employ no fewer than eight distinct silk types in web-building, each of whose unique chemical properties induce variable mechanical results in the web (Su et al., 2018; Andersen, 1970). The necessity for this highly manipulable system is the spider's dependence on its web as its primary sensory mode (Landolfa & Barth, 1996; Naftilan, 1999). Spiders use resonances in the web to notify them of ensnared prey, conspecifics performing courtship rituals, and nearby avian predators, demonstrating the role of spider webs as resonating devices (Frohlich & Buskirk, 1982; Klärner & Barth, 1982; Stafstrom et al., 2020). Web-weaving spiders actively tension silk threads by altering their chemistry to tune the web for these biologically relevant signals. This section analyzes the biochemical, mechanical, molecular, and protein engineering principles used by spiders to generate these mechanical changes in their webs. Protein processing and crystalline structure of silk threads is first investigated, after which the anatomical mechanism governing protein processing is discussed.
Protein synthesis and processing in spider silk
Spider webs are an example of an extended phenotype – a morphological trait that exists outside and independently of the animal's body (Mortimer et at., 2018). Unlike the immutability of the spider's phenotype, spider silk chemistry can be instantaneously altered in the spider's many specialized silk glands, and webs can be rapidly constructed, deconstructed, and modified, granting spiders immense control and versatility over their principal sensory system (Heim, Römer, & Scheibel, 2010). The major constituents of silk are the spidroin family proteins, which are synthesized in specialized glands and are of high molecular weight, ranging from 200 to 350 kilodaltons (Sponner et al., 2007; Heim, Römer, & Scheibel, 2010). By manipulating the concentrations of these proteins in silk threads, and the order of the protein monomers during polymerization, spiders create different silk types with unique physical properties (Sponner et al., 2005; Liu, Shao, & Vollrath, 2005; Vollrath & Knight, 2001). This activity is known as protein processing and provides the biochemical basis for web tuning, enabling web-weaving spiders to amplify certain frequencies and attenuate others based on the resonance properties of the silk threads created. Depending on the environmental conditions, such as strong winds, rain, or limited food supply, spiders will produce threads with different mechanical properties to tune the web and optimize its functions (Masters, 1984; Mortimer et al., 2016; Watanabe, 2000). The process for this molecular and protein engineering is discussed below.
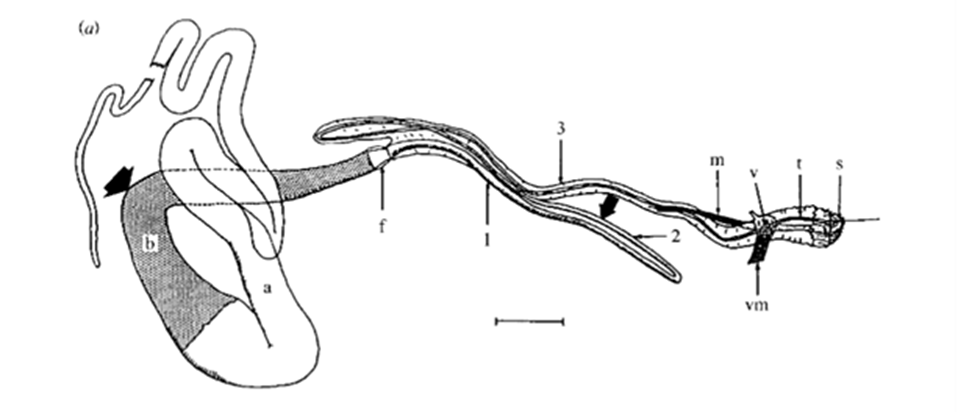
Fig. 16 Drawing of a dissected major ampullate gland. a and b, simply ‘zone A' and ‘zone B' (two arbitrary sections of the gland); f, funnel; 1, 2 and 3, first, second and third limbs of duct; m, duct levator muscle; v, valve; vm, valve tensor; t, terminal tubule; s, spigot. Scale bar: 1 mm (Knight & Vollrath, 1999).
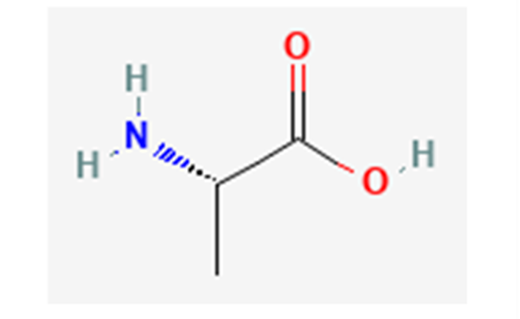
Fig. 17 Alanine in its non-zwitterionic form; in forming alanine chains, the hydroxyl group of one alanine and a hydrogen from an amino group on another detach to become water, enabling the adjacent alanine residues to bond, as shown in Figure 18 below (NCBI, 2022a).
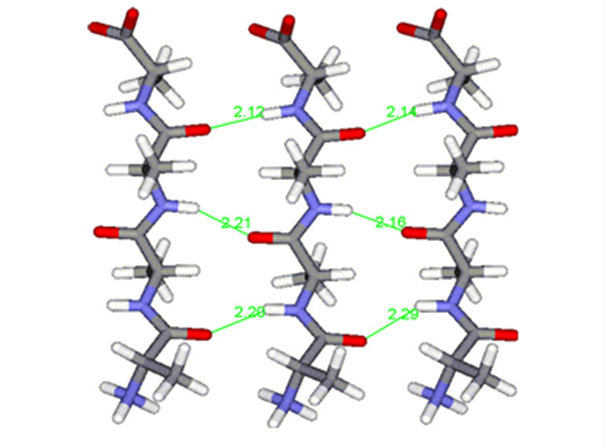
Fig. 18 Polyalanine chains form hydrogen bonds (shown in green) with each other, creating highly stable β-sheets, strengthening molecular cohesion in spider silk (Asakura et al., 2016).
Spiders have several specialized silk glands that produce silk fibers with unique chemical and physical properties (Sponner et al., 2007; Heim, Römer, & Scheibel, 2010). The main framework silk is often called the dragline silk and is produced in a spider's major ampullate (MA) gland (see Figure 16 below), serving as the backbone for web construction (Heim, Römer, & Scheibel, 2010). Dragline silk protein constituents are rich in alanine residues (Sponner et al., 2007; Thiel & Viney, 2003). Polyalanine-based peptides (those with long, repeated sections of alanine) tend to form β-sheets due to hydrogen bonds between the amino and carbonyl groups on different alanine residues (see Figures 17 and 18 below) (Asakura et al., 2016). This results in strong molecular cohesion in dragline silk, conferring fiber strength with high Young's modulus (Sponner et al., 2007). In fact, these β-sheets often form crystalline domains in the silk due to the strength and consistency of the hydrogen bonds (Vollrath & Knight, 2001; Thiel & Viney, 2003).
Dragline silk also tends to have a significant proline concentration; while percent alanine is usually in the 20 to 30% range for most dragline spider silks, proline percentage generally extends from 5 to 10% (still a sizable minority). Contrary to alanine, proline disfavours crystallization in dragline silk by preventing the formation of ordered secondary protein structures, like α-helices and β-sheets, due to the nonpolar section of its ring (Liu et al., 2008) (see Figure 19). Hence, proline-rich silk tends to have more elastic mechanical properties due to the higher structural disorder (Liu et al., 2008). Through the presence of polyalanine chains in peptides, silk increases rigidity, while inserting individual disruptive proline residues among the sequence, it increases tensile strength and ductility. This combination of stiffness and elasticity makes dragline silk incredibly resilient, yet structurally stable – a perfect combination for an architectural backbone.
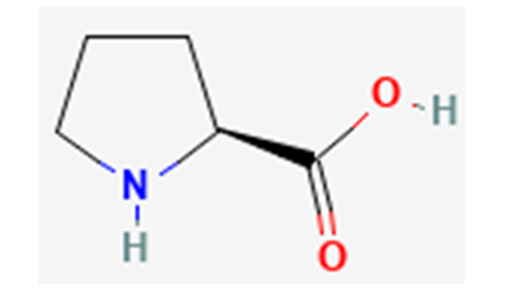
Fig. 19 Proline in its non-zwitterionic form; proline's non polar elements (especially from its ring) disrupt hydrogen bonds between nearby polar amino acid residues, preventing the formation of secondary structures in proteins (NCBI, 2022b).
Spiders can control the chemical makeup of their webs – such as by changing the prevalence of alanine-rich peptides in dragline silk, or the proline concentration in its constituents – providing a means to modulate Young's modulus and tensile strength based on the extent of molecular cohesion (Vollrath & Knight, 2001; Work & Young, 1987). Thus, web-weaving spiders can biochemically engineer the resonance properties of dragline silk by manipulating the thread's peptide sequences.
The three other major silk types observed in most web-weaver silks are minor ampullate (MI) silk, flagelliform silk, and piriform silk (produced in the MI, flagelliform, and piriform glands respectively). Spiders have been observed to eat provisional (MI) threads to recycle materials in the process of depositing flagelliform silk. Figure 20 below illustrates their roles in web architecture. These silks play a secondary role for spider webs as resonating devices but are still integral to the structural design of the web. MI silk is a temporary scaffolding silk that serves to stabilize the emerging structure and provide a template for the later addition of flagelliform silk (Heim, Römer, & Scheibel, 2010). It has a similar amino acid composition to dragline silk, except that it lacks a substantial proline concentration (Work & Young, 1987; Andersen, 1970). This lends to a more rigid structure with greater crystallization, but also greater brittleness. While the evolutionary significance of a lack of proline in MI silk is not completely understood (Andersen, 1970), from an architectural engineering perspective, it seems sensible to have a non-permanent scaffold be more rigid to aid in structural stability during the web-weaving process. More ductile threads would be prone to deformation, while stiff threads would enable precise, expeditious construction. The trade-off of brittleness is of minimal consequence because MI silk is promptly dismantled and replaced by flagelliform silk once structural stability is achievable without it (Heim, Römer, & Scheibel, 2010). The chemical intricacies of the other two silk types are fascinating, but not as relevant to the discussion of resonance in spider webs.
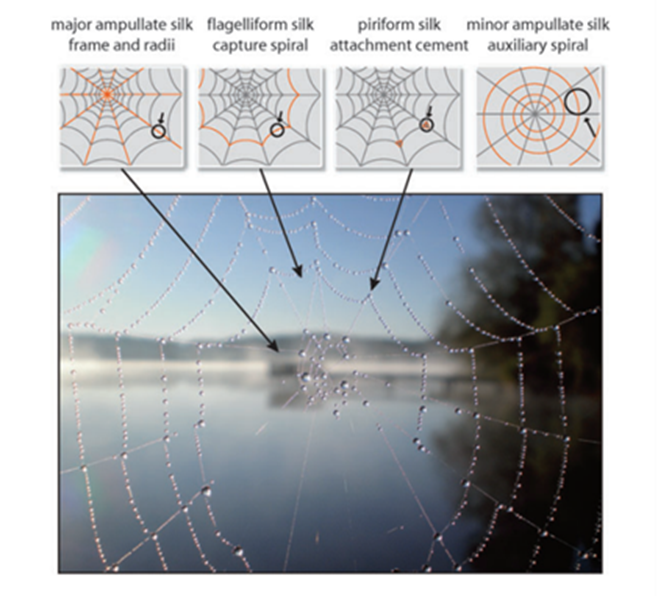
Fig. 20 Major silk types used in the construction of spider webs (Heim, Römer, & Scheibel, 2010).
Anatomical mechanism of protein processing
The molecular arrangement necessary for the formation of β-sheets in dragline silk is now well understood, but the mechanism for designing proteins to achieve this arrangement has not yet been elucidated. Before exiting the spider's body, the pre-processed liquid protein solution, often called spinning dope, is composed of established, folded spidroin proteins. Through evolution, spiders have developed a biomaterial engineering strategy to partially denature these intact proteins, then stimulate their renaturation outside of their bodies in a new form that creates strong, resilient threads through the fibrillogenesis of β-sheet-rich amyloids (Knight, Knight, & Vollrath, 2000; Rammensee et al., 2008). The necessity for this complex procedure arises because spider silk must be malleable during web construction, but stable and resistant afterwards. A human parallel would be cement; if cement were unyielding before being laid, it would not be an effective building material.
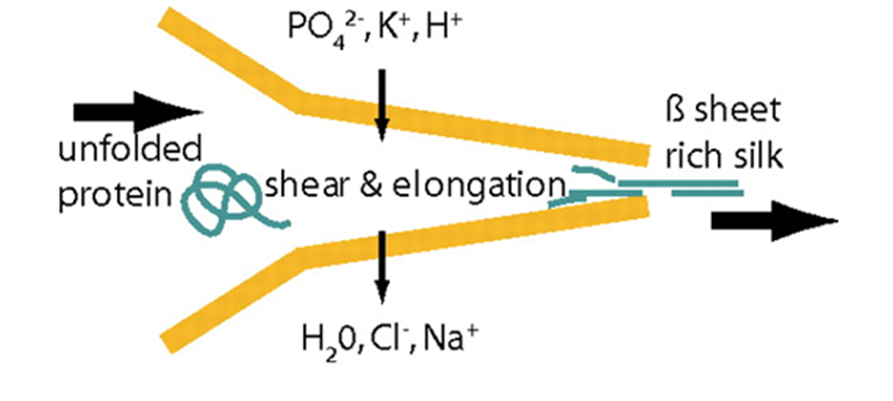
Fig. 21 Schematics of the proposed natural spinning process of spider dragline silk. A protein solution is pulled through the spinning duct, the pH is decreased (from slightly basic to slightly acidic). Further, lone protons, and phosphate and potassium ions are added to the spinning dope while water, sodium, and chloride ions are extracted. The resulting silk fiber is rich in β-sheets, increasing its crystallization (and thus Young's modulus) (Rammensee et al., 2008).
In order for hydrogen bonds to form between polyalanine stretches in spidroins of the dragline silk, spiders must ensure that spinning dope protein constituents are partially uncoiled, or denatured, before exiting the spider, then recoiled in a different configuration after extrusion (Slotta et al., 2008; Knight & Vollrath, 2001). This requires rigid control over strain rate, pH, and ion concentrations in the duct (refer back to Figure 16) that connects a spider silk gland to its spigots (terminal projections through which single silk threads are extruded) (Rammensee et al., 2008; Knight, Knight, & Vollrath, 2000; Mariano-Martins, Hung, & Torres, 2020). Figure 21 illustrates the role of strain, pH, and ion concentration in the process of converting spinning dope into β-sheet-rich dragline silk.
All three effects on the dope play a role in its denaturation and renaturation. Spinning dope undergoes substantial strain in the duct which physically uncoils its constituent proteins by overcoming the intermolecular forces (greatly hydrogen bonds) maintaining secondary and tertiary structures (Knight, Knight, & Vollrath, 2000; Thiel & Viney, 2003). The walls of the duct are hypothesized to provide the necessary stress through a shear force, demonstrating an example of the mechanical engineering of proteins by spiders (Knight, Knight, & Vollrath, 2000).
Changes to pH and ion concentrations aid in moulding the proteins before extrusion. Secretory cells surrounding the duct adjust pH and ionic conditions through proton and ion channels (Rammensee et al., 2008; Knight & Vollrath, 2001). PH within the duct changes from slightly basic to slightly acidic as the dope approaches the spigot (Rammensee et al., 2008). In other words, greater concentrations of H+ are secreted into the duct, resulting in the protein accumulating a net charge, far from its isoelectric point (the condition allowing a protein to reach a neutral charge) (Yang & Honig, 1993). Changing ion concentrations has a similar but more localized effect given the removal or addition of specific ions (rather than a simple proton, as is the case in pH change). The progressing trend of unbalanced charge accumulation causes protein denaturation by disturbing the highly particular interactions that lead to correct protein folding (Talley & Alexov, 2010; Yang & Honig, 1993; Skoulakis & Goodfellow, 2003). The thermodynamic principle behind this result is based on the Linderstrom-Lang model, which states that a protein's electrostatic free energy is proportional to the square of the net surface charge. Formula 2 below illustrates this relationship:
EF ∝ qs2 (2)
where EF is electrostatic free energy and qs is the net surface charge.
Free energy is the energy in a system available to perform thermodynamic work; in other words, higher free energy allows a system to spontaneously exert greater forces on itself or its surroundings (Yang & Honig, 1993). Hence, these spontaneous forces, if the net charge is sufficient, will be greater than the intermolecular forces maintaining secondary and tertiary structures in the spinning dope, enabling protein denaturation prior to extrusion (Yang & Honig, 1993). Hence, pH and ionic conditions contribute to the partial uncoiling of spinning dope spidroins by increasing the free energy in the material beyond functional levels for the proteins.
Post-extrusion renaturation results in a new protein aggregation, forming what is known as amyloid fibers (Yang & Honig, 1993; Skoulakis & Goodfellow, 2003). Amyloids have a core β-sheet structure and are often the result of partial denaturation followed by irreversible aggregation of proteins (Skoulakis & Goodfellow, 2003). The mechanism of amyloid fibrillogenesis is still not fully understood (Skoulakis & Goodfellow, 2003; Kelly, 1998), though some proteins are more susceptible than others, suggesting that spidroins are designed to some extent to enable this renaturation process, demonstrating another instance of protein engineering by the spider. This anatomical mechanism, from partial uncoiling to protein aggregation, produces sturdy, resilient fibers due to an optimized balance of crystalline order and proline-induced disorder. The chemical modulation of the level of order by the spider allows it to tune its web to function as a resonating device; depending on the mechanical characteristics induced by the chemical properties of the threads, the spider can selectively amplify and dampen specific vibrations, contributing to the spider's overall success in hunting, mating, and predator evasion.
Summary
This section has examined the chemical controls that allow web-weaving spiders to modulate the mechanical properties of their webs. Protein processing and its anatomical mechanism were explored to elucidate the engineering principles used by Araneae species while chemically constructing their webs. The incredible mutability of spider silk makes it a versatile tool, allowing the spider to rapidly adapt to changes in its environment. By manipulating structural order in silk on the molecular level, spiders produce a variety of distinct silk types, and each type plays a specific role in the web's function as a resonating device. Differences in alanine and proline content control resilience and elasticity tendencies in the fibers, which in turn affect the web's resonance properties. This biochemical engineering strategy grants spiders precision in choosing which environmental signals they would like to amplify or ignore (through resonance). This section has demonstrated the outstanding capacity of spiders to chemically manipulate their extended phenotype to harness resonance for sensory perception, a strategy that has enabled Araneae success for eons.
Conclusion
The biochemical perspective on these resonating devices has shed light on an often overlooked portion of this physics-dominated field of research. It is important to remember that physical characteristics apparent on the macroscale are often only expressed, and therefore only possible, via the chemical properties of materials in a system. This paper investigated multiple examples of resonating devices at cellular and molecular scales, giving particular attention to their materials, transport phenomena, chemical interactions, and thermodynamic properties. First, the process of mechanotransduction in the mammalian organ of Corti was explored. This process was expanded to the specific examples of bats and dolphins, and their morphological adaptations for echolocation were explained through genetics and evolution. Then, the chitin-resilin biocomposite in cicada tymbal resonators was examined, demonstrating how male cicadas optimize rigidity and resilience in this structure to maximize their resonance capacity for mating calls. Finally, the chemical modulation of silk by web-weaving spiders was analyzed, discussing the specific controls used during silk synthesis to produce specific mechanical results in the spider's web.
Throughout these diverse examples, common themes can be identified, the most salient being that of polymers, particularly proteins, and how they are structured within biological systems. Whether as a material, as in the case of spidroins and resilin, or inside an intricate piece of biological machinery, as in the case of prestin, proteins have exceptional versatility and complexity, taking center stage in biochemical processes. Once again, the conclusion that evolution is an incredibly competent designer is particularly prominent, as genetic factors play a significant role in configuring biochemicals within biological systems. Another recurring theme is the depth of what is unknown, particularly noticeable in the sections on mechanotransduction and spider silk. Bioengineering at the cellular and molecular level is still a nascent field, and much remains to be learned. This review has exemplified what can be achieved in this area of study with regard to resonating devices, as well as the untapped potential of biomimetic methods for solving human issues.
References
Ahmed, Z.M., et al. (2003). The Molecular Genetics of Usher Syndrome. Clinical Genetics, 63(6), 431–444. 10.1034/j.1399-0004.2003.00109.x.
Andersen, S. O. (1970). Amino acid composition of spider silks. Comparative Biochemistry and Physiology, 35(3), 705-711. https://doi.org/https://doi.org/10.1016/0010-406X(70)90988-6
Asakura, T., Horiguchi, K., Aoki, A., Tasei, Y., & Naito, A. (2016). Parallel β-Sheet Structure of Alanine Tetrapeptide in the Solid State As Studied by Solid-State NMR Spectroscopy. The Journal of Physical Chemistry B, 120(34), 8932-8941. https://doi.org/10.1021/acs.jpcb.6b06292
Barber, Elizabeth (2013). Are dolphins basically wet bats? Genetic study reveals surprising similarities. The Christian Science Monitor. https://www.csmonitor.com/Science/2013/0905/Are-dolphins-basically-wet-bats-Genetic-study-reveals-surprising-similarities
Bear, M. F., Connors, B. W., & Paradiso, M. A. (2015). Neuroscience : exploring the brain (4th ed.). Lippincott Williams & Wilkins.
Beurg, M., & Fettiplace, R. (2017). Piezo2 as the anomalous mechanotransducer channel in auditory hair cells: mechanosensitive channels in cochlear hair cells. The Journal of Physiology, 595(23), 7039–7048. https://doi.org/10.1113/JP274996
Brown, C. (2013). Hair Cells [Lecture Recording]. MIT OpenCourseWare. https://ocw.mit.edu/courses/9-04-sensory-systems-fall-2013/resources/lec-15-hair-cells/
Caprara, G. A., Mecca, A. A., & Peng, A. W. (2020). Decades-old model of slow adaptation in sensory hair cells is not supported in mammals. Science Advances, 6(33), 4922. https://doi.org/10.1126/sciadv.abb4922
Dallos, P. (2008). Cochlear Amplification, Outer Hair Cells and Prestin. Current Opinion in Neurobiology. pubmed.ncbi.nlm.nih.gov/18809494/.
Di Palma, F., et al. (2001). Genomic Structure, Alternative Splice Forms and Normal and Mutant Alleles of Cadherin 23 (Cdh23). Gene, 281(1-2), 31–41. 10.1016/s0378-1119(01)00761-2.
Fahrunnida, P. R., Robert, C., Retnoaji, B., & Alam, P. (2022). Morphological and Viscoelastic Properties of the Cicada Tymbal. Macromol, 2, 315-323. doi.org/10.3390/macromol2030020
Fenton, B., et al. (2014). Sonar Signals of Bats and Toothed Whales. Biosonar, 11–59. 10.1007/978-1-4614-9146-0_2.
Fettiplace, R. (2017). Hair cell transduction, tuning and synaptic transmission in the mammalian cochlea. Comprehensive Physiology, 7(4), 1197–1227. https://doi.org/10.1002/cphy.c160049
Fettiplace, R. (2006). Active Hair Bundle Movements in Auditory Hair Cells. The Journal of Physiology, 576(1), 29–36. 10.1113/jphysiol.2006.115949.
Frohlich, C., & Buskirk, R. E. (1982). Transmission and attenuation of vibration in orb spider webs. Journal of Theoretical Biology, 95(1), 13-36. https://doi.org/https://doi.org/10.1016/0022-5193(82)90284-3
Ge, J., Elferich, J., Dehghani-Ghahnaviyeh, S., Zhao, Z., Meadows, M., von Gersdorff, H., Tajkhorshid, E., & Gouaux, E. (2021). Molecular mechanism of prestin electromotive signal amplification. Cell, 184(18), 4669–4679. https://doi.org/10.1016/j.cell.2021.07.034
Hahn, T., Tafi, E., Paul, A., Salvia, R., Falabella, P. & Zibek, S. (2020). Current state of chitin purification and chitosan production from insects. Journal of Chemical Technology and Biotechnology, 95(11). 2775-2795. doi.org/10.1002/jctb.6533
Heim, M., Römer, L., & Scheibel, T. (2010). Hierarchical structures made of proteins. The complex architecture of spider webs and their constituent silk proteins [10.1039/B813273A]. Chemical Society Reviews, 39(1), 156-164. https://doi.org/10.1039/B813273A
Homma, K., & Dallos, P. (2011). Evidence that prestin has at least two voltage-dependent steps. The Journal of Biological Chemistry, 286(3), 2297–307. https://doi.org/10.1074/jbc.M110.185694
Houseman, M. J. (2001). A Novel Mutation in a Family with Non-Syndromic Sensorineural Hearing Loss That Disrupts the Newly Characterised OTOF Long Isoforms. Journal of Medical Genetics, 38(8). 10.1136/jmg.38.8.e25
Kazmierczak, P., et al. (2007). Cadherin 23 and Protocadherin 15 Interact to Form Tip-Link Filaments in Sensory Hair Cells. Nature, 449(7158), 87–91. www.ncbi.nlm.nih.gov/pubmed/17805295, 10.1038/nature06091.
Kelly, J. W. (1998). The alternative conformations of amyloidogenic proteins and their multi-step assembly pathways. Current Opinion in Structural Biology, 8(1), 101-106. https://doi.org/https://doi.org/10.1016/S0959-440X(98)80016-X
Klärner, D., & Barth, F. G. (1982). Vibratory signals and prey capture in orb-weaving spiders (Zygiella x-notata, Nephila clavipes; Araneidae). Journal of comparative physiology, 148(4), 445-455. https://doi.org/10.1007/BF00619783
Knight, D. P., & Vollrath, F. (1999). Liquid crystals and flow elongation in a spider's silk production line. Proceedings of the Royal Society of London. Series B: Biological Sciences, 266(1418), 519-523. https://doi.org/doi:10.1098/rspb.1999.0667
Knight, D. P., & Vollrath, F. (2001). Changes in element composition along the spinning duct in a Nephila spider. Die Naturwissenschaften, 88(4), 179–182. https://doi.org/10.1007/s001140100220
Knight, D. P., Knight, M. M., & Vollrath, F. (2000). Beta transition and stress-induced phase separation in the spinning of spider dragline silk. International Journal of Biological Macromolecules, 27(3), 205-210. https://doi.org/https://doi.org/10.1016/S0141-8130(00)00124-0
Landolfa, M. A.; Barth, F. G. (1996). Vibrations in the orb web of the spider Nephila clavipes: cues for discrimination and orientation. Journal of Comparative Physiology A, 179 (4), 493-508. DOI: 10.1007/BF00192316.
Li, Y., et al. (2010). The Hearing Gene Prestin Unites Echolocating Bats and Whales. Current Biology, 20(2), R55–R56. 10.1016/j.cub.2009.11.042.
Liu, Y., et al. (2009). Convergent Sequence Evolution between Echolocating Bats and Dolphins. Current Biology, 20(2), R53–R54. 10.1016/j.cub.2009.11.058.
Liu, Y., et al. (2011). The Voltage-Gated Potassium Channel Subfamily KQT Member 4 (KCNQ4) Displays Parallel Evolution in Echolocating Bats. Molecular Biology and Evolution, 29(5), 1441–1450. 10.1093/molbev/msr310.
Liu, Y., Shao, Z., & Vollrath, F. (2005). Relationships between supercontraction and mechanical properties of spider silk. Nature Materials, 4(12), 901-905. https://doi.org/10.1038/nmat1534
Liu, Y., Sponner, A., Porter, D., & Vollrath, F. (2008). Proline and Processing of Spider Silks. Biomacromolecules, 9(1), 116-121. https://doi.org/10.1021/bm700877g
Mariano-Martins, P., Lo-Man-Hung, N., & Torres, T. T. (2020). Evolution of Spiders and Silk Spinning: Mini Review of the Morphology, Evolution, and Development of Spiders' Spinnerets [Mini Review]. Frontiers in Ecology and Evolution, 8. https://doi.org/10.3389/fevo.2020.00109
Masters, W. M. (1984). Vibrations in the Orbwebs of Nuctenea sclopetaria (Araneidae): I. Transmission through the Web. Behavioral Ecology and Sociobiology, 15(3), 207–215. http://www.jstor.org/stable/4599721
McPherson, D. R. (2018). Sensory hair cells: an introduction to structure and physiology. Integrative and Comparative Biology, 58(2), 282–300. https://doi.org/10.1093/icb/icy064
Mortimer, B., Soler, A., Siviour, C. R., & Vollrath, F. (2018). Remote monitoring of vibrational information in spider webs. Die Naturwissenschaften, 105(5-6), 37. https://doi.org/10.1007/s00114-018-1561-1
Mortimer, B., Soler, A., Siviour, C. R., Zaera, R., & Vollrath, F. (2016). Tuning the instrument: sonic properties in the spider's web. Journal of the Royal Society, Interface, 13(122), 20160341. https://doi.org/10.1098/rsif.2016.0341
Naftilan, S. A. (1999). Transmission of vibrations in funnel and sheet spider webs. International Journal of Biological Macromolecules, 24(2), 289-293. https://doi.org/https://doi.org/10.1016/S0141-8130(98)00092-0
National Center for Biotechnology Information (2022). PubChem Compound Summary for CID 145742, Proline. Retrieved October 26, 2022 from https://pubchem.ncbi.nlm.nih.gov/compound/Proline.
National Center for Biotechnology Information (2022). PubChem Compound Summary for CID 5950, Alanine. Retrieved October 26, 2022 from https://pubchem.ncbi.nlm.nih.gov/compound/Alanine.
Navaratnam, D., et al. (2005). N-Terminal-Mediated Homomultimerization of Prestin, the Outer Hair Cell Motor Protein. Biophysical Journal, 89(5), 3345–3352. 10.1529/biophysj.105.068759.
Peng, A. W., Effertz, T., & Ricci, A. J. (2013). Adaptation of mammalian auditory hair cell mechanotransduction is independent of calcium entry. Neuron, 80(4), 960–72. https://doi.org/10.1016/j.neuron.2013.08.025
Rammensee, S., Slotta, U., Scheibel, T., & Bausch, A. R. (2008). Assembly mechanism of recombinant spider silk proteins. Proceedings of the National Academy of Sciences, 105(18), 6590-6595. https://doi.org/doi:10.1073/pnas.0709246105
Sajomsang, W. & Gonil, P. (2010). Preparation and characterization of α-chitin from cicada sloughs. Materials Science and Engineering: C, 30(6), 357-363. doi.org/10.1016/j.msec.2009.11.014
Schug, N., et al. (2006). Differential Expression of Otoferlin in Brain, Vestibular System, Immature and Mature Cochlea of the Rat. European Journal of Neuroscience, 24(12), 3372–3380. 10.1111/j.1460-9568.2006.05225.x
Shen, Y.Y., et al. (2012). Parallel Evolution of Auditory Genes for Echolocation in Bats and Toothed Whales. PLoS Genetics, 8(6). www.ncbi.nlm.nih.gov/pmc/articles/PMC3386236/, 10.1371/journal.pgen.1002788.
Skoulakis, S., & Goodfellow, J. M. (2003). The pH-dependent stability of wild-type and mutant transthyretin oligomers. Biophysical journal, 84(5), 2795–2804. https://doi.org/10.1016/S0006-3495(03)70009-5
Slotta, U. K., Rammensee, S., Gorb, S., & Scheibel, T. (2008). An Engineered Spider Silk Protein Forms Microspheres. Angewandte Chemie International Edition, 47(24), 4592-4594. https://doi.org/https://doi.org/10.1002/anie.200800683
Sponner, A., Unger, E., Grosse, F., & Weisshart, K. (2005). Differential polymerization of the two main protein components of dragline silk during fibre spinning. Nature Materials, 4(10), 772-775. https://doi.org/10.1038/nmat1493
Sponner, A., Vater, W., Monajembashi, S., Unger, E., Grosse, F., & Weisshart, K. (2007). Composition and hierarchical organisation of a spider silk. PloS one, 2(10), e998. https://doi.org/10.1371/journal.pone.0000998
Stafstrom, J. A., Menda, G., Nitzany, E. I., Hebets, E. A., & Hoy, R. R. (2020). Ogre-Faced, Net-Casting Spiders Use Auditory Cues to Detect Airborne Prey. Current biology : CB, 30(24), 5033–5039.e3. https://doi.org/10.1016/j.cub.2020.09.048
Su, I., Qin, Z., Saraceno, T., Krell, A., Mühlethaler, R., Bisshop, A., & Buehler, M. J. (2018). Imaging and analysis of a three-dimensional spider web architecture. Journal of The Royal Society Interface, 15(146), 20180193. https://doi.org/doi:10.1098/rsif.2018.0193
Su, R. S.-C., Gill, E. E., Kim, Y. & Liu, J. C. (2018). Characterization of Resilin-like Proteins with Tunable Mechanical Properties. Journal of the Mechanical Behaviour of Biomedical Materials, 91, 68-75. doi.org/10.1016/j.jmbbm.2018.11.015
Su, R. S.-C., Kim, Y. & Liu, J. C. (2013). Resilin: Protein-based elastomeric biomaterials. Acta Biomaterialia. 10(4), 1742-1611. doi.org/10.1016/j.actbio.2013.06.038
Talley, K., & Alexov, E. (2010). On the pH-optimum of activity and stability of proteins. Proteins, 78(12), 2699–2706. https://doi.org/10.1002/prot.22786
Thermodynamics: the entropy spring. (2022). University of Cambridge – Dissemination of IT for the Promotion of Material Sciences. www.doitpoms.ac.uk/tlplib/stiffness-of-rubber/thermodynamics.php
Thiel, B. L., & Viney, C. (2003). Spider major ampullate silk (drag line): smart composite processing based on imperfect crystals. Journal of Microscopy, 185(2), 179-187. https://doi.org/10.1046/j.1365-2818.1997.d01-607.x
Vollrath, F., & Knight, D. P. (2001). Liquid crystalline spinning of spider silk. Nature, 410(6828), 541-548. https://doi.org/10.1038/35069000
Watanabe T. (2000). Web tuning of an orb-web spider, Octonoba sybotides, regulates prey-catching behaviour. Proceedings. Biological sciences, 267(1443), 565–569. https://doi.org/10.1098/rspb.2000.1038
Wolfe, J. (2005). Helmholtz Resonance. The University of New South Wales – Music Acoustics. newt.phys.unsw.edu.au/jw/Helmholtz.ht
Work, R. W., & Young, C. T. (1987). The Amino Acid Compositions of Major and Minor Ampullate Silks of Certain Orb-Web-Building Spiders (Araneae, Araneidae). The Journal of Arachnology, 15(1), 65–80. http://www.jstor.org/stable/3705510
Xu, Z. (2018). Mechanotransduction of the hair cell (Ser. Springerbriefs in biochemistry and molecular biology). Springer. https://doi.org/10.1007/978-981-10-8557-4
Yang, A.-S., & Honig, B. (1993). On the pH Dependence of Protein Stability. Journal of Molecular Biology, 231(2), 459-474. https://doi.org/https://doi.org/10.1006/jmbi.1993.1294
Young, D. & Bennet-Clark, H. C. (1995). The role of the timbal in cicada sound production. Journal of Experimental Biology, 198(4), 1001-1019. doi.org/10.1242/jeb.198.4.1001 https://studycrumb.com/alphabetizer