The Metabolic and Regulatory Roles of Adipose Tissue in Organisms
Edward Tong, Josh Negenman, Michelle Sayeh, Milana Farah
Abstract
Fats are one of the most versatile molecules in mammals, fulfilling countless metabolic and non-metabolic roles. Metabolism of fats serves many purposes. Through lipolysis, fat molecules are broken down into glycerol and three fatty acid chains, which in turn are used by cells to produce energy. Through the reverse process, lipogenesis, extra energy (i.e., glucose) is reacted to form triacylglycerols and stored within an organism's fat deposits. The metabolism of fats into energy also releases water, referred to as metabolic water, which is crucial for many animals' survival in dry environments. Fats also play non-metabolic roles. Adipose tissue is an active endocrine organ, secreting hormones and chemicals to help regulate bodily systems, including blood glucose homeostasis in animals. Fat also plays a key role in the function of bone marrow and hematopoiesis, regulating blood flow within the marrow. This paper reviews some of the chemical functions of adipose tissue in the bodies of animals. Firstly, the metabolic functions of fats are discussed, including the formation and hydrolysis of triacylglycerol, metabolic water, and thermogenesis. Secondly, the regulatory functions of adipose tissues are addressed, providing an overview of adipose tissue's function as an endocrine organ, and the role of adipocytes in bone marrow.
Introduction
Adipose tissue is composed of adipocyte fat cells found in all mammals. It can be categorized into two distinct types: white adipose tissue (WAT) and brown adipose tissue (BAT), both types having distinct properties and functions (Hernández, n.d.).
Triacylglycerol (TAG) makes up most of the volume of adipocytes and is the primary constituent of energy storage in eukaryotic cells. This lipid category is essential for the development of the water barrier of the skin and acts as a mechanical cushion within joints and around internal organs. It also plays a vital role in the body's insulation (Coleman & Mashek, 2011). Each TAG molecule is composed of three fatty acids and one glycerol molecule. These lipid molecules are stored in adipocytes in the form of a single, large lipid droplet that takes up 90% of the cytoplasm and pushes the nucleus, the cytosol layer, and the mitochondria towards the cell's periphery (Figure 1). However, the amount of TAG is inconstant, and is in fact in a constant TAG – fatty acid cycle depending on the balance between lipogenesis and lipolysis (Bolsoni-Lopes & Alonso-Vale, 2015).
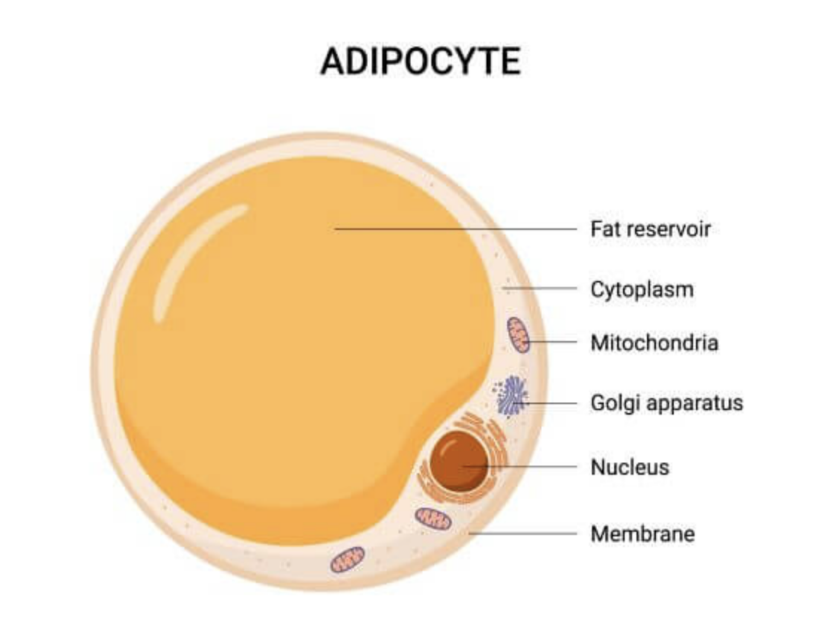
Fig. 1 White adipocyte composition (Latham, 2021).
The bodies of animals are incredibly complex, and each system and organ requires many molecules, hormones, and contributions from other systems in order to properly function. These bodily systems are all interconnected and have evolved incredibly complex mechanisms for the production and regulation of necessary components for the functioning of each system. Adipose tissue contributes to the proper function of many such systems, both in a metabolic and a regulatory sense. This paper discusses some examples of the utility of adipose tissue in the biological systems of organisms.
Hydrolysis and synthesis of triacylglycerol by adipose tissue
Adipose tissue, specifically WAT, is the primary energy reserve in mammals. This tissue has two main functions: lipolysis, the hydrolysis of lipids (primarily TAG) to generate fatty acids; and lipogenesis, the synthesis and storage of TAG. These opposite reactions are regulated to maintain an appropriate body fat level for the animal's environmental lifestyle (Figure 2) (Ahmadian et al., 2007).
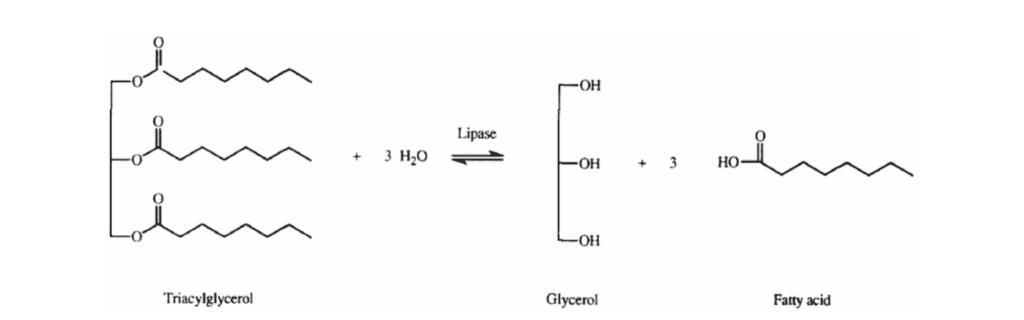
Fig. 2 The hydrolysis or synthesis of a triacylglycerol substrate conducted by a lipase (Abdelmonaem et al., 2010).
Lipolysis
Lipolysis is a catabolic metabolism where TAG hydrolysis occurs to generate fatty acids and glycerol. This process occurs in various tissues but is the most abundant in WAT due to the high concentration of fat molecules and its role in regulating fat in the organism. This metabolism is triggered by fasting and a lack of glucose, which induces a decrease in the body's insulin level. TAG decomposition is essential for bringing the insulin level back to normal, thus satisfying the needs of other organs by supplying fatty acids to the bloodstream, which are transformed into glucose by oxidation (Coleman & Mashek, 2011).
This lipase-based mechanism can be divided into three main steps (Figure 3). The first step involves the enzyme adipose triglyceride lipase (ATGL), which breaks down the triacylglycerol molecules into diacylglycerol and a free fatty acid. The resultant diacylglycerol is then hydrolyzed by hormone-sensitive lipase (HSL), freeing another fatty acid to give a monoacylglycerol molecule. These two steps constitute 95% of lipolysis. Finally, monoacylglycerol lipase (MGL) acts on the monoacylglycerol, removing the third fatty acid and producing a single glycerol molecule (BD Editors, 2018).

Fig. 3 Schematic representation of the lipolytic pathway. TG: triglyceride/triacylglycerol; ATGL: adipose TG lipase; DG: diacylglycerol; FFA (Free Fatty Acid): free fatty acid; HSL: hormone-sensitive lipase; MG: monoacylglycerol; MGL: monoglyceride lipase (Nielsen et al., 2014).
Beyond the adipose tissue
After lipolysis, the free fatty acids and glycerol molecules enter the bloodstream to reach various tissues and organs, where they are reacted to form glucose and produce energy. In these organs, the fatty acids enter each cell's mitochondria and are oxidized by a metabolic process called beta-oxidation. As shown in Figure 4, beta-oxidation consists of a series of redox reactions conducted by enzymes on fatty acids and intermediate molecules such as FAD2, NAD+ and COA-SH. Its product is acetyl-CoA molecules, and with each reaction, a small amount of adenosine triphosphate (ATP), the cell's primary energy molecule, is generated.
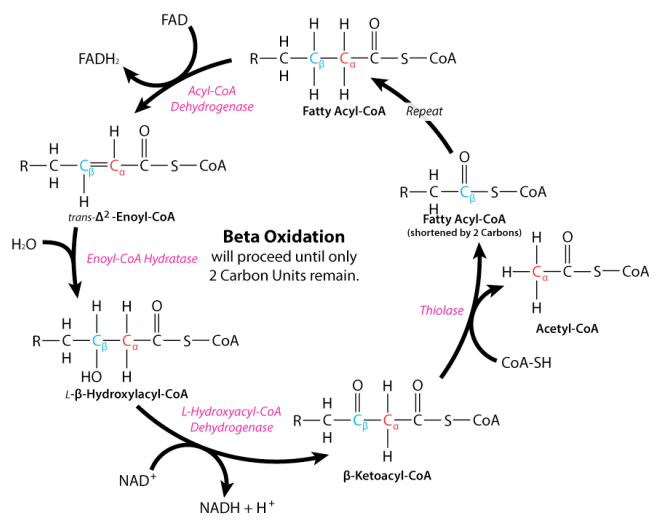
Fig. 4 Beta oxidation of fatty acids (Ahern & Rajagopal, 2016).
The fate of the acetyl-CoA obtained from fatty acid oxidation depends on the needs of an organism and the type of mammal it is. If the body needs energy, acetyl-CoA molecules can enter the citric acid cycle and be oxidized to produce energy. This citric acid cycle is an aerobic metabolic pathway occurring mainly in the smooth muscle cells' mitochondria. It uses a two-carbon molecule (acetyl-CoA) and a four-carbon molecule to form a six-carbon molecule used to generate high-energy electron carriers (NADH and FADH2) and GTP. Although the energy production from fat molecules is slower than the production from carbohydrates (since glucose catabolism is available immediately), fat is considered the most energy-efficient type of food. Each triglyceride molecule generates three fatty acid molecules (of up to 16 carbons each). Therefore, fat molecules produce more energy than carbohydrates and are an essential source of energy for the body.
On the other hand, the glycerol obtained from triglycerides after lipolysis is released in the bloodstream and taken up by the liver. This molecule is phosphorylated by glycerol kinase and oxidized into dihydroxyacetone phosphate (DHAP) (an intermediate of gluconeogenesis) by glycerol phosphate dehydrogenase. The product then undergoes glucogenesis, generating the glucose needed for the citric acid cycle and the electron transport chain. Although both these metabolic pathways take place in the liver, they occur primarily in the smooth muscle cells' mitochondria and in blood cells (Chourpiliadis & Mohiuddin, 2022).
Lipolysis in camelids
The camel (genus Camelus) is a large ruminating hoofed mammal of arid Asia and Africa. They are best known for their ability to withstand harsh conditions in arid environments where food is scarce. A common misconception is that the hump of a camel is filled with water. However, the hump of a well-fed and rested camel consists exclusively of adipose tissue, and its weight can reach up to 25 kilograms (HowStuffWorks, 2009). Studies by Faye et al. (2012) show that the hump is the most representative organ in terms of body fat storage as it can store, on average, 44% of the total fatty reserves of an animal. The fat constitutes 84% of the hump tissue. In the dromedary hump, the fat is composed of two-thirds unsaturated fatty acids and one-third monounsaturated fatty acids. A small quantity of polyunsaturated fatty acid is also present (about 2%) (Sahraoui et al., 2015).
Thanks to their evolutionary adaptation, camels can efficiently use dietary energy and even starve for long periods. They utilize their fatty acid storage for their energy and glucose requirements through lipolysis (Wensvoort et al., 2001). In fact, the animals can survive a 40% weight loss thanks to their fat reserves (San Diego Zoo Animals & Plants, n.d.). During travels, the hump can deflate, droop, and even disappear (Figure 5). Nevertheless, the hump will return to its upright position once the camel has access to food.
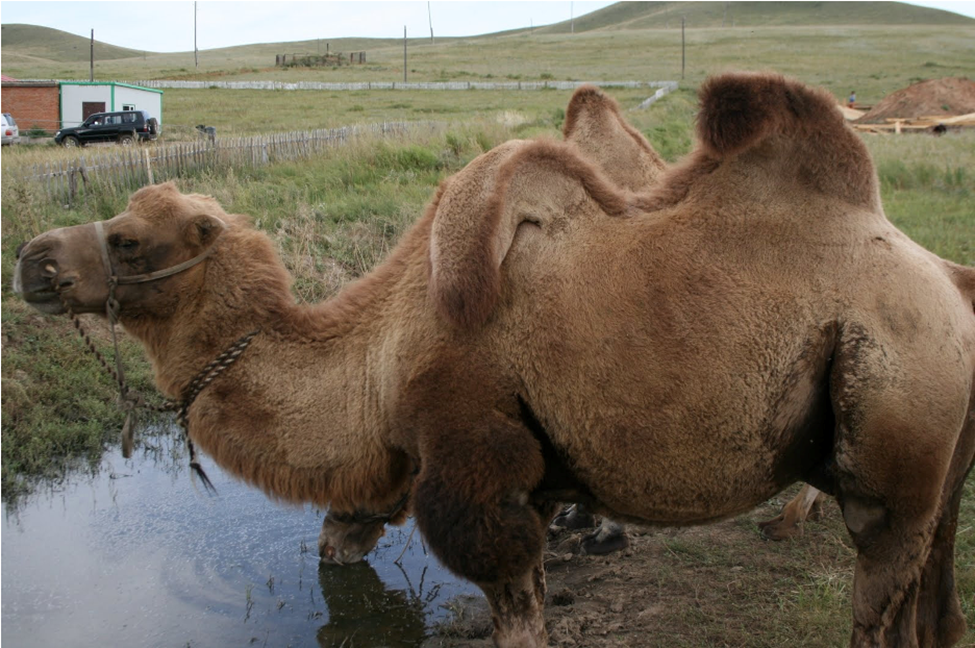
Fig. 5 Camel with a limp hump due to starvation (Shellman, n.d.).
Lipogenesis
Lipolysis and lipogenesis are opposite processes. While lipolysis is the hydrolysis of triacylglycerol, lipogenesis is its synthesis from glucose or other substrates. Also known as de novo lipogenesis, this specific biosynthesis occurs predominantly in the liver, but also in the adipose tissue.
Lipogenesis takes place in both the mitochondria and the cytoplasm of the adipocyte. After entering the cell, glucose is immediately transformed into pyruvate by glycolysis. Pyruvate then undergoes the citric acid cycle in the mitochondria to produce citrate, which synthesizes acetyl-CoA with the help of the ATP-citrate lyase enzyme. This leads to fatty acid synthesis, characterized by the repeated addition of two-carbon units to acetyl-CoA to form complex fatty acids. In triglyceride synthesis, three fatty acids are esterified to glycerol. Esterification consists of combining an organic acid with the three fatty acid molecules and alcohol (glycerol) to form an ester (triglyceride molecules) and water (Figure 6) (Ojha et al., 2014).
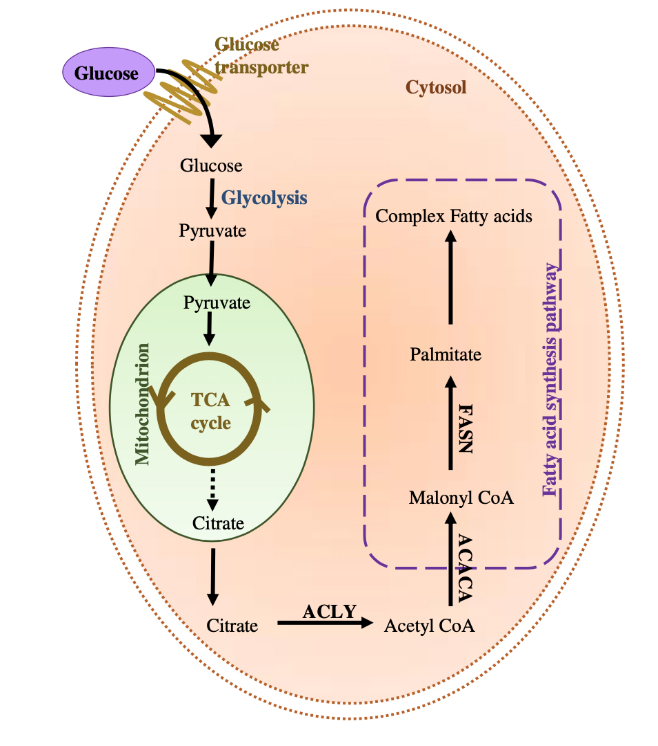
Fig. 6 De novo lipogenesis in an adipocyte. TCA: the tricarboxylic acid cycle; ACLY: ATP-citrate lyase. ACACA: Acetyl-CoA carboxylase; FASN: fatty acid synthase (enzyme) (De Novo Lipogenesis in Health and Disease, 2014).
Lipogenesis and lipid storage are major metabolic requirements for hibernation, giving animals the ability to survive extreme conditions. The animals will consume an enormous amount of food that will lead to fatty acid synthesis and storage in their adipose tissue, which will be used slowly during hibernation. This is showcased in the body and lipid mass increase of the Richardson's ground squirrel Spermophilus richardson (Figure 7) in the months leading up to hibernation. These types of squirrels can hibernate as early as July until approximately the month of March (Richardson's ground squirrel, n.d). Figure 8 highlights the changes in their body and lipid mass during their pre-hibernation period. The ground squirrels' body masses (male, parous female, and non-parous female) increase around May 10, approximately two months before their hibernation period. The variations in body mass seen in the figure are prompted by the increase of lipid mass around the same period indicating lipogenesis and lipid storage in preparation for their dormancy state.
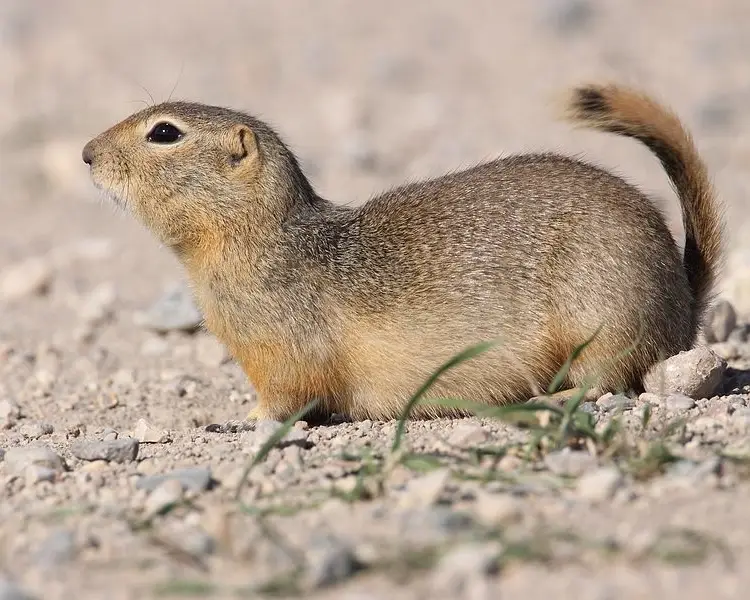
Fig. 7 Picture of a Richardson's ground squirrel (Richardson's ground squirrel, n.d).
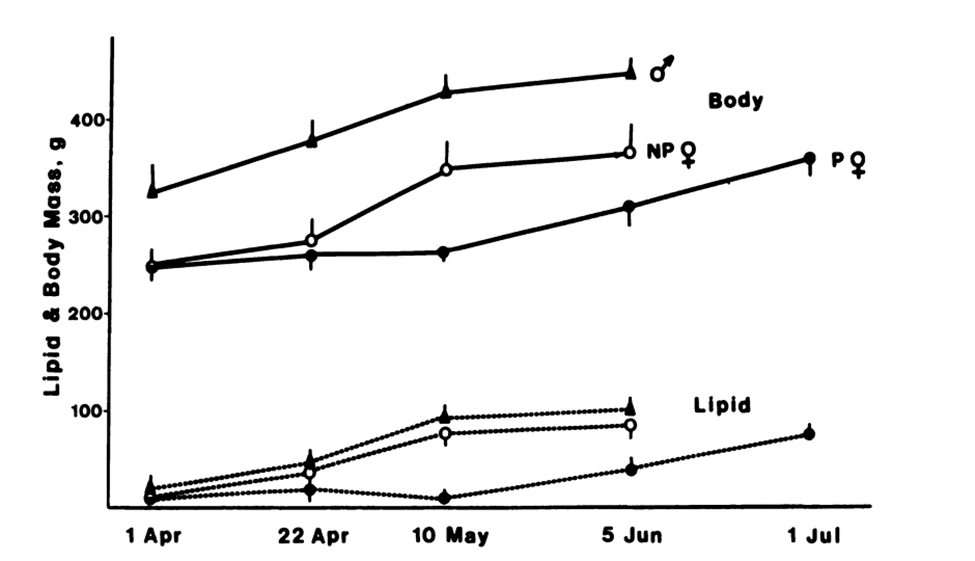
Fig. 8 Whole-body mass (solid lines) and whole-body lipid mass (dotted lines) of Richardson's ground squirrels (University of Chicago Press Journals: Cookie Absent, 1989).
In summary, adipose tissue is vital in regulating fat levels according to the body's needs. When the body lacks glucose and experiences low insulin levels (fasting state), lipolysis is induced in the adipose tissue, converting triacylglycerol into fatty acids, which then enter the bloodstream to fulfill different functions in other organs. However, when the body is experiencing an excess of glucose, lipogenesis is induced to form triacylglycerol using fatty acid synthesis. The triglyceride molecules are then stored in the adipose tissue for future needs.
Importance of metabolic water in animals
Metabolic water
Water is essential for maintaining life; it is the most critical macronutrient in the animal body and is needed in greater quantity than any other orally ingested substrate. Its significance is evidenced by its essential roles in chemical reactions, metabolic processes, temperature regulation, and even the elimination of waste from the body (Cherian, 2020). Several factors influence an animal's water requirements. These include the animal's species, its physiological condition (such as age, pregnancy, growth, and ability to conserve water), its diet, its level of activity, and water quality and availability. Ambient temperature and humidity are also significant factors contributing to water intake (Subcommittee on Environmental Stress, 1981).
Animals' primary sources of water include drinking water, water that presents itself as moisture, and metabolic water. The latter consists of water resulting from living organisms' oxidation of organic materials. This water source, termed “metabolic” or “oxidation”, is sometimes the sole water source. This is the case for hibernating animals and animals inhabiting arid environments where water is scarce, and the water content in the food present is low (Edney, 2012).
The substrates oxidized by animal metabolisms determine the amount of water produced. As shown in Figure 9, carbohydrate, fat and protein oxidation produces 56, 107 and 40 grams of water per 100 grams consumed, respectively (Edney, 2012). Thus, it is easy to assume that an animal would gain weight while metabolizing fat reserves (as water is formed using atmospheric oxygen), were it not for the different processes that lead to the loss of water, such as respiration, urine and feces output, and evaporation.
The reactions of oxidation of the three major substrates can be shown as below (Candlish, 1981):
Fat: C52 H10406 + 75 02 → 52CO2 + 52H2O
Glycogen: C6H10O5 + 6O2 → 6CO2 + 5H2O
Protein: C362H586N104O104S → 52H2NCONH2 + 310CO2 + 188H2O + H2SO4
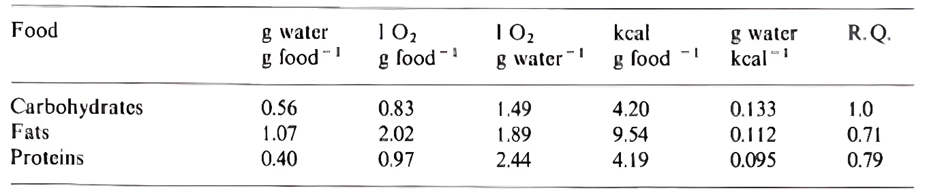
Fig. 9 The oxidation characteristics of the three significant substrates: carbohydrates, fats, and proteins. Values are representative approximations as they vary between foods in the same class (Edney, 2012).
Schmidt-Nielsen (1964) disputes the idea that the amount of water gained in fat oxidation is more significant than that achieved by the oxidation of carbohydrates and proteins. He claims that it is essential to consider oxygen consumption, since the more oxygen required for the oxidation of specific substrates, the more water is lost from the lungs (Candlish, 1981). These values are considered in Figure 9. Because of this, the type of substrate oxidized depends on the specific environment and conditions the animal is in. Two cases will be studied, the metabolic strategies of arthropods and those of the spinifex hopping mouse.
Water regulation in arthropods
Metabolic water is essential for arthropods living in dry environments. For instance, certain species can regulate their water content by varying the proportion of fat consumed. Fasting tsetse-flies oxidize more fat when the air is dry. This increase in metabolic water produced by fat hydrolysis is characterized by an increase in oxygen demand (Figure 9) which could lead to an increase in ventilation (Edney, 2012). Experiments on Locusta by Loveridge and Bursell (1975) found that a shift towards the oxidation of a more significant proportion of fat occurs during dehydration or fasting.
Aphids are highly active insects that can fly continuously for several hours (Figure 10). A study by Cockbain (1961) measures the evaporative water loss during tethered flights of aphids. It is shown that during 6-hour long flights at 25-26°C and a relative humidity level of 57-82%, an aphid weighing 700 μg loses around 9% of its wet weight. During this flight period, about 32 μg of water is generated following the oxidation of about 30 μg of fat. Thanks to the processes of metabolic water, the percentage of water content before and after the flight is almost identical (70%), and it is estimated that only 1% of the total body mass was lost per hour (Cockbain, 1961). This demonstrates the animal's ability to counterbalance the additional evaporative loss during flight by increasing the water production rate through the metabolism of fats (Figure 11).
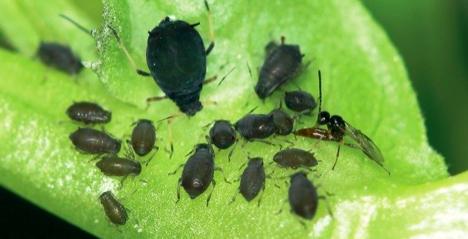
Fig. 10 An image of the aphids Aphis fabae (Vorburger et al., 2013).
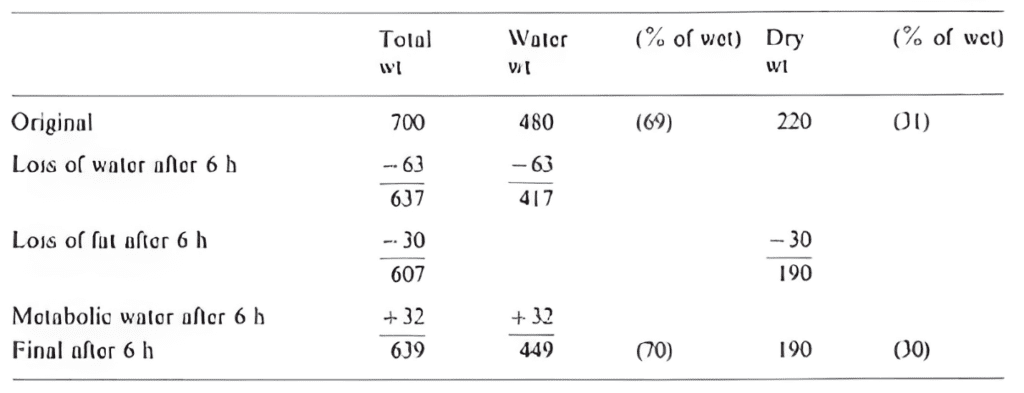
Fig. 11 Variations of the water content of Aphis fabae during a six-hour flight at 25-26°C and a relative humidity of 57-82% (adapted from Cockbain, 1961).
Metabolic strategies of the spinifex hopping mouse
Like many other desert animals, Notomys Alexis, the spinifex hopping mouse (Figure 12), can withstand arid conditions by maintaining water balance without drinking water. This species inhabits the arid zones of central and western Australia. A study performed by Takei et al. (2012) reveals the mechanisms for appetite regulation and energy and water metabolism that are essential for the survival of these desert rodents in such arid environments.
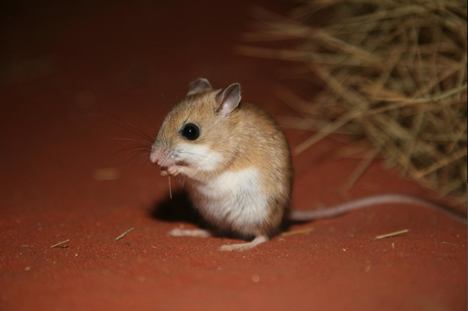
Fig. 12: Image of the Spinifex Hopping mouse (Barritt, 2008).
It is noted that Notomys, like many animals in similar environments, can preserve water through different channels: they produce highly concentrated urine, reduce fecal water content, and reduce respiratory loss through nasal cooling systems. Still, this is insufficient to achieve water balance as the water lost during respiration, urine, and fecal production must be compensated. This is where metabolic water comes into play.
In normal conditions where water is available, a large amount of adipose tissue is accumulated in the abdomen of the rodent. Their diet comprises mainly millet seeds that contain 73% carbohydrates as nutrients. In this state, they metabolize carbohydrates into lipids for fat storage. However, during long periods of water deprivation in arid environments, lipid oxidation might not be the most effective. As previously stated, lipids produce a significantly greater amount of oxidation water than carbohydrates. However, the latter makes 20% more water than fat per kilocalorie because they require less oxygen than fat (Takei et al., 2012). The metabolic strategy of the mouse switches from lipid metabolism to carbohydrate metabolism to minimize respiratory water loss in such dry environments and to enhance the water production per energy molecule.
The changes in metabolic strategies are shown to induce changes in the body fat of the rodent. In the study by Takei et al. (2012), the rodents' body masses tended to decrease until the seventh day of water deprivation and increase after over ten days in a state of deprivation (Figure 13a). The body fat percentage decreased linearly and became undetectable after 12 days (Figure 13b).
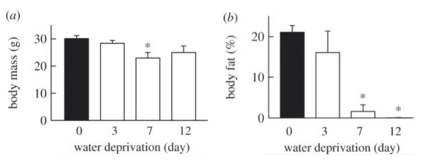
Fig. 13 Variations in (a) body mass and (b) the percentage of body fat in the Spinifex Hopping Mouse depending on the number of days the rodent is subjected to water deprivation (adapted from Takei et al., 2012).
Ultimately, the desert rodent presents a particular physiological adaptation to its harsh living conditions. A water-deprived hopping mouse increases its food intake to increase the available substrates for metabolic production. The switch of metabolic water substrate from fat to carbohydrates and the mechanisms to decrease water loss help achieve water balance in the desert.
To summarize, some animals regularly face a significant physiological problem: obtaining the water required for the normal functioning of their bodies. One of the mechanisms used to resolve this issue is the water metabolism of specific substrates. Different substrates are used depending on their advantages. By altering metabolic activity and thus body fat composition, an animal can reach water balance and even thrive in water-deprived environments.
Thermogenesis in brown adipose tissue
Thermogenesis is defined as the production of heat energy. It is a crucial component in maintaining body temperature in mammals and birds as it is stimulated in cold environments. The primary sources of heat production in said animals are mitochondrial oxidation in BAT (nonshivering thermogenesis), an increased heart rate, and shivering in the skeletal muscle (Morrison et al., 2008).
Nonshivering thermogenesis is defined as an increase in metabolic heat production not associated with muscle activity. The heat produced by nonshivering thermogenesis is mainly a by-product of fatty acid metabolism. This process occurs via brown fat metabolism as well as in skeletal muscle, liver, brain, and white fat, and is governed by a signaling cascade initiated by the hypothalamus. The latter sends signals through the sympathetic nervous system, which are transmitted to the BAT cell in the form of a norepinephrine stimulus, an organic chemical that functions as both a hormone and neurotransmitter (Motoyama & Davis, 2005).
After norepinephrine reaches the cell receptor, several consecutive chemical reactions occur. This adrenergic stimulation prompts the lipolysis of cytoplasmic lipid droplets, in particular triglyceride molecules, upon BAT to form fatty acids. These long-fatty acids then enter the mitochondria and activate the mitochondrial uncoupling protein 1 (UCP1), a BAT-specific transport protein of the inner mitochondrial membrane, also known as thermogenin. Upon activation, UCP1 increases the conductance of the inner mitochondrial membrane (IMM) to make BAT mitochondria generate heat rather than ATP in their inner membrane (Fedorenko et al., 2012). UCP1 provides an alternative pathway by which protons can re-enter the mitochondrial matrix. It generates the proton gradient, which usually induces ATP synthesis from the electron transport chain in mitochondrial respiration. This causes the mitochondria to produce relatively smaller amounts of ATPase, so all the free energy released by substrate oxidation and the proton motive force is forcefully released as heat. Thus, UCP1 is considered the essential element of BAT thermogenesis, and in its absence, no heat is produced in brown fat cells (Figure 14) (Ikeda & Yamada, 2020).
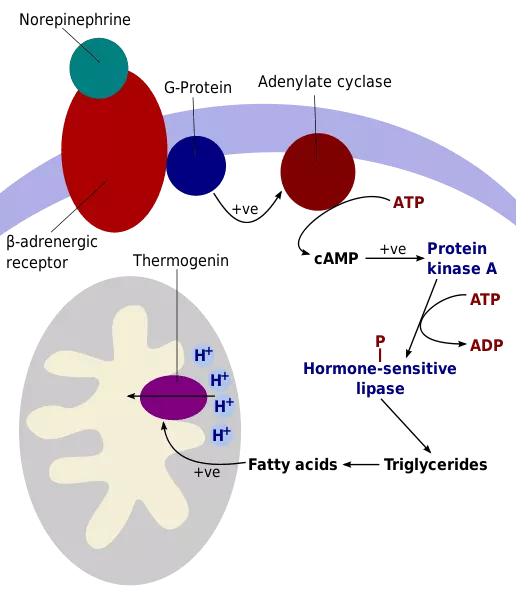
Fig. 14 Illustration of the nonshivering thermogenesis steps in brown adipocyte tissue (Knapp, 2020).
Brown adipocytes are the main driver of nonshivering thermogenesis due to the large number of cytoplasmic mitochondria compared to other types of adipocytes (Figure 15). These mitochondria are densely packed with cristae and have an increased content of respiratory-chain components. A crista is a fold in the mitochondrion's inner membrane, which increases the mitochondrion's ability to synthesize ATP and generate heat. In fact, brown adipocytes are unique in their ability to uncouple oxidative phosphorylation, resulting in heat production instead of generating ATP (Paumard et al., 2002).
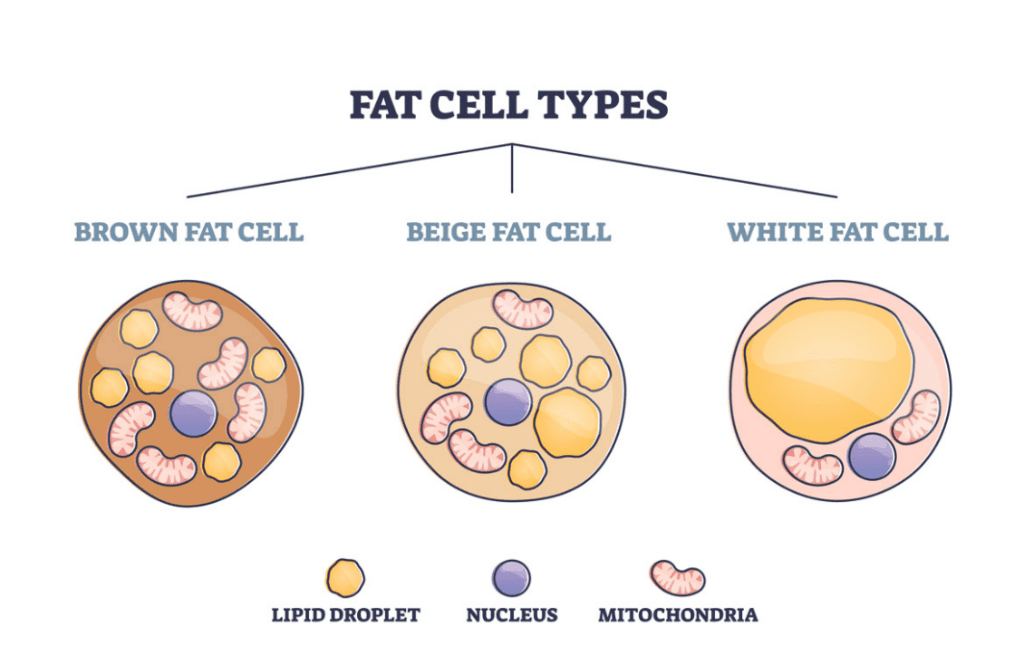
Fig. 15 Comparison of the composition of brown, beige and white adipocytes (MDVIP, 2022).
The case of the thirteen-lined ground squirrel (Ictidomys tridecemlineatus)
In natural hibernators, lipid catabolism by BAT is the most efficient way to oxidize fat per mass of tissue. In the autumn, the accumulation of thirteen-lined ground squirrels' (Ictidomys tridecemlineatus, Figure 16) lipid mass increases the BAT mass. At specific points throughout the hibernation period, BAT uses the stored lipids (triacylglycerides) to produce heat and initiates arousal from hypothermic torpor bouts. These re-warming torpor interruptions are termed interbout arousals (IBAs) and typically occur every one to two weeks to resume normothermic metabolic activities for a short period. These bouts are triggered by nonshivering thermogenesis (Ballinger & Andrews, 2018). In order to produce the heat required for the multiple IBAs, the mammals accumulate BAT during the autumn and winter months.
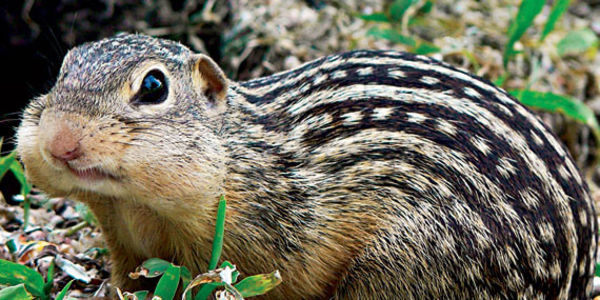
Fig. 16 Image of the thirteen-lined ground squirrel (Ictidomys tridecemlineatus) (Sunday Squirrel Spotlight: The Thirteen-lined Ground Squirrel, 2018).
To sum up, mammals can withstand cold temperatures partly due to nonshivering thermogenesis initiated by BAT. This process inducing lipid catabolism is nature's most effective way of adapting to cold environments by regulating body temperature. As described above, BAT contains numerous mitochondria and proteins involved in the energy producing machinery, which reduce the amount of ATP synthase and increase the amount of heat released.
The machinery of fat can be compared to that of a motor, transforming energy and dissipating heat. In the perspective of an engineered motor, the latter is usually an undesirable product. Although it may seem counterintuitive that a motor would benefit from the dissipation of lots of heat, this effect is vital in fat cells in certain circumstances. Fat is fine-tuned for environmental challenges as it diversifies its function to adapt to the needs of the animal. Both BAT and WAT run on the same substrate, but the ability to uncouple oxidative phosphorylation when the production of heat is needed is unique to brown fat cells and is considered a “side branch” of fat functions that certifies its diversity. This is an adaptation that illustrates the famous principle in nature: minimum inventory, maximum diversity.
Adipocytes as an endocrine organ
There are four classic hormone categories: steroids, polypeptides, amino acid-derived compounds, and fatty-acid derived compounds (Norman & Litwack, 1987). The chemical structures of these hormones vary significantly both from category to category and between molecules within a category. They fulfill a wide variety of tasks in a wide variety of bodily systems. The common ground of all these regulatory molecules is the fact that they are excreted from organs and glands throughout the body, including the posterior and anterior pituitary glands, the thyroid, the intestines, the brain, the kidney, the luteal, and adipose tissues (Norman & Litwack, 1987; Rosen & Spiegelman, 2006).
Adipocytes, primarily those in WAT, are an important endocrine organ that performs many functions in animals, including the regulation of fat mass, nutrient homeostasis, blood pressure, hemostasis, bone mass, and thyroid and reproductive functions (Rosen & Spiegelman, 2006). WAT accomplishes these tasks by releasing a variety of signals, protein factors (adipokines), and fatty acids. WAT adipocytes also release and produce prostanoids, and release cholesterol and retinol, though these are not produced in the cells (Trayhurn, 2005). There is also evidence that adipose tissue is a site for steroid biosynthesis, where base molecules such as cholesterol are transformed into more complex steroids, including sex steroids such as estrogen (Li et al., 2015).
Energy balance system
One system in animals that is regulated with large contributions from adipose tissue is the energy balance/glucose homeostasis system. The primary purpose of this system is to maintain constant blood glucose levels, so that cells can receive glucose to transform into energy to carry out their necessary functions. The system involves compounds such as insulin, which inhibits glucose synthesis from glycogen; glucagon, adrenaline, and corticosteroids, which promote glucose synthesis from glycogen; as well as a whole host of other hormones, processes, and regulatory mechanisms (Rosen & Spiegelman, 2006). Figure 17 shows some of the hormone excretions from adipocytes that impact blood glucose levels.
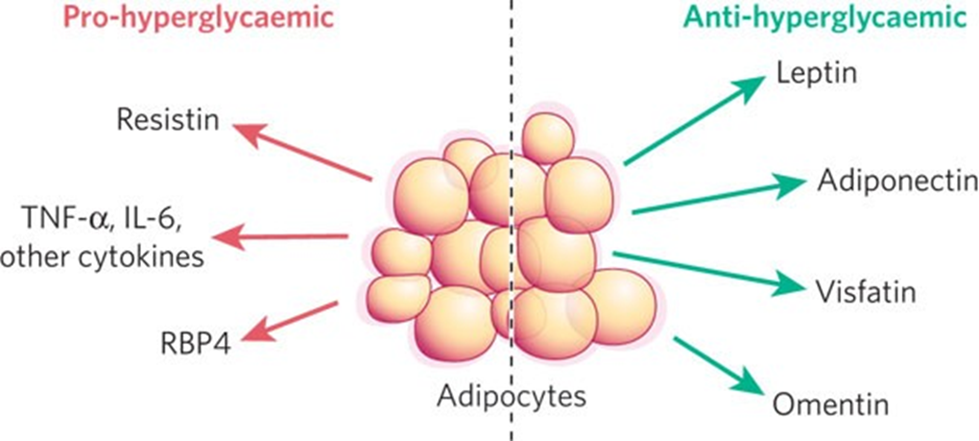
Fig. 17 An illustration of certain pro-hyperglycemic and anti-hyperglycemic hormone secretions from WAT that work to regulate blood glucose levels in organisms (Rosen & Spiegelman, 2006).
One contribution of adipose tissue to maintaining a constant blood glucose level is mentioned in this paper: the metabolism of fatty acids into the bloodstream to be used by organs for energy. The synthesis of glucose from fatty acids is much more efficient than the synthesis of glucose from glycogen, and thus the release of lipids from adipocytes into the blood stream is an effective method of raising an organism's glucose levels (Rosen & Spiegelman, 2006).
Leptin
WAT secretes many types of molecules and hormones that impact the energy balance system in response to signals received by adipocytes (Berg et al., 2001). The most studied is leptin, an anti-hyperglycemic adipokine secreted by both white and brown adipocytes, though primarily by WAT (Rosen & Spiegelman, 2006; Trayhurn, 2005).
There is a high concentration of leptin receptors in the mediobasal hypothalamus, which, when stimulated by leptin, regulates appetite in organisms (Rosen & Spiegelman, 2006; Trayhurn, 2005). This function dictates when an organism feels the need to eat and thus supplement its body's glucose levels through food. As ingestion of nutrients is the primary method by which animals elevate and maintain bodily glucose/energy levels, this function is extremely important to blood glucose homeostasis.
As shown in Figure 17, leptin is also an anti-hyperglycemic hormone, meaning that it acts to suppress high levels of glucose in the bloodstream. It accomplishes this by increasing insulin sensitivity in organs such as muscle and the liver, allowing insulin to inhibit glucose synthesis from glycogen more effectively, thus keeping blood glucose levels down (Rosen & Spiegelman, 2006). Leptin is very efficient in this role and has been shown to significantly impact blood glucose content. Studies have shown that blood glucose homeostasis is improved by adding leptin to the bloodstreams of lipodystrophic mice, showing that a lack of adipose tissue negatively impacts glucose homeostasis through a lack of leptin secreted (Rosen & Spiegelman, 2006).
Acrp30
Another hormone synthesized and released by WAT is Acrp30. Acrp30 is a protein found naturally in animals, and acts as an anti-hyperglycemic molecule in the homeostasis of animals. As with leptin, Acrp30 has been shown to sensitize the liver to insulin, thus reducing hepatic glucose output and keeping blood glucose levels down (Berg et al., 2001).
Berg et al. (2001) studied the effects of Acrp30 injected into mice. They found that in general, injecting the protein into the animals triggered a transient decrease in blood glucose levels, which was eventually counteracted by the mice's bodies' natural glucose homeostasis system. The study also discussed the effects of Acrp30 in diabetic mice, with diabetes that could be linked to obesity or lipodystrophic states. By enhancing insulin action, Acrp30 was effective in reducing hyperglycemic conditions, and helped to regulate the systems of these diabetic mice.
Acrp30 is an important molecule for glucose homeostasis in that it sensitizes organs to insulin, but it may also play other roles in the maintenance of an animal's energy balance. Acrp30 concentrations in the bloodstream are typically constant, and much higher than the leptin concentrations in the bloodstream. This implies that though the two molecules share one common use, they vary in their other uses. Acrp30 may have effects on glucose disposal in muscle and adipose tissue and may also trigger adaptive responses when found in high concentration during food restriction (Berg et al., 2001).
The body energy system is a prime example of a biological system with specific needs: the bloodstream must have a constant glucose concentration for organs to be able to properly utilize the glucose for energy and maintain healthy systems. Pro-hyperglycemic functions must work in tandem with anti-hyperglycemic functions to maintain the perfect balance of all the system's components. Adipose tissues are a critical component of the mechanism to satisfy these demanding conditions. Lipid secretions in response to signals act to directly increase blood glucose levels, while hormone secretions from adipose tissues such as leptin and Acrp30 control appetite, insulin levels, and insulin sensitivity (Berg et al., 2001; Rosen & Spiegelman, 2006; Trayhurn, 2005).
The bodies of animals require very specific conditions to function properly, which are difficult to maintain. In order to self-regulate such complex systems, organisms have developed negative feedback loops, where steps earlier in the reaction chain of a system inhibit later steps in the system, thus regulating overall reaction rates. If there is too much of one substance, the entire system could be thrown out of balance, and the system might not work. Because of this, biological systems necessitate a perfect balance of all the components in the system. Multiple negative feedback loops must work together to ensure the system functions properly and all molecules have the right concentrations. Adipose tissue is integral to evolution's biological systems' regulation mechanism, working to keep concentrations of molecules at just the right levels for systems to function.
Non-metabolic function of fats in bone marrow
Alongside their other utilities, adipocytes present in bone marrow are involved in the regulation of blood cell levels and blood pressure. Through different mechanisms, these adipocytes help to control these two important parameters of the bloodstream in an animal.
Bone marrow
Bone marrow is present in substantial quantities in mammals. Bone marrow makes up about 2% of the body mass of a dog and 3% of the body mass of a mouse. Bone marrow is found in the center of long bones such as in those of the arms and legs (Figure 18), and in the cavities of axial bones such as the ribcage and the skull. It primarily comprises a mixture of hematopoietic tissues (tissues used in the formation of blood), adventitial cells (cells lining the outside of blood vessels), and adipocyte fat cells. Examples of hematopoietic tissues are blood cells and their precursors, hematopoietic stem cells. The main function of bone marrow is to produce red and white blood cells and platelets, a process called hematopoiesis. Consequently, bone marrow is highly vascularized, with many arteries and venules running parallel to the marrow or in the marrow cavities. These channels act to transport nutrients to the marrow as well as to transport the newly formed cells out of the marrow and into circulation. Additionally, bone marrow can store a lot of energy due to its high content of adipocytes. These two roles – blood cell production and energy storage – give rise to two distinct types of bone marrow: red bone marrow and yellow bone marrow, each specialized for their respective role (Travlos, 2006).
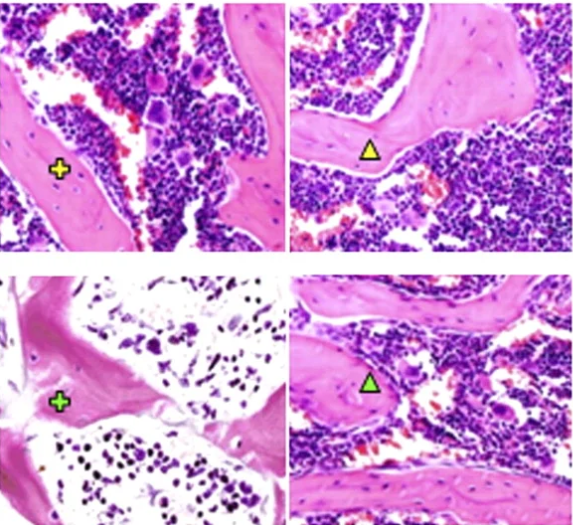
Fig. 18 Bone marrow in the femur of a mouse (Cross: proximal femur; Triangle: Distal femur; Yellow: left femur; Green: right femur) (Cao et al., 2011).
Red marrow (medulla ossium rubra)
At birth, all bone marrow is red marrow, but with age, this red marrow undergoes adipose conversion and turns into yellow marrow. During adulthood, red marrow is mainly found in spongy bones, which are located at the end of long bones (see Figure 19), and axial bones. It holds most of the hematopoietic tissues and hematopoietic stem cells; consequently, it fulfills the blood cell production role of bone marrow, producing red and white blood cells and platelets. The marrow's red color comes from its high concentration of hemoglobin, a protein found in red blood cells. Finally, as red blood cells get old or damaged, red marrow is also responsible for the filtering, recycling, and disposal of these non-functioning blood cells (Lakna, 2006). The life span of these red blood cells varies between species; in chickens, for example, the life span of red blood cells is 35 days on average, while the red blood cells in ducks have a life span of approximately 42 days (Rodnan et al., 1957).
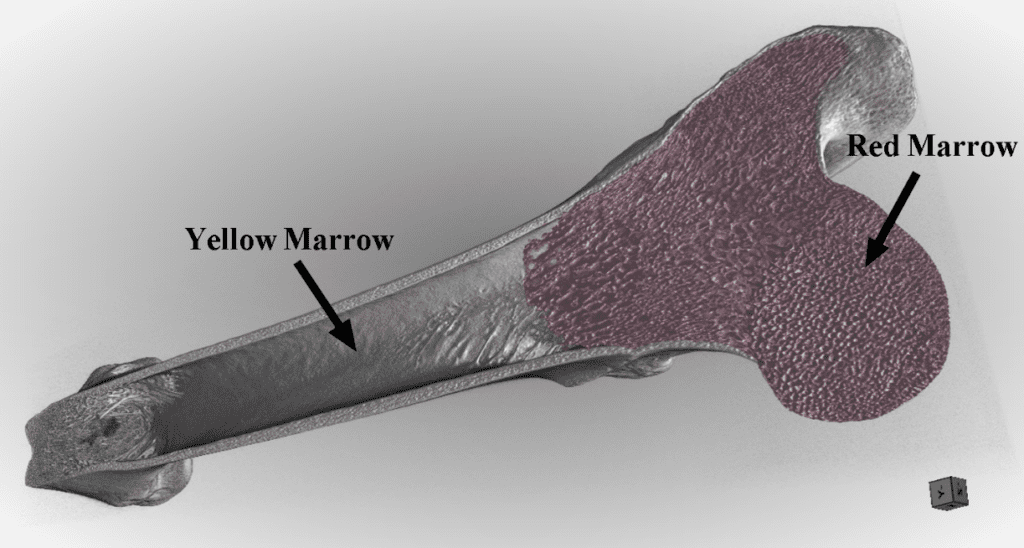
Fig. 19 Anatomy of the long bone (courtesy of Professor Natalie Reznikov, McGill University).
Yellow marrow (medulla ossium flava)
Yellow marrow is found in the shaft of the long bones and is filled with adipocytes. This high number of fat cells gives the marrow its yellow color and allows yellow marrow to be a last resort energy source in cases of extreme starvation. As with red marrow, stem cells (called mesenchymal stromal cells) are stored in the yellow marrow. However, these stem cells do not mature into blood cells; instead, they mature into other adipose cells, cartilage, or bone cells. Finally, in situations where an urgent boost of erythrocyte production is necessary (as a consequence of blood loss after a trauma or birthing, or in hypoxic conditions such as migration to a higher altitude), yellow marrow can be converted back into red marrow (Lakna, 2006).
Adipocyte functions in bone marrow
Bone marrow adipose tissues composed of bone marrow adipocytes (BMA) (Figure 20), also known as yellow adipose tissues, make up around 45% of the mass of red marrow and 90% of the mass of yellow marrow. They fill cavities inside the bone, such as the pores found in trabecular bone and are used as an energy source for the hematopoietic cells and osteoblasts (cells that form bone tissue). Not much is known about bone marrow adipocytes as they are a new subject of research. That said, bone marrow adipocytes serve other purposes.
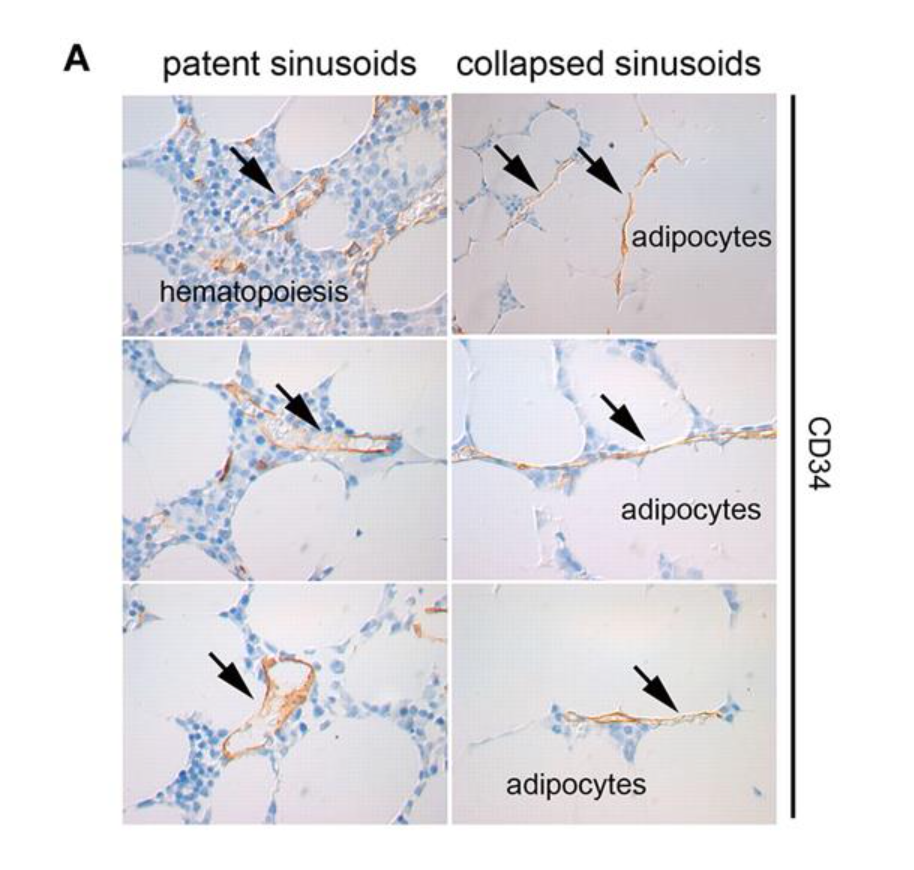
Fig. 20 Bone marrow adipocytes (white blobs) with arrows pointing to blood vessels constricted by the adipocytes (Suchacki et al., 2020).
As erythrocyte production varies depending on needs, blood flow also needs to be regulated to allow for this increase in production. Consequently, one function of BMA is their role as blood-flow regulators within the bone marrow. Adipocytes can be formed from adventitial reticular cells, thin cells lining the blood vessels in the marrow. After hematopoiesis, adventitial reticular cells in the red marrow start to accumulate lipids and turn into adipocytes. These thin adventitial reticular cells are thus replaced by comparatively large adipocytes, causing the vessels to constrict, which leads to the vessel collapsing. This blocks the flow of blood through the vessel, and effectively cuts off the vessel from the circulatory network. In this sense, adipocytes are used to “prune” the blood vessels. This so-called pruning is non-damaging and reversible. As the vessel's cell walls are not destroyed in the process (Figure 21), these blood vessels can be reopened and used at any time. Through lipolysis, the adipocytes that once blocked off a vessel can be broken down and once again allow blood to flow through the previously constricted vessels (Bianco, 2011).
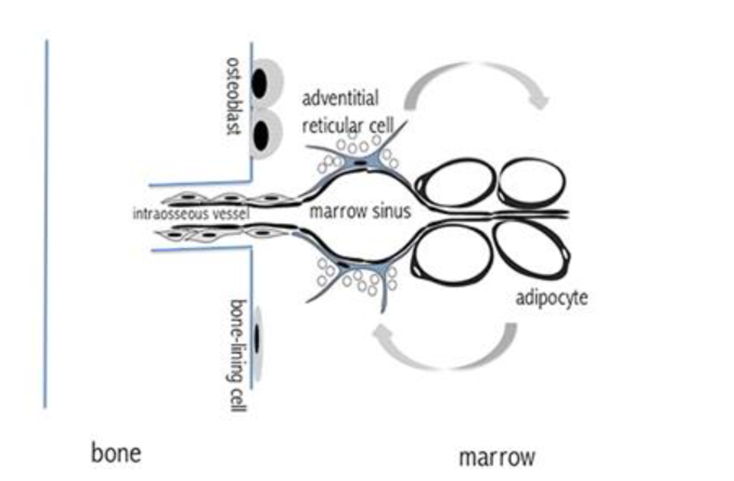
Fig. 21 Adipocytes constricting the vessels within the bone marrow (Bianco, 2011).
Finally, adipocytes may play a part in the conversion of yellow marrow into red marrow during high blood cell demand. The lack of hematopoiesis in yellow marrow either means the hematopoietic stem cells in the yellow marrow are inhibited, or they are dormant, and their activators are inhibited. In mice, for example, the stem cells themselves are inhibited. These adipocytes may be inhibitors of hematopoietic stem cells; if this is the case, then the lipolysis of these adipocytes would allow the yellow marrow to be converted into red marrow (Bianco, 2011).
To summarize, fats in the form of adipocytes are a key component of bone marrow and play a key role in hematopoiesis. They help regulate blood-flow within the bone marrow and may play a role in the adipose conversion of yellow bone marrow into red bone marrow, which is crucial to boosting the production of red blood cells. They also serve as valves for blood vessels, opening vessels up during blood production. Molecules such as water or air cannot be used for this purpose as they cannot be controlled or contained as easily. In the case of water, it would diffuse over time and thus stop constricting the vessels. Air can diffuse into the blood vessels if under pressure, and air bubbles trapped in blood vessels lead to air/gas embolism where the vessels are blocked completely by the air bubbles, leading to potential death (NHS, 2020). Fat, however, is easily stored in cell vacuoles and is unable to enter the blood vessels. In addition, it can be easily formed through adipogenesis and broken down through lipolysis. Because of these factors, fats have evolved to become part of an ideal biological tourniquet system for blood vessels in bones, helping to regulate both the blood pressure and blood cell quantities in organisms.
Conclusion
Adipose tissue is an extremely versatile organ that has evolved to fulfill various functions in animals. When energy levels in an animal are low, adipose tissue will respond by metabolizing triacylglycerols to release glycerol and fatty acids that are used to produce glucose, and thus energy, in an animal. Animals in arid environments use the metabolism of fats to produce metabolic water, using lipid metabolism to aid in hydration. A further utility of fat metabolism takes place in animals in cold environmental conditions, such as hibernators, who rely on the metabolism of BAT to produce thermal energy and maintain a certain core body temperature. Beyond metabolism, adipose tissues act as an endocrine organ, secreting hormones to aid in the homeostasis of extremely complex biological systems such as the blood glucose system. Adipocytes also play a regulatory role in bone marrow, where they help manage blood pressure and red blood cell levels in organisms. Overall, adipose tissue is an extremely versatile and flexible organ that has evolved to play a role in countless intricate and diverse biological systems in organisms.
References
Abdelmonaem, M., Hatem, B., Imen, G., & Jeannette, B. H. (2010). Classification of EC 3.1.1.3 bacterial true lipases using phylogenetic analysis. African Journal of Biotechnology, 9(48), 8243–8247. https://doi.org/10.5897/ajb10.721
Ahern, K., & Rajagopal, I. (2016). 6.11: Fatty Acid Oxidation – Biology LibreTexts. Biology LibreTexts. Oregon State University. https://bio.libretexts.org/Bookshelves/Biochemistry/Book%3A_Biochemistry_Free_and_Easy_(Ahern_and_Rajagopal)/06%3A_Metabolism_I_-_Oxidative_Reductive_Processes/6.11%3A_Fatty_Acid_Oxidation
Ahmadian, M., Duncan, R. E., Jaworski, K., Sarkadi-Nagy, E., & Sook Sul, H. (2007). Triacylglycerol Metabolism In Adipose Tissue. Future Lipidology, 2(2), 229–237. https://doi.org/10.2217/17460875.2.2.229
Aryal, S. (2022, September 5). Bone marrow- types, structure and functions. Microbe Notes. Retrieved October 31, 2022, from https://microbenotes.com/bone-marrow-types-structure-and-functions/
Ballinger, M. A., & Andrews, M. T. (2018, March 7). Nature's fat-burning machine: brown adipose tissue in a hibernating mammal. Journal of Experimental Biology, 221(Suppl_1). https://doi.org/10.1242/jeb.162586
Barritt, M. J. (2008). Spinifex Hopping Mouse (Notomys Alexis), sand dune rodent, Central Australia. Flickr. https://www.flickr.com/photos/centralaustralia/2777957326
BD Editors. (2018). Lipolysis – Definition, Mechanism and Process | Biology Dictionary. Biology Dictionary. https://biologydictionary.net/lipolysis/
Berg, A. H., Combs, T. P., Du, X., Brownlee, M. & Scherer, P. E. (2001). The adipocyte-secreted protein Acrp30 enhances hepatic insulin action. Nature Medicine, 7, 947-953. https://doi.org/10.1038/90992
Bianco, P. (2011). Bone and the hematopoietic niche: A tale of two stem cells. Blood, 117(20), 5281–5288. https://doi.org/10.1182/blood-2011-01-315069
Bolsoni-Lopes, A., & Alonso-Vale, M. I. C. (2015). Lipolysis and lipases in white adipose tissue – An update. Archives of Endocrinology and Metabolism, 59(4), 335–342. https://doi.org/10.1590/2359-3997000000067
Candlish, J. (1981). Metabolic water and the camel's hump — a textbook survey. Biochemical Education, 9(3), 96–97. https://doi.org/10.1016/0307-4412(81)90212-0
Cherian, G. (2020, April 14). A Guide to the Principles of Animal Nutrition: Vol. XVIII. Water in Animal Nutrition (1st ed.) https://open.oregonstate.education/animalnutrition/chapter/chapter-18/
Chourpiliadis, C., & Mohiuddin, S. S. (2022). Biochemistry, Gluconeogenesis – StatPearls – NCBI Bookshelf. Biochemistry, Gluconeogenesis – StatPearls – NCBI Bookshelf. https://www.ncbi.nlm.nih.gov/books/NBK544346/
Cao, X., Wu, X., Frassica, D., Yu, B., Pang, L., Xian, L., Wan, M., Lei, W., Armour, M., Tryggestad, E., Wong, J., Wen, C. Y., Lu, W. W., & Frassica, F. J. (2011). Irradiation induces bone injury by damaging bone marrow microenvironment for Stem Cells. Proceedings of the National Academy of Sciences, 108(4), 1609–1614. https://doi.org/10.1073/pnas.1015350108
Cockbain, A. J. (1961). Low Temperature Thresholds For Flight In Aphis Fabae Scop. Entomologia Experimentalis Et Applicata, 4(3), 211–219.https://doi.org/10.1111/j.1570-7458.1961.tb02136.x
Coleman, R. A., & Mashek, D. G. (2011). Mammalian Triacylglycerol Metabolism: Synthesis, Lipolysis, and Signaling. Chemical Reviews, 111(10), 6359–6386. https://doi.org/10.1021/cr100404w
De novo lipogenesis in health and disease. (2014). De Novo Lipogenesis in Health and Disease – ScienceDirect. https://www.sciencedirect.com/science/article/abs/pii/S0026049514001115
Edney, E. B. (2012, February 9). Water Balance in Land Arthropods: Vol. Chapter 8: Metabolic Water. Springer. https://doi.org/10.1007/978-3-642-81105-0
Fedorenko, A., Lishko, P., & Kirichok, Y. (2012, October). Mechanism of Fatty-Acid-Dependent UCP1 Uncoupling in Brown Fat Mitochondria. Cell, 151(2), 400–413. https://doi.org/10.1016/j.cell.2012.09.010
Guo, F., Si, R., Li, Q., Hai, L., Yi, L., He, J., Ming, L., & Ji, R. (2021). Reversible insulin resistance helps Bactrian camels survive fasting. Scientific Reports, 11(1). https://doi.org/10.1038/s41598-021-98234-y
Hernández, A. (n.d.). Adipose Tissue What Is It, Location, Function, and More. Osmosis from Elsevier. https://www.osmosis.org/answers/adipose-tissue
HowStuffWorks Do the humps on camels hold water?. (2009). Do The Humps on Camels Hold Water? HowStuffWorks. https://animals.howstuffworks.com/mammals/question104.html
Ikeda, K., & Yamada, T. (2020, July 28). UCP1 Dependent and Independent Thermogenesis in Brown and Beige Adipocytes. Frontiers in Endocrinology, 11. https://doi.org/10.3389/fendo.2020.00498
Knapp, S. (2020, May 24). Adipose Tissue – The Definitive Guide| Biology Dictionary. Biology Dictionary. https://biologydictionary.net/adipose-tissue/
Lakna. (2017, September 18). Difference between red and yellow bone marrow: Definition, characteristics, function, similarities and differences. Pediaa.Com. Retrieved October 31, 2022, from https://pediaa.com/difference-between-red-and-yellow-bone-marrow/
Latham, K. (2021). Fat Cells – The Definitive Guide | Biology Dictionary. Biology Dictionary. https://biologydictionary.net/fat-cells/
Li, J., Papadopoulos, V., Vihma, V. (2015). Steroid biosynthesis in adipose tissue. Steroids, 103(1), 89-104. https://doi.org/10.1016/j.steroids.2015.03.016
Loveridge, J. P., & Bursell, E. (1975). Studies on the water relations of adult locusts (orthoptera, acrididae). Respiration and the production of metabolic water. Bulletin of Entomological Research, 65(1), 13–20. https://doi.org/10.1017/s0007485300005708
MDVIP (2022, July 11). Understanding the Colors of Fat: How to Convert White to Brown Fat | MDVIP. https://www.mdvip.com/about-mdvip/blog/understanding-colors-fat
Morrison, S. F., Nakamura, K., & Madden, C. J. (2008, June 28). Central control of thermogenesis in mammals. Experimental Physiology, 93(7), 773–797. https://doi.org/10.1113/expphysiol.2007.041848
Motoyama, E. K., & Davis, P. J. (2005, November 21). Smith's Anesthesia for Infants and Children. Chapter 6 https://doi.org/10.1604/9780323026475
Nielsen, T. S., Jessen, N., Jørgensen, J. O. L., Møller, N., & Lund, S. (2014). Dissecting adipose tissue lipolysis: molecular regulation and implications for metabolic disease. Journal of Molecular Endocrinology, 52(3), R199–R222. https://doi.org/10.1530/jme-13-0277
NHS. (2020, December 16). Air or gas embolism. NHS choices. Retrieved November 20, 2022, from https://www.nhs.uk/conditions/air-embolism/
Norman, A. W. & Litwack, G. (1987). General considerations of hormones. In Hormones (pp. 1-48). Academic Press. https://doi.org/10.1016/B978-0-12-521440-7.50004-2
Ojha, S., Budge, H., & Symonds, M. (2014). Adipocytes in Normal Tissue Biology. Pathobiology of Human Disease, 2003–2013. https://doi.org/10.1016/b978-0-12-386456-7.04408-7
Paumard, P., Vaillier, J., Coulary, B., Schaeffer, J., Soubannier, V., Mueller, D. M., Brèthes, D., di Rago, J. P., & Velours, J. (2002, February 1). The ATP synthase is involved in generating mitochondrial cristae morphology. The EMBO Journal, 21(3), 221–230. https://doi.org/10.1093/emboj/21.3.221
Piotrowska, K., & Tarnowski, M. (2021). Bone marrow adipocytes—role in physiology and various nutritional conditions in human and animal models. Nutrients, 13(5), 1412. https://doi.org/10.3390/nu13051412
Richardson's ground squirrel. Richardson's Ground Squirrel – Facts, Diet, Habitat & Pictures on Animalia.bio. (n.d.). https://animalia.bio/richardsons-ground-squirrel
Rodnan, G. P., Ebaugh, F. G., Fox, M. R., & Chambers, D. M. (1957). The life span of the red blood cell and the red blood cell volume in the chicken, pigeon and duck as estimated by the use of NA2CR51O4. Blood, 12(4), 355–366. https://doi.org/10.1182/blood.v12.4.355.355
Rosen, E. & Spiegelman, B. (2006). Adipocytes as regulators of energy balance and glucose homeostasis. Nature 444, 847-853. https://doi.org/10.1038/nature05483
San Diego Zoo Animals & Plants. (n.d.). Camel | San Diego Zoo Animals & Plants. https://animals.sandiegozoo.org/animals/camel#:~:text=A%20camel%20can%20go%20a,Do%20camels%20really%20spit%3F
Sahraoui, N., Errahmani, M. B., Dotreppe, O., Boudjenah, S., Babelhadj, B., Guetarni, D., & Hornick, J. L. (2015). Fatty acids profile of the dromedary hump fat in algeria. Journal of Camel Practice and Research, 22(1), 27. https://doi.org/10.5958/2277-8934.2015.00005.3
Schmidt-Nielsen, K. (1964). Desert Animals. In Physiological Problems of Heat and Water. Oxford University Press. https://doi.org/10.1126/science.144.3619.715.b
Shellman, V. (n.d.). Do you know what's inside the Camel Hump? Quora https://www.quora.com/What-is-a-camel-hump-made-of
Subcommittee on Environmental Stress, Board on Agriculture, National Research Council, S. O. (1981). Effect of Environment on Nutrient Requirements of Domestic Animals. https://www.ncbi.nlm.nih.gov/books/NBK232337/
Suchacki, K. J., Tavares, A. A., Mattiucci, D., Scheller, E. L., Papanastasiou, G., Gray, C., Sinton, M. C., Ramage, L. E., McDougald, W. A., Lovdel, A., Sulston, R. J., Thomas, B. J., Nicholson, B. M., Drake, A. J., Alcaide-Corral, C. J., Said, D., Poloni, A., Cinti, S., Macpherson, G. J., … Cawthorn, W. P. (2020). Bone marrow adipose tissue is a unique adipose subtype with distinct roles in glucose homeostasis. Nature Communications, 11(1). https://doi.org/10.1038/s41467-020-16878-2
Sunday Squirrel Spotlight: The Thirteen-lined Ground Squirrel. (2018, August 19). Squirrel Gazer. https://www.squirrelgazer.com/blog/sunday-squirrel-spotlight-the-thirteen-lined-ground-squirrel
Takei, Y., Bartolo, R. C., Fujihara, H., Ueta, Y., & Donald, J. A. (2012). Water deprivation induces appetite and alters metabolic strategy in Notomys Alexis: unique mechanisms for water production in the desert. Proceedings of the Royal Society B: Biological Sciences, 279(1738), 2599–2608. https://doi.org/10.1098/rspb.2011.2627
Travlos, G. S. (2006). Normal structure, function, and histology of the bone marrow. Toxicologic Pathology, 34(5), 548–565. https://doi.org/10.1080/01926230600939856
Trayhurn, P. (2005). Endocrine and signalling role of adipose tissue: new perspectives on fat. Acta Physiologica Scandinavia, 184(4), 285-293. https://doi.org/10.1111/j.1365-201X.2005.01468.x
University of Chicago Press Journals: Cookie absent. (1989). RCNi Company Limited. https://www.journals.uchicago.edu/doi/pdfplus/10.1086/physzool.62.6.30156218
Vorburger, C., Ganesanandamoorthy, P., & Kwiatkowski, M. (2013). Comparing constitutive and induced costs of symbiont‐conferred resistance to parasitoids in aphids. Ecology and Evolution, 3(3), 706–713. https://doi.org/10.1002/ece3.491
Wensvoort, J., Kyle, D., Ørskov, E., & Bourke, D. (2001, March). Biochemical adaptation of camelids during periods where feed is withheld. Rangifer, 21(1), 45. https://doi.org/10.7557/2.21.1.1527
Yamada, T., Katagiri, H., Ishigaki, Y., Ogihara, T., Imai, J., Uno, K., Hasegawa, Y., Gao, J., Ishihara, H., Niihima, A., Mano, H., Aburatani, H., Asano, T. & Oka, Y. (2006). Signals from intra-abdominal fat modulate insulin and leptin sensitivity through different mechanisms: Neuronal involvement in food-intake regulation. Cell Metabolism, 3(3), 223-229. https://doi.org/10.1016/j.cmet.2006.02.001
9.4: Oxidation of Fatty Acids – Chemistry LibreTexts. (2020). Chemistry LibreTexts. https://chem.libretexts.org/Courses/Brevard_College/CHE_301_Biochemistry/09%3A_Metabolism_of_Lipids/9.04%3A_Oxidation_of_Fatty_Acids