The Physical Properties of Fats in Mammals
Milana Farah, Josh Negenman, Michelle Sayeh, Edward Tong
Abstract
Fats play many important roles in the bodies of mammals. With various physical and chemical forms, fats can serve different purposes in different animals. In hibernators, different types of adipose tissues—white and brown adipose tissues—serve separate roles. White adipose tissues serve as general purpose energy storage, while brown adipose tissues are specialized in producing heat. In animals living in the Arctic, fats serve another unique purpose. In these cold environments, the threat of hypothermia forces these animals to develop a thick layer of subcutaneous fat called blubber. With its characteristic poor thermal conductivity, blubber serves as an effective insulator against the cold. Beyond thermoregulation, fats serve as mechanical support and protection for animals. In terrestrial animals, fats in the form of subcutaneous foot pads distribute the animal's weight evenly across its feet and absorb and cushion the shock of impacts, preventing damage to foot and leg structures.
Introduction
Fat, also known as adipose tissue, is a connective tissue composed primarily of adipocyte fat cells. Adipocytes are energy-storing cells that house large fat globules called lipid droplets encircled by a structural fiber network (Hernández, n.d.).
Based on their origin, location, and function, adipocytes are divided into three distinct categories: white, brown, and beige. These cell types can be classified into two functionally different tissues: white and brown. The brown adipose tissue (BAT) contains highly metabolically active, heat-producing brown adipocytes. On the other hand, white adipose tissue (WAT) is composed of white and beige adipocytes. White adipocytes are the most abundant adipocytes in mammals and are used for energy storage in the form of fatty molecules (primarily triglycerides) and cushion vital organs. Moreover, beige adipocytes, scattered amongst pockets of white adipocytes, can produce heat in certain circumstances (Fenzl & Kiefer, 2014).
Adipose tissue can be found in various body parts in different forms: WAT is found in subcutaneous fat, visceral fat, and bone marrow fat, which surrounds organs and cushions other body parts such as the soles of the feet, eyeballs, and certain blood vessels. As opposed to white adipose tissue, BAT is primarily found in the body core: on the back, along the vertebrae, and around vital organs. (Hernández, n.d.).
In the following sections, a physical analysis of fat will be done to explain its functions in various phenomena, including the fat's thermal and energetic properties during mammalian hibernation, the role of blubber as a thermal insulator, and its biomechanical functions in the foot pads of mammals.
The importance of adipose tissue in hibernation
When food sources are dwindling and water reserves are limited, some animals in the wild adopt an extreme survival tactic: hibernation. While the type of hibernation varies between animals, the same concept applies to all: it is a form of hypothermic dormancy characterized by significant physiological changes such as reduced metabolic activity, decreased body temperature, a slower heartbeat, a decline in breathing rate, and a minimized brain activity. This state of inactivity is only achievable thanks to fat reserves acquired in the months prior to hibernation, which serve as a source of energy and insulation.
However, not all animals hibernate. Cold-blooded animals, also known as ectotherms, cannot produce significant heat, making them unable to hibernate. Thus, they rely on external heat sources such as sunlight or heated rock surfaces to regulate their body temperature. On the other hand, warm-blooded animals, also known as endotherms, can adjust their metabolic heat production and insulation to maintain a steady body temperature, allowing them to enter states of hibernation (Grigg et al., 2016). The duration of this state varies depending on the species of hibernator. Some go into the torpor state (a state where all physiological changes occur) for many months, while others, such as ground squirrels, oscillate between periods of torpor and interbout arousal (short period of reactivation of metabolism) (Figure 1) (Hampton, Melvin, & Andrews, 2013).
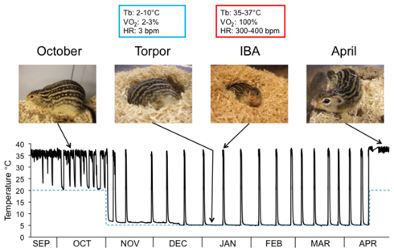
Fig. 1 Graph representing the different states of ground squirrels during pre-hibernation and hibernation (Hampton et al., 2013).
All this is possible thanks to both BAT and WAT. As previously mentioned, BAT generates body heat due to its oxidation by cell mitochondria. It also stimulates the organs by sending a burst of energy to activate the interbout arousal and terminate the torpor state (Webb & Schnabel, 1983). Meanwhile, WAT stores energy in the form of lipid molecules. As showcased in Figure 2, these types of tissues are found in different parts of the organism depending on their functions.
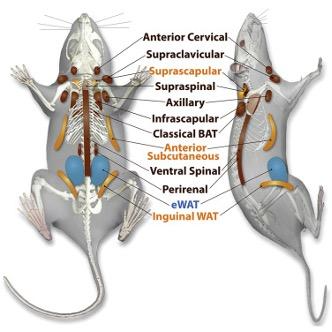
Fig. 2 Location of different types of adipose tissue in a rat. WAT is in blue, beige adipose tissue in yellow and BAT in brown (Zhang et al., 2018).
Altering fat levels
During pre-hibernation periods, mammalian hibernators store fat in white adipose tissue located under the skin, around internal organs, and in central cavities of bones. Different animals utilize various methods to alter fat levels, such as hyperphagia, decreased motor activity, decreased body temperature, and other mechanisms, such as varied feeding efficiency (Mrosovsky, 1976). For instance, the fat-tailed dwarf lemur, Cheirogaleus medius (Figure 3), inhabits the woodlands of Madagascar, where the weather is extremely arid for several months. The lemurs, male or female, gain about 87.8 ± 25.4 % of their former body weight during April, which is their pre-hibernation period. They store the additional mass mainly in their tail, which reaches up to 40% of their total body weight (Figure 4) (Fietz & Ganzhorn, 1999).
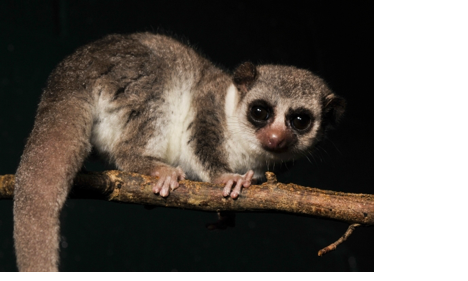
Fig. 3 A fat-tailed dwarf lemur during the pre-hibernation period (Fat-tailed Dwarf Lemur – Duke Lemur Center, 2021).
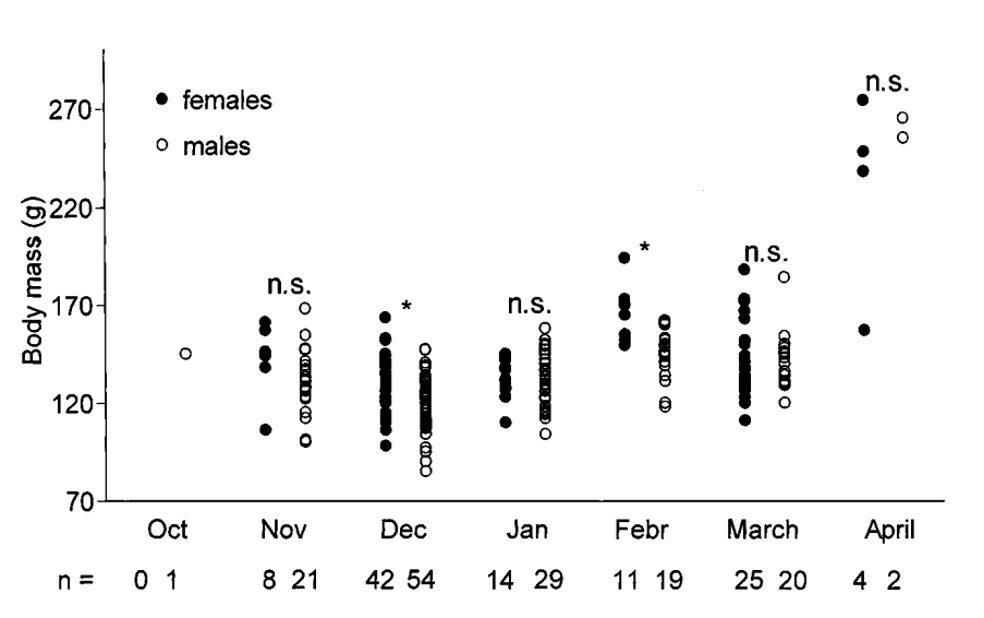
Fig. 4 Variations in body mass of adult male and female Cheirogaleus medius in the Kirindy Forest (Fietz & Ganzhorn, 1999).
The same trend is present in golden-mantled ground squirrels, a hibernating species of squirrels that alter meal patterns and increase meal sizes during pre-hibernation periods. Elevated plasma insulin levels also contribute to increased pre-hibernation obesity (Mrosovsky, 1976).

Fig. 5 Variations of body weights of two representative golden-mantled ground squirrels kept at constant temperatures, 3°C and 12°C. Hibernation seasons are represented by horizontal bars (Adapted from Pengelley & Asmundson, 1969).
Counteracting the loss of fat during hibernation
During hibernation, some mammals adopt a curled position to reduce the surface area available for heat exchanges. In the case of marmots, they can reduce the number of nesting materials required for insulating themselves from the floor in the burrow. The shape of the curled animal resembles a torus, with the innermost portion representing the fur as well as the ventral layer of WAT next to it (Figure 6) (Webb & Schnabel, 1983). The shape the curled marmot adopts can be explained by the Stefan Boltzmann Law of radiation (Bakken, 1976):


Fig. 6 Configurations of the torus shaped curled marmot. (a) Grid of nodal elements, each square represents 1 cm2. (b) Internal distributions of components. (c) Component distributions of external fat. The heavy black line denotes the plane of symmetry. = muscles;
= fat;
= nest material;
= fur (Webb & Schnabel, 1983).
Heat transfer within the hibernator
An animal in the wild is subjected to heat transfer by conduction, convection, and thermal radiation from its environment. The individual heat transfer processes are of the form:
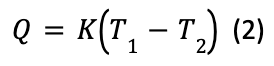
Q denotes heat flow, T1 the animal temperature, T2 the environmental temperature, and K is the lumped thermal conductance (Bakken, 1976).
The energy transfer process during hibernation can be compared with that of a heat engine. As has been previously stated, stored fats are used to fuel and regulate the body, acting similarly to a hot reservoir of energy used during dormant states of hibernation.

Fig. 7 Principle of energy transfers during hibernation compared to the functioning of a heat engine. Qh = heat transfer from stored fats and hormones to the hibernating animal, Qc = heat transfer from the hibernating animal to its environment, and W = Energy required to maintain bodily functions (Karwowski, 2017).
The energy required to maintain bodily functions (W) varies significantly according to the animal's physiological state. For instance, the energy requirements of a lactating mammal are significantly greater than that of a nonlactating mammal. Pengelly and Asmudon (1975) hypothesize that due to the significant body fat decreases caused by lactation, lactating female golden-mantled ground squirrels commence hibernation later in the fall than non-lactating females. This is because they do not reach an adequate weight for hibernation until later in the year (Young, 1976).
Significance of fat in animals without fur
Fur and feathers have a conductivity generally five to eight times weaker than that of fat and therefore are a significantly better source of insulation. However, some animals who do not have fur or feathers must rely exclusively on their fat layer for insulation. The ability of an animal without fur or feathers to maintain a given temperature differential in terms of fat, metabolic rate, and moisture loss by breathing and sweating is expressed by the total temperature difference between Tb, the internal body temperature and Ts, the external radiating surface temperature:
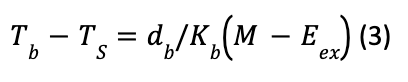
With M the metabolism, Eex the respiratory moisture loss, db the thickness of fat, and Kb the conductivity of fat (Porter & Gates, 1969).
Brown adipose tissue in black bears
As previously mentioned, these animals do not consume food over the hibernation season but use WAT as their primary energy source. On the other hand, BAT is crucial for heat formation. In fact, BAT is considered a specialized thermogenic tissue that converts chemical energy into heat. The latter is generated very rapidly, uniformly, and continuously within this layer of fat, which is present in many endotherms to maintain a high body temperature during the hibernation cycle. This phenomenon is known as non-shivering thermogenesis (Fenzl & Kiefer, 2014). This heat generation occurs multiple times during the hibernation season, when BAT utilizes stored lipids at high rates, prompting hypothermic torpor bouts to be interrupted. These interruptions, also known as interbout arousals, are frequent and fast-warming episodes that occur every few weeks during hibernation (Hampton et al., 2013). They are essential for getting rid of built-up metabolic waste, activating a dormant immune system, and combating pathogens introduced directly before or during hibernation (Prendergast et al., 2002).
A Cornell study on black bears, or Ursus americanus, highlights the role of brown adipose tissue during extreme external conditions (Cottrell et al., 2003). The black bear has four main layers: an outer fur layer, skin and fat layers, and an inner lean body mass core, all contributing to preserving its body heat.
During a hibernation period of approximately three and a half months, the temperatures in the bear's fur layer decrease to below freezing and drop to as low as 12.5 °C in the fat layer. However, the body core's minimum temperature is 26°C, with the temperature greater at the center, certifying that black bears can generate heat within their brown fat layer to survive (Figure 8) (Cottrell et al., 2003).
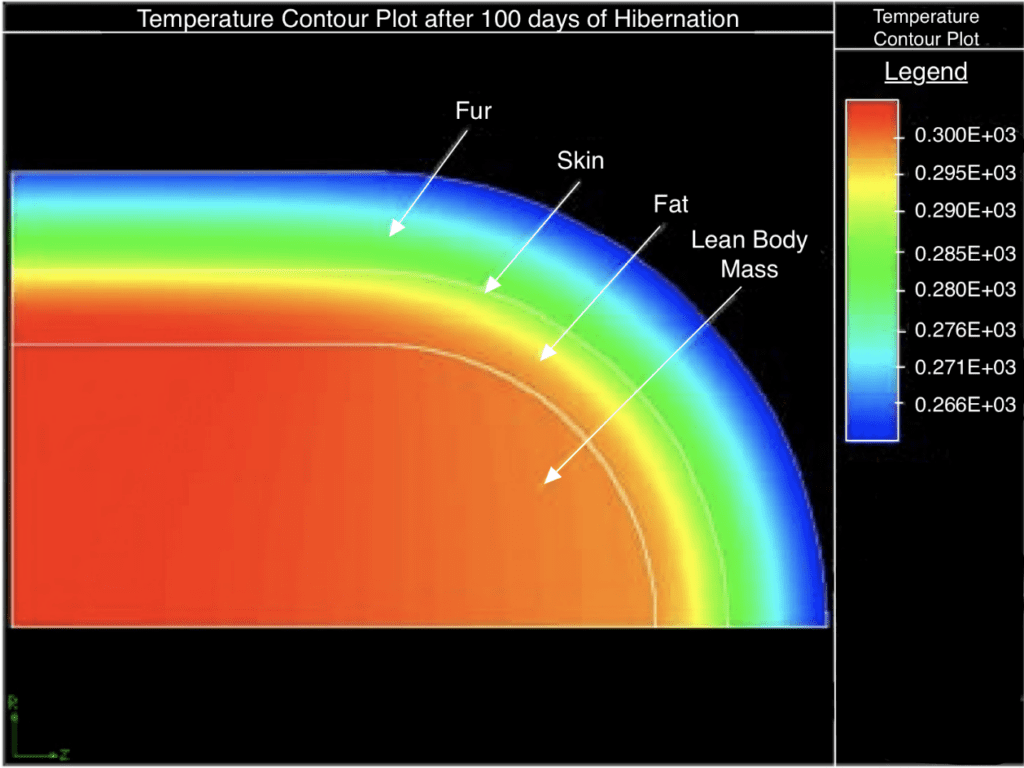
Fig. 8 Temperature plot of the body layers of a black bear after roughly 100 days (about three and a half months) of hibernation (Adapted from Cottrell et al., 2003).
Furthermore, according to the Cornell experiment, the hibernating bear will have a core body temperature of roughly 30° C with a volumetric heat generation term of 1050.369 W/m3. These values are deducted by computing a basal metabolic rate (BMR) based on Kleiber's Law, assuming a 40% efficiency of energy conversion in the BMR (Cottrell et al., 2003). Kleiber's Law (Niklas & Kutschera, 2015) states that an organism's BMR, which is the lowest number of calories an animal must burn to maintain their basic life-sustaining functions (Fletcher & Sampson, 2020), is approximately equal to its mass M to the power of three-quarters (with β as a normalization constant):
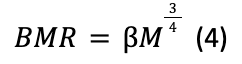
Hence, the black bear can survive the winter since it has suitable energy stores to maintain a high body core temperature and BMR.
Interdependence of white and brown adipose tissue
Even though BAT and WAT play distinct roles in an organism, they work together to ensure the mammal's survival during hibernation. While the brown fat layer initiates heat production, white fat can be a source of fuel for the body and an insulator. By altering thermal properties such as the fat conductivity in a black bear, significant changes in the temperature of fat tissue as well as the body mass core were recorded by Cottrell et al. (2003). As shown in Figure 9, the center's temperature decreased by 7.5°C with only a rise of 0.3 W/(m*K) in fat conductivity. Therefore, during hibernation season, the white fat tissue acts as an insulator preserving the heat generated by the brown fat in the core.
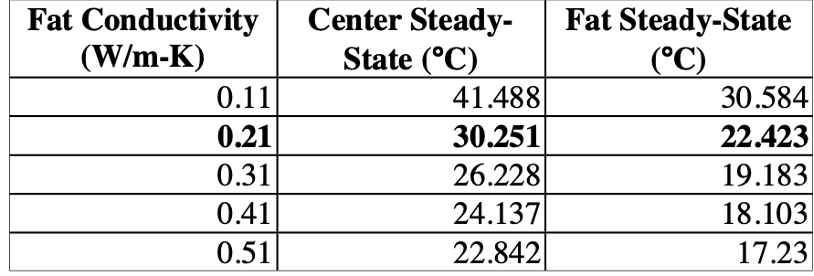
Fig. 9 Table representing the effect of fat conductivity on the body's temperature (Cottrell et al., 2003).
In summary, hibernation is the natural solution to environmental challenges for certain mammals that are physically unable to withstand harsh weather conditions. However, like all solutions, it has its limitations. In this state of inactivity, the hibernators are incapable of providing for themselves. The overconsumption of food before hibernation resolves this issue as it contributes to their white fat supply providing the energy to maintain bodily functions. Nonetheless, mammals cannot stay in this state of dormancy during the whole hibernation period due to their need to defecate and stimulate their immune system. These physiological needs are satisfied by the interbout arousal induced by brown fat.
The functions of blubber in arctic mammals
Beyond hibernators, many other animals use fats to keep themselves warm in freezing environments such as the polar regions (Arctic and Antarctic) and the cold waters deep in the ocean. This is especially true for warm-blooded mammals. These mammals have body temperatures ranging from 37oC (99°F) to 40oC (104°F) (Britannica, 2018), and to function properly, they need to maintain their body temperatures. Even a decrease of a few degrees in an animal's core temperature can lead to hypothermia, at which the animal's organs will slowly stop functioning, eventually leading to the animal's death (CDC, 2019).
In the harsh climate of the Arctic and Antarctic regions, temperatures are often around negative 40oC to negative 50oC, but can reach as low as negative 65oC, and the waters of those polar regions are constantly around their freezing point. Arctic and Antarctic animals such as polar bears must live in this harsh environment where the temperature gradient between the environment and the animal's core temperature can reach 100oC (Blix, 2016). Additionally, for marine animals such as seals who live in water, heat is lost to the surrounding water much faster than on land, as water is 25 times more conductive than air. As a result, the skin of these marine animals is usually only a few degrees above freezing (Castellini, 2018). This means that maintaining the animal's core temperature becomes difficult. Animals who live in these climates have two options: either produce more heat, or mitigate the heat lost to their surroundings. The first choice is much harder to achieve as it would require the animal to increase its metabolism and force it to consume more food in an environment where food sources are already scarce. The second option is, therefore, the only feasible one.
Insulation through blubber
The second law of thermodynamics shows that heat will naturally diffuse from a warmer region to a colder region. The rate at which heat will be diffused from the warmer region to the colder one is determined by the thermal conductivity of the medium. The general equation of heat transfer is the following:
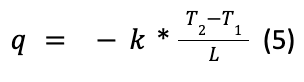
Where q is the heat flux which represents the rate at which heat is transferred, k is the thermal conductivity of the medium with units of watts per meter kelvin (W/(m*K)), T2 and T1 are the temperatures of the warmer and colder regions, respectively, and collectively represent the temperature gradient, and L is the distance that separates both regions (Britannica, 2018).
To decrease the heat flux, animals such as seals and polar bears have developed a thick layer of fat, called blubber, to properly isolate themselves from the cold. This layer of blubber increases the separation distance between the two environments of the freezing air and the core body. The thickness of these layers varies between animals, from a few millimeters in newborn pinniped seal pups to over 50 centimeters in large whales (Castellini, 2018). Blubber also creates a poorly conducting medium between the body core and the air, as the thermal conductivity of blubber is around 0.08 W/(m*K) (Hatfield & Pugh, 1951). For comparison, asbestos, a mineral insulator used in the past, has a similar thermal conductivity of around 0.08 W/(m*K), and water at 20oC has a thermal conductivity of 0.2 W/(m*K) (Nave, 2017). Also, the thicker layers of blubber are often present in large whales with lower metabolism, meaning they produce less heat (Parry, 1949).

Fig. 10 Cross section of a harp seal. b: blubber, s: spine, k: kidneys, i: intestines (scale bar = 5 cm) (Blix, 2016).
Blubber in whales
In whales, blubber comprises three tissue layers: the epidermis, the dermis, and the hypodermis, with the latter two containing most of the blood vessels. The epidermis is the superficial level of blubber which is the closest level to the skin, with its white or dark gray pigmentation, determining the color of the animal. The lower section of the epidermis layer consists of more flattened cells with thicker cell walls and lower cytoplasm volume. The dermis is the middle layer between the epidermis and the hypodermis and is the thinnest layer out of the three. It is mainly composed of connective tissue fibers, which get denser towards the epidermis and can even protrude into its lower layer (Figure 11). The number of fat cells increases as it approaches the hypodermis, which makes it less dense near the hypodermis. Finally, the hypodermis is the bottom layer closest to the core body. It is the thickest layer consisting of almost solely fat cells and makes up most of the whale's blubber (Parry, 1949).
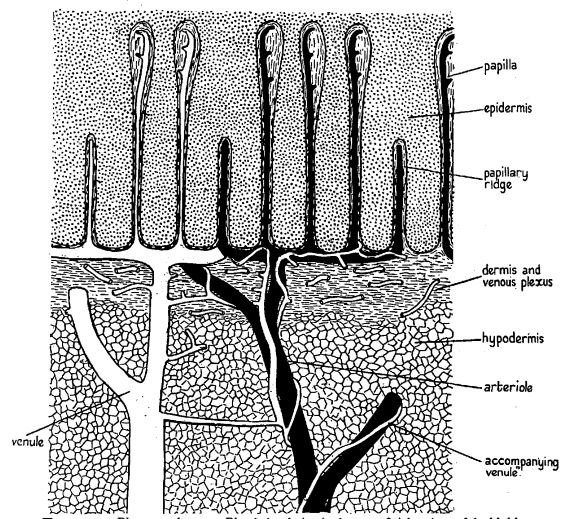
Fig. 11 Drawing of a cross-section of whale blubber (Parry, 1949).
Fats are one of the most insulating molecules in an animal's body. Muscles, for example, are about twice as thermally conductive as fats (Hatfield & Pugh, 1951) (see Figure 12). Also, thermal conductivity has been found to be inversely proportional to a product's fat content, meaning that the more fat a product contains, the less thermally conductive it is (Tavman & Tavman, 1999). However, fur is often a better insulator than fats for an equal thickness with its thermal conductivity of 0.035 W/(m*K) due to its ability to trap air, which is one of the best insulators at 0.02 W/(m*K) (Cena & Clark, 1978). That said, an advantage of blubber over fur for marine animals is that, under the high pressures of water, fur loses its ability to trap air as the pressure pushes the air out, decreasing the thermal conductivity of the fur to around 0.16 W/(m*K). Blubber, on the other hand, does not lose its insulating properties under those pressures (Frisch et al., 1974).

Fig. 12 Table comparing the thermal conductivity (in cal*cm/(sec*cm²*°C)) of fats and muscles (Hatfield & Pugh, 1951).
Unlike inert layers of fats, blubber consists of a loose protein matrix of collagen fibers forming a sponge-like structure in which lipids are free to move in and out. Additionally, blubber is highly vascularized. These many blood vessels within the blubber are crucial for controlling heat loss. In cold environments, these blood vessels within the blubber would contract to minimize heat loss; thus, the only heat lost would be through the poorly conducting blubber. However, as the whale travels through hotter regions, these blood vessels must dilate and allow heat to escape more easily to prevent the whale from overheating. Thus, blubber is not only used for insulation but also serves as a tool to regulate the whale's temperature (Parry, 1949).
Physics behind blubber
To understand why blubber makes such a great insulator, one needs to understand how heat is transferred. Heat can be transferred through three processes: conduction, convection, and radiation. Conduction occurs when molecules transfer their thermal energy through collision with other molecules. One can visualize it with two neighboring molecules: the vibration of one molecule will force the other to vibrate at the same speed and thus transfers thermal energy. Convection occurs when the medium constantly gets replaced. For example, when a body transfers heat into its surrounding air, the hot air will rise, and cold air will come to replace it, thus creating a cycle that transfers heat away from the body. Radiation is caused by the transfer of energy through electrons and photons. Thus, many factors influence a material's thermal conductivity, such as materials phases (i.e., solid, liquid or gaseous), and structure and electrical conductivity (Britannica, 2019).
Blubber is a non-conducting solid, meaning its primary method of heat transfer is conduction. While radiation can occur, its impact on the fat's thermal conductivity in a non-conducting material at those temperatures is negligible. Also, convection cannot occur in solids as convection currents cannot form. This limiting path to transfer energy allows blubber to be such a poor thermal conductor (Connor, 2019).
Beyond insulation
Beyond insulation and energy storage, fats are used by marine animals for different purposes. For example, in seals, blubber is used as a flotation device. As fat is less dense than water, a seal's blubber layer allows it to be buoyant and float on water (Nature, 2022). These blubber layers allow the seal to rest while on water, unlike its natural predator, the polar bear who would sink and drown if it stopped swimming. However, this buoyancy factor can limit the amount of blubber in some marine animals as it would hinder their ability to dive in deep waters.

Fig. 13 A seal floating showcasing the buoyancy of its blubber (Mel, 2016).
Blubber can also serve structural and locomotive roles, such as the blubber in the dorsal fin of marine animals, which allows them to keep a hydrodynamic shape (Iverson & Koopman, 2018).
To summarize, the freezing conditions of the Arctic and Antarctic regions are inhospitable for many mammals, and the threat of hypothermia is constant. Blubber allows animals to create an insulated environment, isolated from the harsh external conditions, inside their body, thus keeping their normal body temperature.
The biomechanics of fat in mammal foot pads
In addition to being an efficient form of energy storage and insulation, fats possess other physical properties that make them an extremely useful building block for biological systems. Fats are used in the absorption of mechanical energy, can have spring-like properties, store elastic energy, and store and absorb strain energy, making them incredibly diverse and useful in physical biology outside of thermodynamics (Alexander et al., 1986; Chi & Roth, 2010). An ideal example of this is in the fatty foot pads found on the undersides of the feet of mammals. The geometry, composition, and size of these foot pads vary based on mass, size, species, and environment. However, animals from elephants to carnivorous animals, to hooved animals all possess fatty foot pads that help distribute load, absorb impact, and protect the foot and leg structures from damage (Chi & Roth, 2010; Räber et al., 2004; Weissengruber et al., 2006). Though the macroscopic mechanical properties of fats in biological systems are not a topic of extensive research, studies examine the physical composition of foot pads, their physical properties, and the mechanical functions they perform in animals.
The physical composition of foot pads
The African elephant is the largest terrestrial creature on earth, with individuals reaching masses of up to 8 000 kg (Panagiotopoulou et al., 2016). To help support this weight, they have evolved to have thick fatty foot pads as an important structural component of their foot anatomy. This fat makes up a substantial proportion of the foot's volume, and this cushion and other fat structures are interwoven with the foot digits' bones, ligaments, and other anatomical components of the foot.
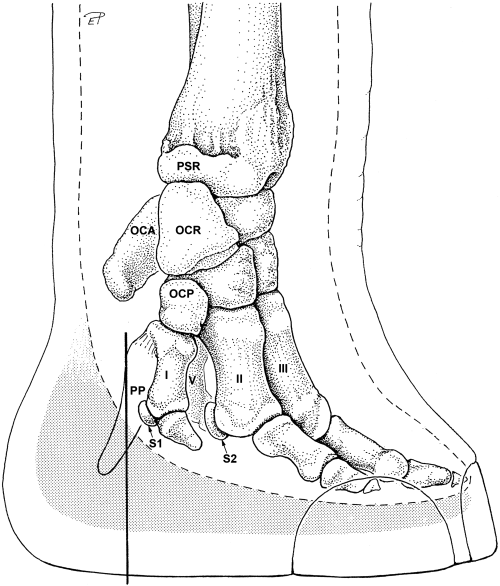
Fig. 14 Diagram of the hindlimb foot structure of an African elephant. The dotted line represents the border between soft tissues and the inner structures of the limb; the gray shaded area represents the fatty cushion found at the base of the foot structure (Weissengruber et al., 2006).
The composition of the foot pad itself varies depending on species, animal size, and other factors. For the African elephant, the foot pad cushions are composed of a network of white or yellowish adipose tissue interwoven with a network of elastic strands. These fatty structures are found between the bones, tendons, muscles, and ligaments in the elephants' feet. The foot pads can be categorized into metacarpal/metatarsal and digital compartments based on their locations in the foot and their appearances (Weissengruber et al., 2006). Other studies support the assertion that foot pads contain similar physical properties across different species. Räber et al. (2004) and Räber et al. (2006) discuss how cattle have a foot pad structure containing three distinct parallel pads; axial, middle, and abaxial, that run from the heel of the cows' feet to underneath the distal phalanx of the foot. They maintain that the cushions themselves are complex structures composed of fat and connective tissue. Carnivores have foot pads that are also composed of fat lobules with connective tissue strands, and even horses, who have suspensory apparatuses in their hooves that support most of their weight, have fatty cushions underneath their feet, though their chemical composition is somewhat different from the other mammals mentioned above (Weissengruber et al., 2006).
At the microscopic level, African elephant foot pads are composed of subcutaneous adipose tissue lobules surrounded by coarse bundles of collagen fibers. This system creates a supportive network of tissue that can withstand the extreme forces that come from supporting the weight of an African elephant (Weissengruber et al., 2006). Figure 15 shows a microscopic view of the elephants' fat pad composition.
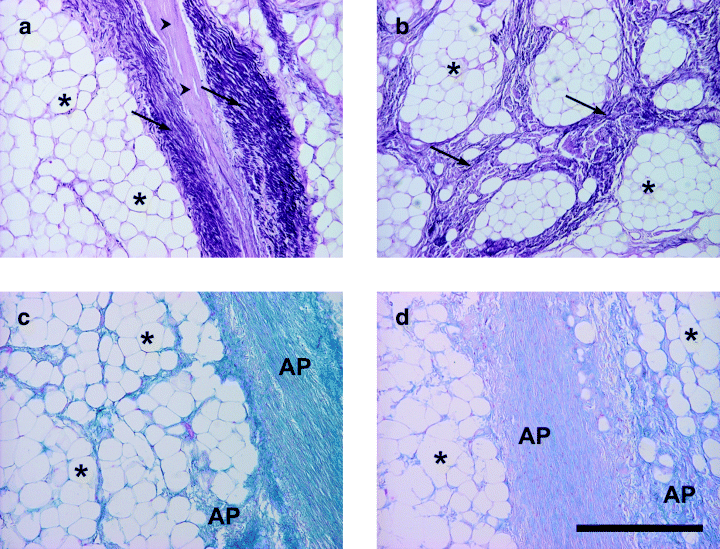
Fig. 15 Images of the tissue composition of the footpads of African elephants. Asterisks show adipocytes, arrowheads show collagen fibers (scale bar = 500 µm) (Weissengruber et al., 2006).
The chemical composition of these cushions will not be explored in detail. It is worth understanding the basic composition of these structures prior to investigating their physical and mechanical properties, as understanding the structure can help to explain many of the properties displayed by these fat pads.
Scaling of foot pads with animal size
Foot pads play a variety of roles in mammals' feet. They absorb mechanical shock, distribute loads across the foot to reduce localized pressure and stress, and store and return elastic strain energy (Chi & Roth, 2010; Weissengruber et al., 2006). All these properties are essential for the function of the foot and the limb. One interesting way to investigate the properties and function of these foot pads is to study their functions, size, and composition with respect to the size of the animal.
Foot pads vary in size, shape, and composition in all animals, but they become increasingly more important in larger and faster animals. This is due to higher stresses being placed on the limbs and feet from increased forces. In fact, stresses increase with mass (M) at a factor of M0.33 due to higher locomotor forces. Mass increases with volume while foot pads are limited to scaling with area (Clemente et al., 2020). Animals such as elephants, rhinoceroses, and camels are ideal subjects for study, as their fat pads are well developed and functionally active due to the necessity of supporting large weights. Chi & Roth (2010) found that foot contact area does not increase linearly with increased animal body mass and proposed four hypothesized models for how the properties of the foot pads would increase with increasing body mass. Their scaling relationships took the form:

Where y is the variable of interest, M is the mass of the animal, and a and b are parameters determined through experiment. They found that the model that best fit their data was a scaled stiffness model, where the compressive stiffness (ky) scales with the mass of the animal:
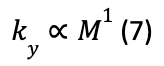
Findings also indicated that there was a reduced strain in the pads for larger animals, but there was some deviation from the model above that indicates a potential compromise between scaled stiffness (model above) and constant strain (model below) in the foot pads.
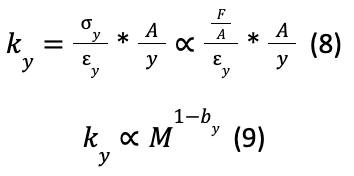
Where ky is compressive stiffness, y is the constant strain (yM0), A is the area of the foot pad, F is the force applied, by is an empirically deduced constant, and y is the variable of interest.
These results indicate that the material properties of footpads vary along with size and shape to best suit the needs of the specific species; specifically, the strain energy density and compressive modulus of the material is not constant across species. Further, the material properties within a species may vary with the age of the animal. In cattle, the older and heavier the animal, the higher the proportion of connective tissue, and thus the stiffer the foot pad (Räber et al., 2004). It is uncertain if this same trend is also present in elephants (Weissengruber et al., 2006). As far as the significance of this with respect to the mechanical performance of the foot pads, this means that different animals' pads will be able to accommodate higher pressures than others, distribute forces differently, and will have different elastic properties to suit their specific needs (Chi & Roth, 2010; Clemente et al., 2020).
The elastic properties of foot pads
Part of the function of the fat pads found on the feet of animals is to absorb impact, which is accomplished by temporary deformation of the structures. This gives rise to another mechanical property of foot pads: elasticity and strain energy storage. Foot pads can store this mechanical energy when compressed and release it as the foot leaves the ground, which could impact walking and propulsion in some animals (Chi & Roth, 2010; Weissengruber et al., 2006).
It is well documented that quadrupeds generally place more load on their front legs. African elephants, for example, carry about 60% of their mass on their front legs, and this front-heavy loading trend is also present in a variety of animals, including camels, alpacas, and carnivores (Chi & Roth, 2010; Clemente et al., 2020; Panagiotopoulou et al., 2016). As the body mass of an animal increases, the strain energy stored in the hind foot pads increases at a slower rate than the strain energy stored in the front foot pads. This preserves more of the elastic properties of the foot pads, and when combined with the lower loading of the hind legs, the foot pads act as a biological spring to help propel the animal forward during locomotion (Chi & Roth, 2010).
The nature of this spring action is not linear. If foot pads behaved according to a linear spring model, a running animal would set the pad into oscillation. If this occurs, further contact between the pad and the ground results in chattering, where the foot would bounce up and down on the ground throughout one step, resulting in poor grip and foot performance (Alexander et al., 1986). Instead of acting in a linear manner, compression is very low when there is low pressure on the pad and ramps up as more pressure is added and the pad is compressed further. This ramping up phenomenon makes sense, as the foot pad can never be squeezed to a thickness of zero, and hence the stiffness of the material must increase to counteract increasing pressure. By testing the foot pads of certain mammals between an actuator and a load cell, Alexander et al. (1986) were able to plot the force applied against the displacement of the foot pad, giving good graphical data of the stiffness curve of the tested foot pads.

Fig. 16 The curves of force vs. pad displacement. The ForceWeight axis is the force divided by the weight of the respective animal, to give a measurement in multiples of the animal's mass. (a) Is the data from a wallaby metacarpal pad; (b) is the data from a foxhound metacarpal pad; (c) is a camel metatarsal pad; and (d) shows the data compared to the model made by the researchers. The test was conducted for multiple oscillations, and each graph shows the data from one oscillation at frequencies 1.1 Hz, 11Hz, 0.11 Hz, and 1.1 Hz (Alexander et al., 1986).
These curves show a trend where the force may be proportional to the square of the deflection or could be represented by the sum of two terms, one proportional to the foot pad's deflection and one proportional to the 10th power of the foot pad's deflection (Alexander et al., 1986). This nonlinear action is explained by the necessities of the fat pads as functional tools. They must absorb some impact, but cannot fully compress, as they must also offer support and effectively distribute pressure across the foot, and so cannot simply absorb shock with linear support, as this would not effectively perform these functions. Instead, foot pads display viscoelastic properties, meaning they behave somewhat as elastic devices, but also portray viscous properties (Alexander et al., 1986). These properties are a unique application of fats as biomechanical devices and display interesting and functional properties to fulfill their role in mammals.
Compression and pressure distribution of foot pads
Another function of the fatty foot pads in mammals is the distribution of pressure across the base of the foot. Localized pressures in feet can cause inflammation, enthesopathy, cracks in calluses, and defects in callus growth in camels, and causes similar injuries in other animals (Clemente et al., 2020; Panagiotopoulou et al., 2016). To avoid such injuries, animals have evolved fat pads specific to their needs.
In large mammals, pressures through the foot pads can be extremely high. In cows, average pressures in the hind foot pads can range from 50 to 80 N/cm2, and median pressures under the lateral hind claws have been measured at 28.2 N/cm2 (Räber et al., 2006). In white rhinoceroses, Panagiotopoulou et al. (2019) measured mean peak pressure values at 22 N/cm2 and 18 N/cm2 for two individual adults. Studies have recorded that African and Asian elephants exert mean peak pressures of 94.6 N/cm2 and 56.7 N/cm2 (equivalent to 946 kPa and 567 kPa), respectively (Panagiotopoulou et al., 2016; Panagiotopoulou et al., 2019). These pressures can cause considerable damage to tissues if not distributed evenly across the foot pad.
Due to their large weights and large foot diameters, elephants and rhinoceroses are good subjects for analyzing pressure distribution in foot pads. Figure 17 shows the mean pressure distributions on each foot of five African elephants.

Fig. 17 The mean pressure distribution patterns of five African elephants participating in a study. The images are smoothed over to interpolate between pressure points on the sensory grid (Panagiotopoulou et al., 2016).
The fat pads effectively distribute pressures across the entire foot and minimize pressure points underneath the feet. The highest concentrations in peak pressures are typically found in the lateral parts of the feet, a trend also found in other animals (Panagiotopoulou et al., 2016; Räber et al., 2006). A further topic of interest regarding pressure is the center of pressure (COP) trajectory throughout the stride of the African elephants studied.
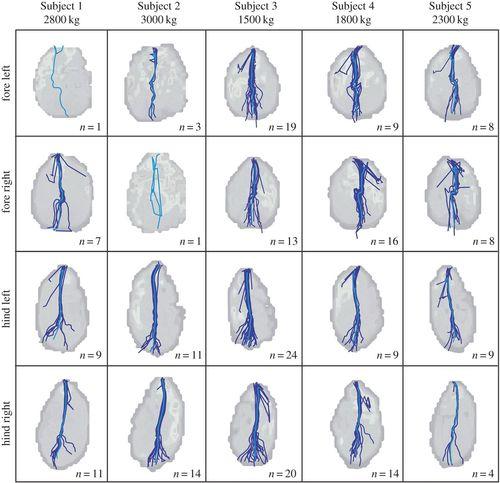
Fig. 18 The center of pressure (COP) trajectories throughout the stance phase of five African elephants. Individual trials are depicted by dark blue lines and the mean of all the trials is depicted by a light blue line (Panagiotopoulou et al., 2016).
The general COP trajectory goes from the rear of the foot to the front, though each foot has slight deviations from a true straight trajectory. Throughout the stride, the elephants' lipid-based foot pads evenly distribute pressure across the foot, while also maintaining the structural integrity needed to follow through with the stride and maintain a relatively straight COP trajectory.
The elephant stride is a prime example of the functionality of fats as a biomechanical device. Their fat lobule and protein strand structure enable them to act both as a viscous damping device and as a nonlinear spring (Alexander et al., 1986; Weissengruber et al., 2006). These properties allow the pads to effectively distribute pressure and maintain structural integrity to support the weight of animals as large as the African elephant (Panagiotopoulou et al., 2016). This evolutionarily developed mechanical system is an excellent illustration of the mechanical properties that fats can have in biological systems.
Locomotion in terrestrial mammals puts a huge amount of pressure and stress on animals' limbs, particularly for large animals. These stresses can cause serious damage to animals' feet, legs, and tissues if not properly managed. To solve this problem, mammals have evolved specialized fatty foot pads, whose compositions and structures vary depending on the animal's needs, to distribute forces across the bases of their feet. These foot pads provide the support and physical characteristics necessary for animals to move with extreme speed and support extreme weight while preventing injury to the other structures of the animals' bodies.
Conclusion
The fat organ, located throughout the bodies of countless organisms, contributes to many different physiological needs. Endotherms rely on adipose tissue to endure harsh conditions during hibernation, with WAT gained during pre-hibernation serving as energy storage for the hibernators. Additionally, BAT generates heat and induces interbout arousal to satisfy the mammals' defecation needs and immunity reactivation. These heat transfer properties depend on the type of mammals (e.g., fur or no fur), their size, and their circumstances (e.g., lactating or not). In aquatic mammals, the fat tissue known as blubber is a vital organ characterized by its insulating properties. It contains complex layers of fats alongside blood vessels that contract to minimize heat loss. Blubber is also a floatation device for aquatic mammals, allowing creatures such as seals to rest while on water. Finally, land mammals' foot pads contain fats that have viscoelastic properties whose specific parameters vary depending on the needs of the animal. They act as a spring and damping mechanism, storing, and releasing strain energy to aid in locomotion, and distribute pressures across feet to reduce localized stresses and prevent injury. Overall, fats are an extremely versatile and useful tool and fulfill a wide variety of functions in animals, making them an integral component to evolution and mammalian life.
References
Alexander, R. McN., Bennet, M. B., & Ker, R. F. (1986). Mechanical properties and function of the paw pads of some mammals. Journal of Zoology 209(3), 405-419. https://doi.org/10.1111/j.1469-7998.1986.tb03601.x
Bakken, G. S. (1976). A heat transfer analysis of animals: Unifying concepts and the application of metabolism chamber data to field ecology. Journal of Theoretical Biology, 60(2), 337–384. https://doi.org/10.1016/0022-5193(76)90063-1
Ballinger, M. A., & Andrews, M. T. (2018). Nature's fat-burning machine: brown adipose tissue in a hibernating mammal. The Journal of experimental biology, 221(Pt Suppl 1), jeb162586. https://doi.org/10.1242/jeb.162586
Birkebak, R. C., Birkebak, R. C., & Warner, D. W. (1964). A Note on Total Emittance of Animal Integuments. Journal of Heat Transfer, 86(2), 287–288. https://doi.org/10.1115/1.3687122
Blix, A. S. (2016). Adaptations to polar life in mammals and birds. Journal of Experimental Biology, 219(8), 1093–1105. https://doi.org/10.1242/jeb.120477
Britannica, T. Editors of Encyclopedia (2018, October 17). warm-bloodedness. Encyclopedia Britannica. https://www.britannica.com/science/warm-bloodedness
Britannica, T. Editors of Encyclopaedia (2019, July 4). heat transfer. Encyclopedia Britannica. https://www.britannica.com/science/heat-transfer
Britannica, T. Editors of Encyclopaedia (2018, February 7). Thermal conduction. Encyclopedia Britannica. https://www.britannica.com/science/thermal-conduction
Cena, K., & Clark, J. A. (1978). Thermal insulation of animal coats and human clothing. Physics in Medicine and Biology, 23(4), 565–591. https://doi.org/10.1088/0031-9155/23/4/001
Centers for Disease Control and Prevention. (2019, February 8). Hypothermia|Winter weather. Centers for Disease Control and Prevention. https://www.cdc.gov/disasters/winter/staysafe/hypothermia.html
Chi, K., & Roth, V. L. (2010). Scaling and mechanics of carnivoran footpads reveal the principles of footpad design. Journal of the Royal Society Interface 7(49), 1145-1155. https://doi.org/10.1098/rsif.2009.0556
Clemente, C. J., Dick, T. J. M., Glen, C. L., & Panagiotopoulou, O. (2020). Biomechanical insights into the role of foot pads during locomotion in camelid species. Scientific Reports 10, 3856 (2020). https://doi.org/10.1038/s41598-020-60795-9
Connor, N. (2019, June 4). What is thermal insulation – thermal insulator – definition. Thermal Engineering. Retrieved October 4, 2022, from https://www.thermal-engineering.org/what-is-thermal-insulation-thermal-insulator-definition/
Cottrell, J., Hogan, C., Jain, N., Nogal,B., & McWay, M. (2003), Modeling Heat Flows in a Hibernating Black Bear, Cornell University. https://ecommons.cornell.edu/bitstream/handle/1813/137/Bear.pdf;sequence=2
Evers, J. (2022, May 20). Blubber. National Geographic Society. https://education.nationalgeographic.org/resource/blubber
Fat-tailed Dwarf Lemur – Duke Lemur Center. (2021, May 28). Duke Lemur Center. https://lemur.duke.edu/discover/meet-the-lemurs/fat-tailed-dwarf-lemur/
Fenzl, A., & Kiefer, F. W. (2014). Brown adipose tissue and thermogenesis. Hormone Molecular Biology and Clinical Investigation 19(1), 25-37. https://doi.org/10.1515/hmbci-2014-0022
Fietz, J., & Ganzhorn, J. U. (1999). Feeding ecology of the hibernating primate Cheirogaleus medius : how does it get so fat? Oecologia, 121(2), 157–164. https://doi.org/10.1007/s004420050917
Fletcher, J., & Sampson S. (2020, March 9). What to know about basal metabolic rate. Medical News Today. https://www.medicalnewstoday.com/articles/basal-metabolic-rate
Frisch, J., Øritsland, N. A., & Krog, J. (1974). Insulation of furs in water. Comparative Biochemistry and Physiology Part A: Physiology, 47(2), 403–410. https://doi.org/10.1016/0300-9629(74)90002-4
Grigg, G., Geiser, F., Nicol, S., & Turbill, C. (2016, May 20). Not just sleep: all about hibernation. Australian Academy of Science. https://www.science.org.au/curious/hibernation
Hatfield, H. S., & Pugh, L. G. C. (1951). Thermal conductivity of human fat and muscle. Nature, 168(4282), 918–919. https://doi.org/10.1038/168918a0
Hampton, M., Melvin, R. G., & Andrews, M. T. (2013). Transcriptomic Analysis of Brown Adipose Tissue across the Physiological Extremes of Natural Hibernation. PLOS ONE, 8(12), e85157. https://doi.org/10.1371/journal.pone.0085157
Hernández, A. (n.d.). Adipose Tissue What Is It, Location, Function, and More. Osmosis from Elsevier. https://www.osmosis.org/answers/adipose-tissue
Karwowski, S. K. (2017). The physics of hibernation. University of Alaska-Fairbanks. http://ffden-2.phys.uaf.edu/webproj/212_spring_2017/Sabina_Kryshak-Karwowski/Sabina_Karwowski/Home.html
Mel. (2016, November 3). Playful seals at the Chatham Fish Pier on Cape Cod. Cape Cod Blog. https://www.mycapecodblog.com/?p=2265
Mrosovsky, N. (1976). Lipid Programmes and Life Strategies in Hibernators. American Zoologist, 16(4), 685–697. https://doi.org/10.1093/icb/16.4.685
Niklas, K. J., & Kutschera, U. (2015). Kleiber's Law: How the Fire of Life ignited debate, fueled theory, and neglected plants as model organisms. Plant signaling & behavior, 10(7), e1036216. https://doi.org/10.1080/15592324.2015.1036216
Nave, C. R. (2017). Thermal conductivity. http://hyperphysics.phyastr.gsu.edu/hbase/Tables/thrcn.html
Panagiotopoulou, O., Pataky, T. C., Day, M., Hensman, M. C., Hensman, S., Hutchinson, J. R., & Clemente, C. J. (2016). Foot pressure distributions during walking in African elephants (Loxodonta africana). Royal Society Open Science 3(10), 160203. https://doi.org/10.1098/rsos.160203
Panagiotopoulou, O., Pataky, T. C., & Hutchinson, J. R. (2019). Foot pressure distribution in White Rhinoceroses (Ceratotherium simum) during walking. PeerJ 7, e6881 (2019). https://doi.org/10.7717/peerj.6881
Parry, D. A. (1949). The structure of whale blubber, and a discussion of its thermal properties. Journal of Cell Science, s3-90(9), 13–25. https://doi.org/10.1242/jcs.s3-90.9.13
Pengelley, E., & Asmundson, S. M. (1969). Free-running periods of endogenous circannian rhythms in the golden mantled ground squirrel, Citellus lateralis. Comparative Biochemistry and Physiology, 30(1), 177–183. https://doi.org/10.1016/0010-406x(69)91312-7
Pengelley, E., & Asmundson, S. J. (1975). Female gestation and lactation as zeitgebers for circannual rhythmicity in the hibernating ground squirrel, Citellus lateralis. Comparative Biochemistry and Physiology Part A: Physiology, 50(3), 621–625. https://doi.org/10.1016/0300-9629(75)903254
Porter, W. P., & Gates, D. M. (1969). Thermodynamic Equilibria of Animals with Environment. Ecological Monographs, 39(3), 227–244. https://doi.org/10.2307/1948545
Prendergast, B. J., Freeman, D. A., Zucker, I., & Nelson, R. J. (2002, April 1). Periodic arousal from hibernation is necessary for initiation of immune responses in ground squirrels. American Journal of Physiology-Regulatory, Integrative and Comparative Physiology, 282(4), R1054–R1062. https://doi.org/10.1152/ajpregu.00562.2001
Räber, M., Lischer, Ch. J., Geyer, H., & Ossent, P. (2004). The bovine digital cushion – a descriptive anatomical study. The Veterinary Journal 167(3), 258-264. https://doi.org/10.1016/S1090-0233(03)00053-4
Räber, M., Scheeder, M. R. L., Ossent, P., Lischer, Ch. J., & Geyer, H. (2006). The content and composition of lipids in the digital cushion of the bovine claw with respect to age and location – A preliminary report. The Veterinary Journal 172(1), 173-177. https://doi.org.proxy3.library.mcgill.ca/10.1016/j.tvjl.2005.03.009
Tavman, I. H., & Tavman, S. (1999). Measurement of thermal conductivity of dairy products. Journal of Food Engineering, 41(2), 109–114. https://doi.org/10.1016/s0260-8774(99)00079-5
Webb, D., & Schnabel, R. (1983). Functions of fat in hibernators: Thermal aspects. Journal of Thermal Biology, 8(4), 369–374. https://doi.org/10.1016/0306-4565(83)90024-4
Weissengruber, G. E., Egger, G. F., Hutchinson, J. R., Groenwald, H. B., Elsässer, L., Famini, D., & Forstenpointner, G. (2006). The structure of the cushions in the feet of African elephants (Loxodonta Africana). Journal of Anatomy 209(6), 781-792. https://doi.org/10.1111/j.1469-7580.2006.00648.x
Iverson, S. J. (2018). Encyclopedia of Marine Mammals. Academic Press. https://doi.org/10.1016/B978-0-12-373553-9.00032-8
Castellini, M. (2018). Encyclopedia of Marine Mammals. Academic Press. https://doi.org/10.1016/B978-0-12-804327-1.00258-2
Young, R. A. (1976). Fat, Energy and Mammalian Survival. American Zoologist, 16(4), 699–710. https://doi.org/10.1093/icb/16.4.699
Zhang, F., Hao, G., Shao, M., Nham, K., An, Y., Wang, Q., Zhu, Y., Kusminski, C. M., Hassan, G., Gupta, R. K., Zhai, Q., Sun, X., Scherer, P. E., & Oz, O. K. (2018). An Adipose Tissue Atlas: An Image-Guided Identification of Human-like BAT and Beige Depots in Rodents. Cell Metabolism, 27(1), 252-262.e3. https://doi.org/10.1016/j.cmet.2017.12.004