Biomolecular Factors for Immunity, Color Change, and Mechanical Strength of the Integument
Juyoun Bae, Andrei Bocan, Justin Charney, Lan Anh Huynh
Introduction
The skin is a highly complex organ. It contains many components which allow it to fulfil its functions. These components include various cells and proteins which are regulated by intricate biological pathways. Across different organisms, there is a large variety of these components, which exist at several levels of complexity and development. This variety is a direct result of the many functions fulfilled by the skin throughout the animal world.
The epidermis, dermis and hypodermis are the three layers comprising the skin. The epidermis is divided into the stratum germinativum, stratum spinosum, stratum granulosum, and the stratum corneum (“The Skin” | Anatomy and Physiology (Boundless) | Lumen Candela, 2020). The main cellular components of the epidermis are keratinocytes, Langerhans cells, melanocytes, and Merkel cells. Keratinocytes are constantly moving through the epidermal layers towards the skin surface. In the process, they lose their organelles and die, forming a layer of protective dead cells which makes up the stratum corneum (“The Skin” | Anatomy and Physiology (Boundless) | Lumen Candela, 2020). Langerhans cells are dendritic antigen-presenting cells of the adaptive immune system. Melanocytes are melanin-producing cells, and Merkel cells are mechanoreceptors (Yousef et al., 2020). Other types of pigment containing cells; xanthophores, erythrophores, and cyanophores, are found in the skin of physiological color changing vertebrates. The components of the epidermis are held together by cellular junctions, which also provide mechanical strength. Vertebrates rely on desmosomes and adherens junctions, while invertebrates rely only on adherens junctions (Green et al., 2020). The epidermal-dermal junction holds the epidermis and dermis together and allows the exchange of cells and fluids. The dermis is heavily vascularized and innervated (Kolarsick et al., 2011). It is largely composed of the proteins: elastin and collagen along with fibroblasts which assemble into collagen fibrils. The dermis also contains hair follicles, various types of glands, and mast cells, which are associated with allergic response (Kolarsick et al., 2011). The glands found in the dermis vary between vertebrate classes; in general, mammals have sebaceous and sweat glands (Kolarsick et al., 2011), while amphibians have mucous and granular glands. Peptides are also an important part of the skin, they are helpful for functions such as healing and immunity, among others (Demori et al., 2019).
Glands are a collection of secretory cells that produce different types of secretions found everywhere in every living organism. The focus of this report will be specifically on integument glands found in between the epidermal and dermal layers of amphibian skin. Mammalian glands are widely heard of and frequently studied (e.g., sweat glands, sebaceous glands, etc.); however, amphibian glands consisting of mucous (MG) and granular glands (GG) hold a more vital role in amphibian survival. They are also unique to these vertebrates and should receive more attention. Although these glands have a very conserved function and general chemical composition throughout all amphibians, the specificity of the operation and distribution of MGs and GGs vary drastically between fossil amphibians and lissamphibians. Comparison of the distribution and morphology of MGs and GGs in S. annulatus, a fossil amphibian, and T. verrucosus a lissamphibian will shed light onto the incredible roles of these cellular components.
Chromatophores are a group of pigment containing cells that can be either light-absorbing or light-reflecting. Melanophores, xanthophores, erythrophores, cyanophores are light-absorbing and pigment containing. Their pigment containing organelles are melanosomes, xanthosomes, erythrosomes, and cyanosomes respectively (Fujii, 2000). Light-reflecting iridophores contain guanine crystals forming light-reflecting platelets, and leucophores contain leucosomes. The most ubiquitous chromatophore is the melanophore found in virtually all vertebrates, whereas the others are specific to color changing vertebrates. The fish Astronotus ocellatus and the amphibian Rana ridibunda illustrate rapid color change, called physiological color change, through a host of neuronal and/or hormonal factors (Fujii, 2000). We will explore the most important neuronal and hormonal factors and discuss pathways, receptors, and motor proteins that enable the translocation of chromatosomes, facilitating color change.
Adherens junctions (AJ) and desmosomes are intercellular junctions that provide mechanical strength to the skin by holding epithelial cells together. This report explores the structures of each type of junction on the cellular level with focus on the different cell-adhesion molecules and the filaments that form each junction. Following is a comparison of intracellular interactions between the adhesion glycoproteins to generate mechanical strength in each type of junction. The origins of AJs can be traced back to the earliest periods of metazoan evolution and thus can be found in most types of animals including vertebrates, whereas desmosomes are a relatively new type of adhesion junction that provide further rigidity and elasticity for vertebrates. Particularly, we examine Caenorhabditis elegans (roundworm) that first introduced desmosomes in addition to adherens to the cell-junction mechanism. In doing so, we provide a basis to better understand its emergence through evolution.
Langerhans cells and antimicrobial peptides are important elements of cutaneous immunity. In this report, we examine these elements in amphibians and mammals. We see how the Langerhans cells of the mammalian epidermis may capture antigens and aid in adaptive immune responses. The minor role played by antimicrobial peptides in mammalian cutaneous immunity is also explored. We then see that the mucus layer covering the amphibian skin contains a large variety of microbial symbiotes and peptides, elements crucial for amphibian cutaneous immunity. Comparing Langerhans cells and peptides in amphibians and mammals will allow us to draw a clear picture of the structural and operational differences in skin immunity.
Glands
Glands are single cellular or multicellular components that are found in numerous dermal regions of living organisms. In particular, exocrine glands can be found in large numbers on amphibian skin distinguishing these animals from other vertebrate groups (Pereira et al., 2018). These cellular secretory components can be found embedded in the dermis of their skin and unique to amphibians are granular and mucous glands; they produce secretions with multiple purposes: as a lubricant, as bioadhesion or as a water, microbial or chemical barrier (Wanninger et al., 2018). Although granular (serous) — produce toxins —and mucous glands are common in amphibians, their distribution, size and secretions are all varied and specific to each amphibian based on the environment and particular necessities. Examined below will be the comparison between fossil amphibians and lissamphibians — “smooth” amphibians: referring to current amphibians excluding fossil amphibians (“Lissamphibia” | Merriam-Webster, n.d.) — as well as the exploration of possible drug and biomimetic applications from the study of the biochemical composition of granular and mucous secretions.
From previous studies, the distribution of mucous and granular glands along the body of the caecilian Siphonops annulatus has long been known. S. annulatus is a limbless fossil amphibian found in Southeast Asia, Central and South America and Africa that has larger and higher concentrations of poison glands in their posterior ends (Jared et al., 2018). The study by Jared and his team (2018) looked at the possible reasons behind this morphology.

The study looked at histological analysis of the S. annulatus skin section to compare the distribution of mucous and poison glands along their entire body. Mucous glands were discovered to be of two types: Type I and Type II (Jared et al., 2018). Starting from the head, Type I glands were enlarged and numerous and progressively decreased in number and size further away from the head region (Fig. 1). From Fig. 1 we also see that the poison glands (g) are large and concentrated at the posterior end (Fig. 1f) and as we move up the body towards the head the size and number of poison glands also decrease (Jared et al., 2018). Another observation made is the homogeneous distribution of Type II mucous glands along the entire body of the S. annulatus. However, the heterogeneous distribution of the glands observed above is reported to be lacking attention from the research community and therefore the reason behind such distribution still remains unknown (Jared et al., 2018). Although details on the chemical composition of secretion contents are relatively unknown, the study was able to identify a more abundant and diverse composition of proteins in the secretion sample at the posterior end as opposed to samples taken at the head region, which were much more toxic. In addition, Type I mucous glands concentrated in the anterior region contained more lipid content in its secretions which can be used to reduce friction between the skin of S. annulatus and soil (Jared et al., 2018). Therefore, from both morphological and biochemical analysis of the glands and their operation, the conclusion that glands relate to the “locomotion and defense against predators in the fossorial environment” (Jared et al., 2018) can be made. The team also suggests that the funnel-like head of the amphibian, that it uses to dig through the soil, contains large concentrations of mucous Type I glands to lubricate the body with its lipid containing mucus and reduce friction as the animal moves through soil. Additionally, the posterior end which is enlarged in size due to the numerous and large poison glands is used not only to physically block potential predators but also secretes toxins creating a chemical barrier protecting the S. annulatus (Jared et al., 2018).
Lissamphibian gland secretions have very similar functions to fossil am- phibians; however, there is an increase in the complexity of the architecture and operation. Certain secretions are used as a lubricant to help amphibians “slip off” and escape predators, and minimize friction underwater (Wanninger et al., 2018), whilst others act as bioadhesives to immobilise a predator (Evans and Brodie, 1994). These gland secretions also take part in wet adhesives seen in tree frogs (Langowski et al., 2019). Some lissamphibian mucus is found to contain antimicrobial peptides that aid in microbial pathogen protections (see below); others regulate water loss by acting as a water barrier (Wanninger et al., 2018). Wanninger et al.'s study (2018) also highlights that secretions can be toxic or present the amphibian skin as being unpalatable to predators.
T. verrucosus — newts— like other amphibians, also have glandular tissues and in particular have two types of granular glands (GG1 and GG2) and a mucous gland (MG) (Wanninger et al., 2018). Unlike S. annulatus, the distribution of the glands in newts are not as distinct; scanning electron microscopy (Fig. 2) identified regions with high densities of cutaneous glands whilst others were fewer packed as well as smaller in size with the most notably dense areas being in the parotoid and the dorsal trunk region (Wanninger et al., 2018).

The researchers also noticed that GGs and MGs were both covered in a myoepithelial sheath, although MG sheaths were disconnected whilst GGs had a dense concentration of smooth muscles around the gland enabling rapid and efficient discharge of toxic secretions in a stress-evoked situation (Wanninger et al., 2018). This set up allows T. verrucosus to be able to fine-tune gland secretion accordingly based on external stimuli, effectively protecting itself from danger using a chemical barrier. This secretion mechanism is controlled by the sympathetic nervous system's response to varying degrees of external stimuli and neurotransmitter molecules' binding to alpha-adrenergic receptors leading to the contraction of the myoepithelial; a mechanism that does not follow the all or none law (Wanninger et al., 2018). Being one of the main defensive strategies against predators, maximization of the efficiency of toxic discharge is crucial for these vertebrates. To further optimize their defense mechanism, some amphibians such as African frogs and salamanders (Heiss et al., 2009) go to the extent of implementing the direct transfer of these poisonous secretions directly into the bloodstream of their predators through causation of wounds (Blackburn et al., 2008).
Chromatophores
Integument functions like camouflage, social signaling, thermoregulation, and even immunity are mediated by chromatophores and their pigment containing organelles. Vertebrates' pigment cells are derived from neural crest cells, an embryonic population of cells that emerges from the dorsal neural tube (Fig. 3) which migrates under the control of several pathways and differentiates into as many as six types of pigment cells depending on the organism (Hirata et al., 2003). In the lower vertebrate teleosts — infraclass of ray-finned fish (Weitzman, 2018) — and amphibians, the color change of the integument is often rapid and regulated by neuronal, hormonal, or paracrine mechanisms. The same cannot be said for the color change observed in the integument of some mammals or birds, during development or seasonally, since their chromatophores lack many components enabling bidirectional color change in other vertebrates (Aspengren et al., “Different strategies for color change”).
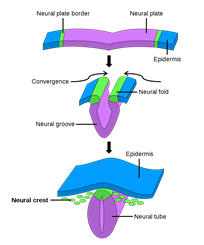
The color change in vertebrates can be separated into morphological and physiological types. Morphological color change results from altering the density of chromatophores in the integument (Aspengren et al., “New insights into melanosome transport) and does not allow for the rapid color change that will be detailed in this report. Physiological color change is of interest as it enables several unique functions of the integument in fish and amphibians through the rapid translocation of pigment containing organelles (Sköld et al., 2013). It is noteworthy that chromatophore distribution is distinct among vertebrates, with melanophores being present in all species and generally playing the largest role (Fujii, 2000).
Amphibians and fish provide an interest basis for a biochemical comparison of chromatosome motility since they have both similar and divergent mechanisms for color change. Whereas fish use hormonal and neuronal regulation to induce a rapid change in chromatosome distribution, amphibian response is slower as it is regulated by hormonal factors alone (Aspengren et al., “Different strategies for color change”). However, before exploring their differences in chromatophore regulation, we will detail the fundamental molecular mechanisms that perform the translocation of chromatosomes within pigment cells. Movement of these pigment containing organelles is dependent on microtubules, actin filaments, and their motor proteins dynein, kinesin, and myosin (Aspengren et al., “New insights into melanosome transport).
In both amphibians and fish, melanosome aggregation, resulting in a paling effect, is due to dynein which moves the pigment containing organelles toward the negative pole of the microtubules. Dispersion of melanosomes, which results in skin darkening (Figure 4), is facilitated by both microtubules and actin filaments and their respective motor proteins (Sugden et al., 2004).

In amphibians, kinesin-II is responsible for dispersion and is constantly active, unlike regular kinesin which controls dispersion in fish (Aspengren et al., “Different strategies for color change”). Additionally, in amphibians and fish, the actin motor protein myosin-V aids kinesin in a “tug- of-war” with dynein, leading to net dispersion while also maintaining proper dispersion by preventing the melanosomes from being irreversibly caught in the cell periphery. This tug-of-war does not seem to be the case in melanosome aggregation since blocking of kinesin does not increase aggregation speed (Sugden et al., 2004).
The motile activity of chromatosomes in fish is highly regulated, particularly by neuronal stimuli. An interesting example that illustrates the biomolecular mechanisms of color change in fish is with Astronotus ocellatus. When defeated in combat or startled, A. ocellatus changes the color of its integument from an olive-brown to near black with white barring (Fig. 5) for conspecific communication to inhibit aggression (Beeching, 1995). Changes in coloration were measured to occur fully within two seconds for A. ocellatus, which is likely due to a combination of (MCH) and α-melanophore stimulating hormone (α-MSH). These hormones/neurotransmitters are the most widely known and are important for melanin aggregation and dispersion respectively, thus we will now turn our focus to their role in chromatophore regulation (Beeching, 1995).

MSH is secreted by the intermediate lobe of the pituitary gland and induces rapid dispersion of melanosomes within melanophores. In fish, α-MSH, derived from the precursor proopiomelanocortin, also disperses the pigment organelles of xanthosomes and erythrosomes (Beeching, 1995). Conversely, in the light reflecting motile iridophores, it has also been shown to cause aggregation of the guanine light-reflecting platelets, allowing for the reflection of shorter wavelength light (Fujii, 2000). The combination of the aggregation in light-reflecting chromatophores and dispersion in light-absorbing ones allows for the darkening of the skin (and vice versa for paling). In general, it is observed that neuronal or hormonal stimulus induce inverse responses in light-absorbing and light-reflecting chromatophores. The effect of α-MSH on fish chromatophores is mediated by MSH receptors which in his paper, Fujii (2000) deemed α and β MC-R receptors to differentiate between those on light-absorbing and light scattering chromatophores respectively.
The skin of amphibians contains several peptides that regulate the secretion of α-MSH from the pars intermedia of the pituitary. The pars intermedia in amphibians is composed of melanotrope cells that are connected to neural fibers containing neurotransmitters and regulatory peptides that influence the melanotrope cells through neuroendocrine factors. In the frog Rana ridibunda, thyrotropin-releasing hormone (TRH) stimulates the release of MSH; the skin of amphibians has a very high concentration of TRH relative to other body parts (Vaudry et al., 1999). In R. ribidunda, TRH is produced by cells in the poison glands and the observation of high concentrations of TRH in the blood of R. ribidunda suggests TRH produced by the skin can be released into the bloodstream. Additionally, the arboreal frog Phyllomedusa bicolour produces a peptide called skin peptide tyrosine-tyrosine (SPYY) that is found in very high concentrations on the skin. SPYY is an inhibitor of α-MSH secretion that when looked at with TRH, suggests a possible regulatory loop between the skin and pituitary of amphibians (Vaudry et al., 1999).
Noradrenaline is the most important neurotransmitter in chromatosome motility, allowing for the rapid aggregation of melanosomes. In response to neural stimuli, noradrenaline is released from the synaptic cleft, where it binds α-adrenoreceptors that mediate its aggregating action (Fujii, 2000). Noradrenaline has also been shown to have a similar response on xanthophores and erythrophores; however, it is less pronounced. Conversely, neuronal stimuli have been shown to have dispersing effects on the light scattering organelles in leucophores mediated by the cell surface β-adrenoceptors (Fujii, 2000). In addition to the release of the primary transmitter noradrenaline, there is a simultaneous release of the co-transmitter ATP which allows for re-dispersion after aggregation prompted by noradrenaline. The mechanism for this requires ATP to be converted into adenosine in the synaptic cleft through dephosphorylation by ATPase and 5'- nucleotidase before binding to cell surface adrenoreceptors (Fujii, 2000).
Melatonin is a hormone secreted from the pineal gland that is present in both amphibians and fish; however, its chromatosome aggregating response is particularly important to amphibians whose chromatophores lack neuronal regulation. The hormone binds to the mel1c-receptor (a G-coupled protein receptor) which then decreases cAMP and inactivates protein kinase, a prompting melanosome aggregation, and skin paling (Sugden et al., 2004). It has also been shown to aggregate other light-absorbing chromatosomes; it is worth noting that melatonin does not cause aggregation in certain fish or regions of fish skin, which could allow for the appearance/disappearance of fish color patterns. Interestingly, the fish Nannostomus beckfordi is known to have darkening regions of its integument during the evening when melatonin levels are highest, suggesting the presence of melatonin receptors that mediate dispersion, deemed β-MT receptors by Fujii (2000).
Cell Junctions
The stratifying epidermis of the skin functions as a barrier that provides the skin with mechanical strength capable of withstanding physical assaults. Apart from the keratinocytes that form the outermost protective dead layer of the skin, the living cells themselves form a barrier by providing tight mechanical cohesion between the cells of the same and different epidermal layers (Brandner et al., 2010). To establish this barrier, the viable cells connect to each other by intercellular junctions such as adherens junctions and desmosomes, whose architectures and operations are subject to analysis on a biomolecular and cellular level. Further analysis in the evolutionary perspective can be done to answer how and why invertebrates depend on adherens junctions for skin mechanical strength whilst vertebrates depend on both adherens and desmosomes for the same function.

Adherens junctions are systems of intercellular structures that adhere to the cytoskeleton to create a transcellular network that coordinates the physical behavior of cells (Brandner et al., 2010). Adherens junctions anchor bundles of actin microfilaments with classical cadherins, such as E-cadherin, as their transmembrane glycoproteins (Amagai, 1995). Cadherins are a multiple gene family of Ca 2+-dependent cell adhesion molecules with a typical single-spanning transmembrane structure. Cadherins have two major subfamilies, classic cadherin and desmosomal cadherin (Amagai, 1995). E-cadherin is a single-pass, transmembrane glycoprotein that belongs to the classical cadherin family of Ca2+-dependent adhesion proteins (Hartsock and Nelson, 2008). To elaborate, the classical cadherins form a cell adhesion receptor complex (Fig. 6b) called the classical cadherin/catenin complex capable of linking to the actin cytoskeleton (Brandner et al., 2010). The classical cadherins initiate intercellular contacts through trans-pairing between cadherins on opposing cells on two different binding regions: juxtamembrane domain (JMD) and catenin binding domain (CBD) (Fig. 7). The E-cadherins also bind to many cytoplasmic proteins like the previously mentioned catenin family, which locally regulates the organization of the actin cytoskeleton providing cadherin stability. Upon the formation of intercellular contacts, cadherins cluster then spread laterally, thereby strengthening the contact. Not only are these cadherins responsible for generating the mechanical strength in epithelial cells, but they are also responsible for maintaining them. In this regard, the classical cadherins bind to specific proteins that regulate E-cadherin endocytosis, recycling and degradation, intracellular signaling, and gene transcription, in addition to those that control the actin cytoskeletons, for the continual replacements of functional transmembrane glycoproteins (Hartsock and Nelson, 2008).
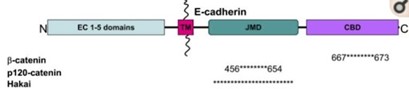
The elements of adherens junctions were first assembled as a complex linking the actin cytoskeleton to the plasma membrane during metazoan evolution (Fig. 8). Genes encoding both the transmembrane and cytoplasmic components of the adherence junctions are present in animals as diverse as sponges and vertebrates, indicating that this complex emerged during the earliest periods of animal evolution (Green et al., 2020). Likewise, in invertebrates, the formation and function of epithelial sheets depends on classical cadherin-containing adherens junctions. Caenorhabditis elegans, however, is exceptional in that it marked the occurrence of linkage between intermediate filaments and membrane junctions in invertebrates before vertebrates. In contrast to other invertebrates which have functionally and physically distinct junctions, epithelial cells contain a single junction that executes both barrier and adhesive functions. The apical junction (CeAJ) of C. elegans is positioned near the apicolateral interface, consisting of adherens junction and DAC junction (Fig. 8). The classical adherens junction components of the CeAJ are not necessary in providing robust adhesion between epithelial cells. Instead, the functional proteins in the CeAJ assemble to control the actin cytoskeletal organization in a hemidesmosome-like structure that links intermediate filaments to the extracellular matrix to ensure tissue integrity (Armenti and Nance, 2012). This effectively introduces the emergence of a new type of intercellular junction called the desmosomes in vertebrates.

Desmosomes are cell-cell adhesion complexes that are composed of the desmosomal cadherins, similar to the classical cadherins of adherens junctions, and are part of the cadherin superfamily (Brandner et al., 2010). These complexes link intracellularly to the intermediate filament cytoskeleton, forming bonds in a network that gives mechanical strength to epithelial tissues. This network is called the desmosome intermediate filament complex (DIFC). The DIFC is divided into three components: two intracellular one intercellular (Fig. 9).

Intracellularly, there are the intermediate filaments (IF) and the linkage between the IF and the desmosomal adhesion molecules (desmosomal cadherins), mediated by desmoplakin — major component of the desmosome that connects cytoskeletal networks to the plasma membrane, integrating actin, microtubules, and intermediate filaments (Kline and Mohler, 2013) — and the armadillo proteins plakoglobin and plakophilin (Garrod and Chidgey, 2008). Intercellularly, there is the adhesive bond provided by the cadherins. The desmosomal adhesiveness comes from the “hyper-adhesive” state, characterized by calcium independence, by which desmosomes are resistant to disruption by chelating agents — organic compounds that may link together metal ions to form complex ring-like structures called chelates (Flora et al., 2009) — Hyper-adhesion is the normal adhesive state of epithelial desmosomes, keeping the skin strongly adhesive at all times with enough mechanical strength to resist disruption by physical forces. Hyper-adhesiveness is a property unique to desmosomes, which is why they are able to provide a strong link in the DIFC chain (Garrod and Chidgey, 2008).
Desmosomes, as mentioned earlier, are a relatively new type of cadherin-based intercellular junction that is common to vertebrates. While desmosomes have a molecular blueprint that is similar to that of adherens junctions (Fig. 6a), desmosomal cadherins link intermediate filaments rather than actin filaments to the plasma membrane (Green et al., 2020). Importantly, its ability to anchor the highly elastic and tough IF cytoskeleton endows vertebrates with robust tissues and increased mechanical integrity, thereby making the vertebrate skin well suited for resisting and responding to environmental stress unique to vertebrates (Green et al., 2020).
Adherens junctions and desmosomes are two different types of cadherin-based junctions that are capable of providing the epithelial tissues of the skin with sufficient mechanical strength to withstand physical assaults (Green et al., 2020). Whereas adherens junction anchor actin microfilaments, desmosomes link intermediate filaments to the plasma membrane. Each junction has structural differences that lead to different processes of endowing the epidermis with mechanical strength. In invertebrates, cadherin-based junctions are limited to adherens junctions (AJ). In vertebrates, AJs persist, but there are also desmosomes providing increased elasticity and rigidity to the skin (Green et al., 2020).
Immunity
The first vertebrates to colonize land did so some 400 million years ago. Some of them evolved into mammals, while others became today's amphibians (Hanken, 1989). Over the course of this evolution, both groups developed in different ways. An example of this is skin; amphibian skin is more permeable, to allow for gas and ion exchange, as well as osmoregulation. This permeability increases susceptibility to pathogens, so amphibian skin is covered in a layer of mucus containing various elements crucial for stopping pathogens. Mammalian skin is less permeable. Its outermost layer, the stratum corneum resembles a brick wall, and is thus better able to stop pathogens (Quaranta et al., 2009). Similar elements of cutaneous immunity are found in both amphibian and mammalian skin, namely Langerhans cells and antimicrobial peptides.
Langerhans cells (LC) populate the mammalian epidermis, accounting for 3 – 5 % of epidermal cells. LCs are a type of antigen-presenting cell (APC). APCs process and present antigens to T cells (or other lymphocytes), which enables an adaptive immune response (Deckers et al., 2018). LCs are dendritic in nature, have a clear cytoplasm, and lack any desmosomes (Fig. 10). They are also characterized by their convoluted nucleus and the presence of a cytoplasmic granule (Birbeck granule) (Zelickson, 1965). In the mammalian epidermis, LCs occupy the spaces between neighboring keratinocytes (Merad et al., 2008). Above the LCs, the tight junction is found, it is a barrier made up of cell-cell junctions (Kirschner et al., 2010), under the stratum corneum.
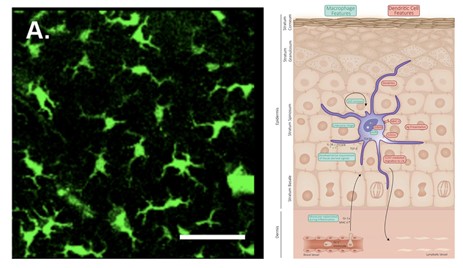
When activated, LCs push their dendrites through the tight junction and take up external antigens (Matsui and Amagai, 2015). Langerin, a C-type lectin receptor, which is highly expressed by mammalian LCs, plays an important role in the capture of pathogens. When a cell-surface langerin is engaged by a langerin-specific antibody, it is internalized and directed to a Birbeck granule, where antigen processing likely occurs (Merad et al., 2008). A few studies have demonstrated the existence of LC-like cells in the skin of the amphibian species Rana pipiens, Rana catesbeiana, and Xenopus laevis (Castell-Rodriguez et al., 2001; Carillo-Farga et al., 1990; Mescher et al., 2007). The function of these cells in amphibians is yet to be described, though it is likely that they behave similarly to mammalian LCs. However, amphibians have a less developed adaptive immune system (Xu and Lai, 2015), so these cells likely play a more significant role in mammals.
Antimicrobial peptides (AMPs) are involved in nonspecific immune responses. They generally target bacteria, fungi, protozoa, and viruses. AMPs have a net positive charge and bind to negatively charged phospholipids in cell membranes of bacteria (or anionic components of fungi/viruses) (Afshar and Gallo, 2013). They insert into the phospholipid bilayer, forming pores. These pores disrupt the cell membrane and lead to lysis. The principal AMPs found in mammals are cathelicidins, β-defensins, dermcidin, and psoriasin, which are synthesized within a variety of tissues (Afshar and Gallo, 2013). In contrast, in the amphibian Xenopus genus, more than 60 AMPs have been identified in skin secretions. They are divided into the families magainin, CPF, peptide glycine-leucine amide (PGLa), and xenosin-precursor fragment (XPF). Some AMPs in Xenopus appear to lack antimicrobial activity; the genes encoding them are non-functional. 13 AMP families are also found in the amphibian genus Rana (Xu and Lai, 2015). Many amphibian peptides have been found to inhibit the growth of fungal pathogens such as Batrachochytrium dendrobatidis (Bd) and Basidiobolus ranarum (Br). Bd is a fungal pathogen which causes the skin disease chytridiomycosis. This disease affects amphibian populations worldwide and has caused the decline of over 200 amphibian species. Magainin, PGLa, and CPF, from Xenopus, as well as ranalexin from Rana all inhibit growth of Br. Magainin and PGLa also act in synergy to inhibit growth of Bd and Br. AMPs from Xenopus and Rana were found to be somewhat effective against the iridovirus, frog virus 3, the herpesvirus, and the channel catfish virus, pointing to the increased importance of these molecules in amphibian cutaneous immunity (Grogan et al., 2018; Rollins-Smith et al., 2005). AMPs are produced in the granular glands of the amphibian dermis. Surrounding these glands is a layer of smooth muscle cells innervated by sympathetic nerves (Fig. 11). AMPs are released upon stimulation of the sympathetic nervous system (which occurs during injury or infection) (Rollins-Smith et al., 2005).
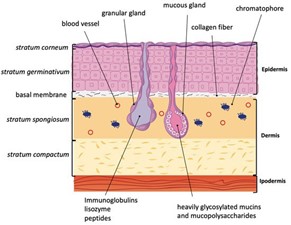
The skin mucus of amphibians is also home to symbiotic microbial communities. These communities are predominantly composed of bacteria, but also include archaea, fungi, viruses, protozoans, and a few multicellular eukaryotes. In the frog Rhinella marina, 0.5-1.7×106 bacterial colonies and 1.6-2.6×104 fungal colonies were found per square centimeter of dorsal skin. Together, these microorganisms secrete various antimicrobial compounds, which have repellent and growth inhibitive properties against pathogenic microbes (Grogan et al., 2018). To regulate these communities and prevent dysbiosis events, frogs use sloughing, where cells of the stratum corneum detach, thereby removing some microorganisms (Colombo et al., 2015). Sloughing is also a defense mechanism against external pathogens (Grogan et al., 2018). The exact composition of the cutaneous microbial community depends largely on the species of the host, while the host's environment plays a smaller but significant role (Kueneman et al., 2014). Apart from AMPs and microorganisms, the amphibian mucus also contains lysozyme and mucosal antibodies (Grogan et al., 2018).
The skin mucus layer is the main driver of cutaneous immunity in amphibians. Due to their weaker adaptive immune system, amphibians rely more heavily on the AMPs and microbial symbiotes found within this protective layer. Comparatively, mammals rely on the adaptive immune function of their Langerhans cells, and the wall-like function of their stratum corneum, to fulfil the function of cutaneous immunity.
Conclusion
Gland secretions in amphibians are important to several biological functions which could also prove useful to human engineering problems. Therefore, it is logical to question the biomimetic applications that these findings could inform. Back in 1994, a study by Evans and Brodie demonstrated the incredible adhesive strength of granular secretions in certain anurans including but not limited to: Ambystoma gracile (Brodie and Gibson, 1969), Ambystoma jeffersonianum (Rand, 1954, as cited in Evans and Brodie, 1994), Plethodon cinereus and Batrachoseps attenuatus (Arnold,1982), even surpassing adhesion strength seen in rubber cement. All the species tested excluding the B.meliana, were found to have secretions stronger than rubber cement; in particular, the D. antongilii (Madagascar tomato frog) was found to have secretion adhesion strength five times stronger (refer to Table 1 in Appendix) (Evans and Brodie, 1994). The study postulated that species with weaker secretion strength may be using it for chemical defense instead, proposing an inversely proportional relationship between adhesive strength and toxicity or distastefulness. Although the findings open potential research pathways in adhesives taking inspiration from these amphibians, we still do not have enough research and interest on its chemical composition to garner understanding on how they operate; thus, amphibian secretion chemical composition with regards to its adhesive function should be a potential topic of interest for future studies.
The biochemical pathways involved in physiological color change via chromatophore regulation are fascinating and under-explored in many vertebrates, especially amphibians. Further research could yield greater understanding into the neural, hormonal, and endocrine factors along with the various receptors that allow for color change. Implications of this knowledge are also wide-ranging, from allowing fishery scientists to better assist fish in survival, to making t-shirts that react to intense light on hot days by aggregating light-reflecting crystals within fabric to improve thermoregulation. With much more to uncover about the nature of color change, its future is very bright.
Cell adhesion junctions, particularly adherens junctions and desmosomes, are very complex yet significant systems involving many different epithelial components. The delicate mechanisms that provide barrier functions to animal skin can give rise to various future research with great potential for scientific improvements. Specifically, one can examine the features that separated desmosomes from AJs along the evolutionary timeline. Desmosomes, unlike AJs, can switch from calcium-dependent to calcium-independent status through hyper-adhesion, which provides extra flexibility and toughness in addition to that conferred on tissues by intermediate filaments. We expect that further research done on this property will bring about ways to help organisms to dynamically resist and respond to environmental stress. To elaborate, desmosomes could become more dynamic or tougher in response to mechanical or other types of physical stress on the skin. Likewise, enabling facilitated control over regulatory modules that mediate this switch to hyper–adhesion would be a ground-breaking discovery towards the increased flexibility or rigidity of desmosomes, especially for those animals that are prone to various types of physical stress on their skin.
The layer of mucus found on amphibian skin is effectively an immune “wall”. The variety of anti-pathogenic agents it contains form a barrier against pathogen attack. Despite this, the skin remains permeable, to allow for gas exchange and osmoregulation. In contrast, the mammalian epidermis is less permeable and similar to a real wall. Thus, nature uses a variety of interesting designs, each tailored to a given organism's needs, to fulfil the same function. Further research is needed to clarify certain aspects of cutaneous immunity. In mammals, the role of Birbeck granules in Langerhans cells must still be clarified. Little is known at the moment about these cells in amphibians. Additional research is required to clarify how much similarity exists between amphibian and mammalian LCs. Amphibian antimicrobial peptides also require further investigation. While the properties of individual peptides are well described, there is little information on how mixtures of these peptides behave in vivo, and how different peptides interact. Future research should focus on how AMPs act against chytridiomycosis, to better understand the natural defenses of amphibians against this disease, and how they might be enhanced.
Appendix
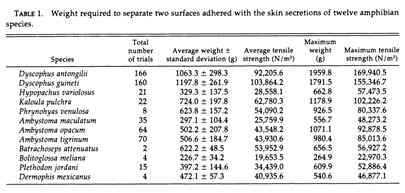
References
Afshar, M., & Gallo, R. L. (2013). Innate immune defense system of the skin. Veterinary Dermatology, 24(1), 32-e39. doi:https://doi.org/10.1111/j.1365-3164.2012.01082.x
Amagai, M. (1995). Adhesion molecules. I: Keratinocyte-keratinocyte interactions; cadherins and pemphigus. Journal of Investigative Dermatology, 104(1), 146-152. doi:10.1111/1523-1747.ep12613668
Armenti, S. T., & Nance, J. (2012). Adherens junctions in C. elegans embryonic morphogenesis. Sub-Cellular Biochemistry, 60, 279-299. doi:10.1007/978-94-007-4186-7_12
Arnold, S. J. (1982). A Quantitative Approach to Antipredator Performance: Salamander Defense against Snake Attack. Copeia, 1982(2), 247-253. doi:10.2307/1444602
Aspengren, S., Hedberg, D., Sköld, H. N., & Wallin, M. (2009). New insights into melanosome transport in vertebrate pigment cells. International Review of Cell and Molecular Biology, 272, 245-302. doi:10.1016/s1937-6448(08)01606-7
Aspengren, S., Sköld, H. N., & Wallin, M. (2009). Different strategies for color change. Cell Mol Life Sci, 66(2), 187-191. doi:10.1007/s00018-008-8541-0
Beeching, S. C. (1995). Colour pattern and inhibition of aggression in the cichlid fish Astronotus ocellatus. Journal of Fish Biology, 47(1), 50-58. doi:https://doi.org/10.1111/j.1095-8649.1995.tb01872.x
Blackburn, D. C., Hanken, J., & Jenkins, F. A. (2008). Concealed weapons: erectile claws in African frogs. Biology Letters, 4(4), 355-357. doi:doi:10.1098/rsbl.2008.0219
Brandner, J., Haftek, M., & Niessen, C. (2010). Adherens Junctions, Desmosomes and Tight Junctions in Epidermal Barrier Function. The Open Dermatology Journal, 4, 14-20. doi:10.2174/1874372201004020014
Brodie, E. D., & Gibson, L. S. (1969). Defensive Behavior and Skin Glands of the Northwestern Salamander, Ambystoma gracile. Herpetologica, 25(3), 187-194. Retrieved from http://www.jstor.org/stable/3891393
Candela, L. (2020). The Skin. In Anatomy and Physiology (Boundless). Retrieved from https://courses.lumenlearning.com/boundless-ap/chapter/the-skin/
Carrillo-Farga, J., Castell, A., Pérez, A., & Rondán, A. (1990). Langerhans-like cells in amphibian epidermis. Journal of Anatomy, 172, 39-45.
Castell-Rodríguez, A. E., Sampedro-Carrillo, E. A., Herrera-Enriquez, M. A., & Rondán-Zárate, A. (2001). Non-specific esterase-positive dendritic cells in epithelia of the frog Rana pipiens. Histochemical Journal, 33(5), 311-316. doi:10.1023/a:1017985209296
Colombo, B. M., Scalvenzi, T., Benlamara, S., & Pollet, N. (2015). Microbiota and Mucosal Immunity in Amphibians. Frontiers in Immunology, 6(111). doi:10.3389/fimmu.2015.00111
Commons, W. (2014). Neural crest In N. crest.svg (Ed.), (pp. Neural crest formation during neurulation.). Wikimedia Commons. Retrieved from https://commons.wikimedia.org/wiki/File:Neural_crest.svg
Deckers, J., Hammad, H., & Hoste, E. (2018). Langerhans Cells: Sensing the Environment in Health and Disease. Frontiers in Immunology, 9, 93. doi:10.3389/fimmu.2018.00093
Demori, I., El Rashed, Z., Corradino, V., Catalano, A., Rovegno, L., Queirolo, L., . . . Grasselli, E. (2019). Peptides for Skin Protection and Healing in Amphibians. Molecules, 24(2), 347. Retrieved from https://www.mdpi.com/1420-3049/24/2/347
Dictionary, M.-W. (Ed.) (n.d.). Lissamphibia. In. merriam-webster.com: Merriam-Webster. Retrieved from https://www.merriam-webster.com/dictionary/Lissamphibia
Evans, C. M., & Brodie, E. D. (1994). Adhesive Strength of Amphibian Skin Secretions. Journal of Herpetology, 28(4), 499-502. doi:10.2307/1564965
Flora, S. J. S., Flora, G., & Saxena, G. (2009). CHAPTER 9 – Arsenicals: Toxicity, their Use as Chemical Warfare Agents, and Possible Remedial Measures. In R. C. Gupta (Ed.), Handbook of Toxicology of Chemical Warfare Agents (pp. 109-133). San Diego: Academic Press.
Fujii, R. (2000). The Regulation of Motile Activity in Fish Chromatophores. Pigment cell research / sponsored by the European Society for Pigment Cell Research and the International Pigment Cell Society, 13, 300-319. doi:10.1034/j.1600-0749.2000.130502.x
Garrod, D., & Chidgey, M. (2008). Desmosome structure, composition and function. Biochim Biophys Acta, 1778(3), 572-587. doi:10.1016/j.bbamem.2007.07.014
Green, K. J., Roth-Carter, Q., Niessen, C. M., & Nichols, S. A. (2020). Tracing the Evolutionary Origin of Desmosomes. Current Biology, 30(10), R535-R543. doi:10.1016/j.cub.2020.03.047
Grogan, L. F., Robert, J., Berger, L., Skerratt, L. F., Scheele, B. C., Castley, J. G., . . . McCallum, H. I. (2018). Review of the Amphibian Immune Response to Chytridiomycosis, and Future Directions. Frontiers in Immunology, 9(2536). doi:10.3389/fimmu.2018.02536
Hanken, J. (1989). Development and Evolution in Amphibians. American Scientist, 77(4), 336-343. Retrieved from http://www.jstor.org/stable/27855832
Hartsock, A., & Nelson, W. J. (2008). Adherens and tight junctions: structure, function and connections to the actin cytoskeleton. Biochim Biophys Acta, 1778(3), 660-669. doi:10.1016/j.bbamem.2007.07.012
Heiss, E., Natchev, N., Rabanser, A., Weisgram, J., & Hilgers, H. (2009). Three types of cutaneous glands in the skin of the salamandrid Pleurodeles waltl. A histological and ultrastructural study. Journal of Morphology, 270(7), 892-902. doi:10.1002/jmor.10728
Hirata, M., Nakamura, K., Kanemaru, T., Shibata, Y., & Kondo, S. (2003). Pigment cell organization in the hypodermis of zebrafish. Developmental Dynamics, 227(4), 497-503. doi:10.1002/dvdy.10334
Jared, C., Mailho-Fontana, P. L., Marques-Porto, R., Sciani, J. M., Pimenta, D. C., Brodie, E. D., & Antoniazzi, M. M. (2018). Skin gland concentrations adapted to different evolutionary pressures in the head and posterior regions of the caecilian Siphonops annulatus. Scientific Reports, 8(1), 3576. doi:10.1038/s41598-018-22005-5
Kirschner, N., Houdek, P., Fromm, M., Moll, I., & Brandner, J. M. (2010). Tight junctions form a barrier in human epidermis. European Journal of Cell Biology, 89(11), 839-842. doi:10.1016/j.ejcb.2010.07.010
Kline, C. F., & Mohler, P. J. (2013). Chapter Four – Evolving Form to Fit Function: Cardiomyocyte Intercalated Disc and Transverse-Tubule Membranes. In V. Bennett (Ed.), Current Topics in Membranes (Vol. 72, pp. 121-158): Academic Press. Retrieved from https://www.sciencedirect.com/science/article/pii/B9780124170278000040
Kolarsick, P. A. J., Kolarsick, M. A., & Goodwin, C. (2011). Anatomy and Physiology of the Skin. Journal of the Dermatology Nurses' Association, 3(4), 203-213. doi:10.1097/JDN.0b013e3182274a98
Kueneman, J. G., Parfrey, L. W., Woodhams, D. C., Archer, H. M., Knight, R., & McKenzie, V. J. (2014). The amphibian skin-associated microbiome across species, space and life history stages. Molecular Ecology, 23(6), 1238-1250. doi:10.1111/mec.12510
Langowski, J. K. A., Singla, S., Nyarko, A., Schipper, H., van den Berg, F. T., Kaur, S., . . . van Leeuwen, J. L. (2019). Comparative and functional analysis of the digital mucus glands and secretions of tree frogs. Front Zool, 16, 19. doi:10.1186/s12983-019-0315-z
Matsui, T., & Amagai, M. (2015). Dissecting the formation, structure and barrier function of the stratum corneum. International Immunology, 27(6), 269-280. doi:10.1093/intimm/dxv013
Merad, M., Ginhoux, F., & Collin, M. (2008). Origin, homeostasis and function of Langerhans cells and other langerin-expressing dendritic cells. Nature Reviews: Immunology, 8(12), 935-947. doi:10.1038/nri2455
Mescher, A. L., Wolf, W. L., Ashley Moseman, E., Hartman, B., Harrison, C., Nguyen, E., & Neff, A. W. (2007). Cells of cutaneous immunity in Xenopus: Studies during larval development and limb regeneration. Developmental & Comparative Immunology, 31(4), 383-393. doi:10.1016/j.dci.2006.07.001
Pereira, K. E., Crother, B. I., Sever, D. M., Fontenot, C. L., Jr., Pojman, J. A., Sr., Wilburn, D. B., & Woodley, S. K. (2018). Skin glands of an aquatic salamander vary in size and distribution and release antimicrobial secretions effective against chytrid fungal pathogens. Journal of Experimental Biology, 221(Pt 14). doi:10.1242/jeb.183707
Quaranta, A., Bellantuono, V., Cassano, G., & Lippe, C. (2009). Why amphibians are more sensitive than mammals to xenobiotics. PloS One, 4(11), e7699. doi:10.1371/journal.pone.0007699
Rattanapak, T., Birchall, J. C., Young, K., Kubo, A., Fujimori, S., Ishii, M., & Hook, S. (2014). Dynamic visualization of dendritic cell-antigen interactions in the skin following transcutaneous immunization. PloS One, 9(2), e89503. doi:10.1371/journal.pone.0089503
Rollins-Smith, L. A., Reinert, L. K., O'Leary, C. J., Houston, L. E., & Woodhams, D. C. (2005). Antimicrobial Peptide Defenses in Amphibian Skin1. Integrative and Comparative Biology, 45(1), 137-142. doi:10.1093/icb/45.1.137
Sköld, H. N., Aspengren, S., & Wallin, M. (2013). Rapid color change in fish and amphibians – function, regulation, and emerging applications. Pigment Cell Melanoma Res, 26(1), 29-38. doi:10.1111/pcmr.12040
Sugden, D., Davidson, K., Hough, K. A., & Teh, M.-T. (2004). Melatonin, Melatonin Receptors and Melanophores: A Moving Story. Pigment Cell Research, 17(5), 454-460. doi:https://doi.org/10.1111/j.1600-0749.2004.00185.x
Vaudry, H., Chartrel, N., Desrues, L., Galas, L., Kikuyama, S., Mor, A., . . . Tonon, M. C. (1999). The pituitary-skin connection in amphibians. Reciprocal regulation of melanotrope cells and dermal melanocytes. Annals of the New York Academy of Sciences, 885, 41-56. doi:10.1111/j.1749-6632.1999.tb08664.x
Wanninger, M., Schwaha, T., & Heiss, E. (2018). Form and Function of the skin glands in the Himalayan newt Tylototriton verrucosus. Zoological Letters, 4(1), 15. doi:10.1186/s40851-018-0095-x
Weitzman, S. H. (2018, 15 Nov 2018). Teleost. Retrieved from https://www.britannica.com/animal/teleost
Xu, X., & Lai, R. (2015). The Chemistry and Biological Activities of Peptides from Amphibian Skin Secretions. Chemical Reviews, 115(4), 1760-1846. doi:10.1021/cr4006704
Yousef, H., Alhajj, M., & Sharma, S. (2020). Anatomy, Skin (Integument), Epidermis. In StatPearls [Internet]. Retrieved from https://www.ncbi.nlm.nih.gov/books/NBK470464/
Zelickson, A. S. (1965). THE LANGERHANS CELL. Journal of Investigative Dermatology, 44, 201-212. doi:10.1038/jid.1965.35