A Chemical Perspective on the Carapace
Elisabeth Lawton, Emma Geoffroy, Osandi Hewage, Yitao Sun
Abstract
The carapace’s chemical properties are of interest in a lot of scientific research since they provide insight into an organisms’ life cycles and ecological niche. This article presents a few chemical perspectives on carapace, including their evolution, calcification, pigment production, and composition. In the evolution section, the chemical processes involved to harden the turtle’s carapace throughout its evolution is discussed. The calcification section explores the carapace’s structure and the chemical reactions involved in the molt cycle of blue crabs. This paper’s pigment production section explores the astaxanthin and crustacyanin in lobsters; the camouflage of crustaceans; and the fluorescence in scorpions. In the carapace composition section, the multi-scale complex composition of the carapace with collagenous and keratinous layers that harden the turtle’s shell are described.
Introduction
The carapace has been the primary subject of interest for numerous researchers as it varies significantly between different organisms, not only in terms of chemical composition, but also with regard to structure and biological function. With the fusion of vertebrae and other dermal elements, carapace formation may result from an integrated ribcage, as seen in turtles, or the mineral deposition and calcification of the exoskeleton, as evident in crustaceans.
In addition to providing an understanding of the life cycle and ecological niche of organisms, research about the carapace has provided scientists with insight about the social advantages and the key role it plays in ensuring their survival. For instance, the keratinized layers of the turtle carapace contribute to its structural integrity and rigidity. In contrast, the carapace in lobsters and crabs only serves as a protective cover. The soft sheath can be easily shed during the molting process and is subsequently reformed with calcification. Alternatively, the carapace also has photobiological and physiological functions. From fluorescence in scorpions to camouflage abilities in lobsters, the carapace has been used in numerous studies to devise engineering applications and implement conservation strategies.
Carapace formation and composition
Turtle carapace formation – an evolutionary perspective
The carapace is a dorsal structure primarily known for its protective function. However, it does not only serve this purpose. Taking a look at turtles, their carapace also serves as the main mineral reservoir in the body. Specifically, “over 99% of the total body calcium, magnesium, and phosphate, over 95% of the carbon dioxide, and over 60% of the body’s sodium resides in the shell and bone” (Jackson, 2000). The carapace is also involved in regulating the pH by lactic acid buffering. This process is important since the accumulation and production of lactic acid is dangerous for the turtle. Even though the production rate is small, its accumulation over time in the body can result in metabolic acidosis. The regulating process is done by first releasing carbonate buffers from the bone into the extracellular fluid. Then, the lactic acid will enter and remain isolated in the bone. This buffering process allows turtles to remain underwater without oxygen for at least three months. It is important to note, however, that this occurs at 3°C and under severe metabolic depression (Jackson, 2000).
Taking a more evolutionary look into turtles, it has been found that the carapace initially evolved to provide structural support for burrowing. In fact, it is the turtle’s broad ribs that extend, in a dorsolateral way, into the dermis–their skin–that allow them to have an increased stability. This results in the ability to support their trunks while digging. However, this feature also reduces their mobility (Schoch et al., 2019). Figure 1 shows the general evolution of the turtle carapace from three different stem-turtles’ fossils dating back to the Triassic era. Both (A) Pappochelys rosinae and (B) Odontochelys semitestacea do not form complete carapaces due to their broad, T-shaped ribs and their lack of secondary metaplastic ossifications. However, (C) Proganochelys quenstedti has sutured ribs as well as metaplastic ossifications that result in the formation of a complete carapace.

Fig. 1. Aerial view of stem-turtles carapaces where (A) is Pappochelys rosinae, (B) is Odontochelys semitestacea, and (C) is Proganochelys quenstedti. The black coloring in (A) and (B) corresponds to the plastron as to differentiate it from the carapace of stem-turtles [Adapted from Schoch et al., 2019].
The formation of the turtle carapace is a multi-step process that involves the growth of ribs within the dermis, and the ossification of the carapace. Firstly, in turtle embryos, the carapacial ridge—the region responsible for the formation of the outer edge of the carapace—will be formed by the thickening of the ectoderm, which is a layer of cells found in embryos. The development of this structure is the first indication of carapace formation. The carapacial ridge is composed of both an epithelial layer, consisting of specialized cells that serve as structural lining, and a mesenchyme layer, consisting of unspecialized connective tissue cells derived from the mesoderm. It is important to note however that the mesenchyme does not refer to mesenchymal stem cells that are found in bone marrow. Therefore, the interaction between these two layers allows structures in embryos to form (Gilbert et al., 2008). Next, rib cells, which are made from the mesenchyme, will enter the carapacial ridge and then grow laterally in the forming carapace. This, therefore, results in the initiation of the ossification process (Gilbert et al., 2001).
The process of bone formation of the turtle carapace starts with endochondral ossification of costal bones. Costal bones make up the scutes of the carapace (Fig. 2). This ossifying process includes bone cells replacing the rib cartilage found in the dermis. The formation starts from the proximal part of the ribs, near the back side of the body. However, the distal parts of the ribs, near the ventral side of the body, remain in their cartilaginous form and are only ossified later (Gilbert et al., 2008). This process can be seen in Figure 3 (A-D). Once the ribs are in the process of ossification, the costal bones begin forming around the ribs using intramembranous ossification, as shown in Figure 3 (E-H) (Gilbert et al., 2001). It has been found that during the process of bone formation, bone morphogenetic proteins (BMP) are secreted and can promote the intramembranous ossification of the dermis. This happens through BMP signaling between the ribs and dermal cells. However, BMP signaling is also used as dermal cells ossify, thus further allowing more cells to go through this process. This results in the bone formation of the costal scutes of the turtle carapace (Gilbert et al., 2008).

Fig. 2. Ventral view (A) and bone structure pattern (B) of the Chelydra turtle carapace [Adapted from Gilbert et al., 2001].
Lastly, there is the ossification of the nuchal and peripheral bones. The nuchal bone formation, which is for the bones near the turtle’s neck, occurs using primary and secondary dermal ossification. The primary phase corresponds to forming a thin band of condensed cells in the dermis that can expand laterally. There is also calcium deposition that occurs along the first scute. As Gilbert et al. (2001) found, the second phase corresponds to the ossification of the nuchal plate by forming a structure of trabecular bone, which is a type of bone composed of non-solid and porous tissue. This lattice-like structure will then expand into the dermis and will surround the condensed cells formed from the primary phase. Unlike the primary phase, the secondary phase will not only solidify the carapace in the embryo, but additionally, throughout the turtle’s life (Gilbert et al., 2001). This process can also be seen in Figure 3 (E-H).

Fig. 3. Development of the turtle carapace in embryos. (A-D) shows the endochondral ossification of the ribs occurring at different days in development. (A) is a turtle embryo at 25 days, (B) is at 36 days, and (C, D) at 45 days. (E-H) shows the dermal bone formation where intramembranous ossification occurs. (E, F) shows a turtle embryo at 90 days, (G) is at 118 days and (H) is at 185 days [Adapted from Gilbert et al., 2001].
Chemical composition of the turtle carapace
The turtle shell is known to be a remarkable armor specifically designed to protect the turtle from various types of high-stress events (Achrai et al., 2017). The turtle carapace is the top piece forming the dorsal component of the turtle’s shell (Achrai et al., 2017). To understand how this exceptional structure was able to evolve, there are many components to consider. Among the many regions of the turtle carapace analyzed, the exact composition of the carapace is of specific interest in the field of biological materials. This is because the carapace has a multi-scale complex composition (Fig. 4) (Achrai et al., 2017).


Fig. 4. a) The structural hierarchy of bone b) Ventral view of a turtle carapace specimen with an individual rib marked by a white arrow and a suture marked by a yellow arrow c) A specimen of a single section of the rib enclosed with sutures at each edge d) A tomographic reconstruction of the specimen in c). The dorsal cortex, ventral cortex, and cancellous interior are all labelled with DC, VC, and CI, respectively. e) The suture region represented by an ortho-slice view f) Scanning electron microscopy of the cross-section of the suture region (perisuture) g) High-resolution micro-computer tomography of the section in the red box from image f) [Adapted from (Achrai et al., 2017)].
The composition of the carapace begins with type I collagen helices, hydroxyapatite nano-platelets and water. Together these three components form mineralized collagen fibrils which are common to all bones. Mineralized collagen fibrils assemble into thicker fibrils, which then form bundles with various structures such as parallel-fibered, woven, radial, and plywood arrays. While all four arrays are common to the carapace, the plywood pattern is the most common. The plywood array is found in concentric lamellae which form the osteonal unit (Achrai et al., 2017).
The bony carapace is covered by a keratinous layer which can be divided into two layers; the stratum corneum which is the outer layer and a keratin matrix forming the lower layer. The outer layer is composed of β-pleated sheets with few α-helices resulting in a rigid and hard structure. The lower keratin matrix layer is reinforced with fibril which embed keratinocytes, melanocytes, pigments, and lipids (Achrai et al., 2017).
Keratin is a specific kind of protein, specifically an intermediate filament forming protein (Wang et al., 2016). Keratins are classified as having α-keratin and β-keratin (Lawrence, E.M., 2014). Α-keratin, involves a structure of amino acids which form a right-handed α-helix secondary protein structure stabilized by hydrogen bonds. Alanine and glycine (Fig. 5) are the primary amino acids which form keratins, but up to 20 amino acids can compose keratins. Cystine and Serine are other common amino acids which compose keratin fibers (Fig. 5) (Wang et al., 2016).

Fig. 5 Principal amino acids composing keratin-based protein fibers [Adapted from Lawrence, E.M., 2014].
In nature, a typical model of mechanical protective structures consists of a multilayer structure where the exterior is harder and stiffer than the interior backing plates which are more compliant. So, any impact hits the harder exterior first (Achrai et al., 2017). The goal of this design is that the projectile deforms upon contact with the hard outer layer and the kinetic energy it carries is absorbed by the interior more compliant layer. The turtle carapace follows a different model with the opposite design. The turtle’s carapace consists of a thin keratin coating on the exterior followed by stiff and brittle dorsal bone underneath. It is believed that this design is still effective because the ductile and tough keratin exterior spreads the applied force. In fact, a study has been performed on turtle carapace specimens to test the result of the keratin coating. This was done by comparing carapace specimens with keratin and without keratin when a low velocity impact was applied. The results demonstrated that specimens with the keratin coating absorbed three times more energy. Furthermore, the keratinous specimen was able to better maintain its structural integrity. After impact, 95% of the specimens without keratin were completely split whereas, approximately 50% of the specimens with keratin were split. Additionally, the exact placement of the keratin layer was tested and when the keratin layer is on the opposite side of the impact site, total breakage occurs. This suggests that the exact positioning of the keratin layer with respect to the impact plays an important role in preserving the structural integrity of the carapace. Additionally, another toughening mechanism of the keratin sheet is tension induced α-helix to β-sheet transition in the keratin nano-fibrils. This transition mechanism lengthens the keratinous materials by breaking hydrogen bonds in the α-helices and reforming the hydrogen bonds to form β-sheet structures (Achrai et al., 2015).
The keratin coating is connected to the underneath bone through a middle layer called the dermis layer. The collagenous dermis spans from one suture to another attaching the keratinous epidermis to the dorsal bone. The keratin scutes are composed of many layers where each sublayer is a fiber matrix sheet. These layers come together and form a keratinous matrix with the support of nanofibrils. Most of these nanofibrils are β-pleated sheets with few α-helices in the inner layers of the matrix. Energy dispersive spectrometry demonstrated a high amount of sulfur and a slightly higher amount of calcium when compared to the surrounding bone. Cross-linking disulfide bonds of cysteine residues are known to add to the stiffness and hardness of the keratin scutes. Therefore, this additional sulfur quantity may be attributed to these disulfide bonds. The small addition of calcium may be related to the dermis layer which connects the bone to the keratinous sheets (Achrai et al., 2017).
The outer keratin coating has inspired some designs contrary to the standard design of having a more rigid exterior and a softer interior to absorb impact. For example, in military vehicles, a soft elastomeric polyurea coating covers a stiff interior. The reasoning behind this counterintuitive idea is that the soft coating breaks up the compressive shock wave produced by the impacting projectile (Achrai et al., 2015). Laminated glass, commonly used in windshields, shares a similar sandwich composite structure as the turtle carapace. However, the difference lies in a rigid layer coating the exterior of the laminated glass instead of the flexible external keratin coating in the turtle carapace(Achrai et al., 2015). The outer keratin coating may inspire future designs of laminated self-healing glass where a flexible coating is used on the exterior of laminated glass in place of the rigid layer (Achrai et al., 2015).
Armadillo carapace composition
The armadillo shares many similarities when compared with the turtle carapace (Wang et al., 2016). A chemical analysis was performed on a variety of specimens from the nine banded armadillo carapace, specifically the forward shell (Fig. 6). The outermost layer of the carapace mainly consists of carbon, oxygen, and sulfur. This makes sense when considering the composition of the keratin protein. The top and side surfaces of the nine banded armadillo have slightly different chemical composition compared to the superficial keratin layer. The key difference is the presence of calcium, phosphorus, sodium, and magnesium. Together these molecules can form calcium phosphate which is a main component of bone when in the specific structure known as hydroxyapatite. This chemical analysis of the nine banded armadillo shell confirms the composite layered structure of the armadillo shell (Chen et al., 2011).

Fig. 6 Energy dispersive x-ray analysis results obtained from different phases of the armadillo’s forward shell (Rhee et al., 2011). (a) Outside keratin layer. (b) Top surface. (c) Front surface. (d) Seam region in between scutes.
Blue crab molting
A blue crab, Callinectes sapidus, must shed its exoskeleton to expose a new, larger exoskeleton to grow. Smaller crabs shed four or five times per month, while older crabs may need thirty to fifty days to grow large enough before shedding again. Every time a blue crab molts, the old shell is abandoned, and the new shell will cover the body (Fig. 7). The new shell will remain until the crab needs to shed again to allow a bigger cuticle to cover its growing body. This periodic phenomenon is called the molt cycle. A blue crab typically has aroundt 25 molt cycles in a lifetime.

Fig. 7. A blue crab is molting. The old cuticle is on top of the new cuticle of the crab [Adapted from Horizons, 2022)].
Blue crab’s carapace structure
The structure of a blue crab’s carapace consists of a rigid cuticle and the soft cellular hypodermis underneath, with vertical pore canals piercing through the cuticle. The cuticle is an exoskeleton composed of three layers, from outside to inside: epicuticle, exocuticle, and endocuticle (Fig. 8). The hypodermis is arranged in three layers of cells, the outer epithelium, the sub-epithelial connective tissue, and the inner epithelium, where the outer epithelium is the most involved in the molt cycle. (Roer & Dillaman, 2018).
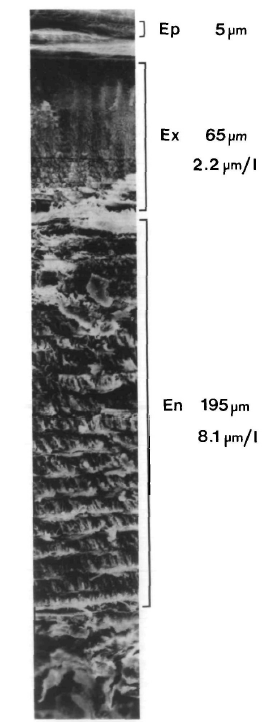
Fig. 8. A SEM (scanning electron microscope) fractography of the mineralized cuticular layers of the carapace En = endocuticle, Ex = exocuticle, Ep = epicuticle. Numbers represent the total thickness of the individual layers [Adapted from Roer & Dillaman, 1984].
The epicuticle is the most outside layer of the cuticle and is also the thinnest. It consists mainly of calcium salts. It is also the hardest part of the crab’s carapace. The exocuticle immediately lies under the epicuticle, and is composed of chitin-protein fibrils in the Bouligand structure.
The chitin-protein fibrils (Fig. 9) are commonly found in crustaceans. Chitin is a polysaccharide composed of β-l,4-linked N-acetylglucosamine. Chitin is cross-linked with protein, which consists of amino acids to form chitin-protein fibrils, via the hydrogen bonds between hydroxyl groups and amide groups.
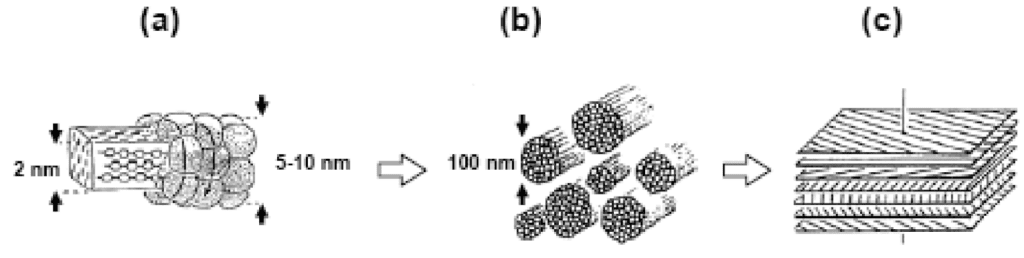
Fig. 9. Schematic of the chitin-protein matrix in crustacean cuticles: (a) Chitin crystals surrounded by proteins, (b) Chitin-protein fibrils, (c) Schematic representation of fibrils lying in the Bouligand structure (Einbu, 2007).
These fibrils are arranged in the Bouligand structure, a structure characterized by the stack of multiple layers with slight rotation between each adjacent layer. The Bouligand structure provides fracture resistance and enables strength through crack deflection. Deflection can take the form of crack tilting, and crack bridging. In crack tilting, the crack propagates along the direction of the fibrils. When the energy release rate at the tip is sufficiently low, the crack can no longer propagate along the fibril direction and must switch to crack bridging. The crack bridging mode involves the crack changing direction drastically and cutting through fibrils to reach a new direction to propagate along. A combination of crack tilting and crack bridging in the Bouligand structure results in a highly distorted and enlarged crack. This causes the new surface area created by the propagating crack to increase dramatically relative to a straight crack. This dispersion of cracks makes further straight-line propagation less favorable than smaller propagation in a smaller area , which in turn toughens the material.
Besides the Bouligand structure, the exocuticle is also hardened by quinone tanning and the calcification of mineral crystals between the chitin-protein fibers (Travis, 1955). The quinone tanning is the chemical crosslinking of proteins by quinones through the binding between amino acids from the protein and the carbonyl group on the quinone. Quinone tanning is enhanced by exposure to u.v. light according to Schlein (Schlein, 1975).
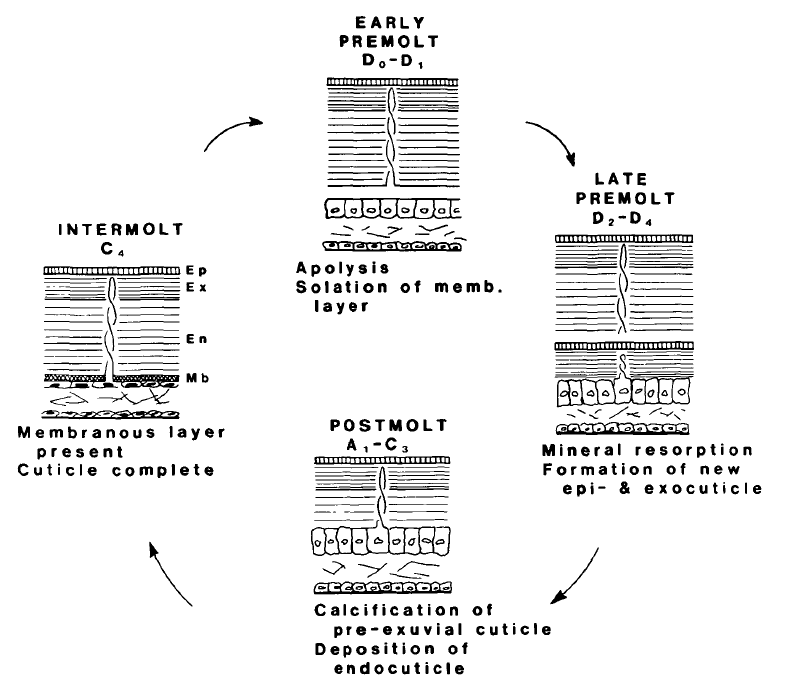
Fig. 10. Some common quinones. From left to right, 1,2-benzoquinone, 1,4-benzoquinone, 1,4-naphthoquinone, 9,10-anthraquinone [Drawn using ChemDraw Professional 21.0.0].
The endocuticle is the thickest layer of the cuticle and also the most heavily calcified one. The endocuticle is also composed of chitin-protein fibrils with a Bouligand structure, just like the exocuticle. However, one difference is that there is no evidence of quinone tanning in the endocuticle, and the calcification of mineral crystals is the only factor that solidifies the endocuticle. All three layers of the cuticle contain calcium carbonate (CaCO3), in the form of calcite or amorphous calcium carbonate. During the molt cycle, soft amorphous calcium carbonate will accumulate to form rigid calcium calcite. This process is called calcification.
Unlike the cuticle, the hypodermis that lies under the endocuticle consists of three layers of cells. The outer epithelial layer is one cell thick, with the cells varying in size during different stages of the molt cycle. The epithelial cells are enlarged to provide extra protection during the shedding process since the cell can absorb more kinetic energy upon contact when being large. The other two layers of the hypodermis, the sub-epithelial connective tissue layer and the inner epithelium, are not involved in the molt cycle but provide stability to the whole structure.
The pore canals are vertically arranged over the whole cuticle. Researchers have shown that the canals are the cytoplasmic extensions of the outer epithelial cells. The pore canals start from the outer epithelial cells border and end at the epicuticle (Hegdahl et al., 1978). These pore canals play important roles during the molt cycle.
Molt cycle
The molt cycle is divided into five stages from A to E, in which the postmolt period is stages A1, A2, B1, B2, C1, C2, and C3; intermolt is stage C4; premolt is stages D0, D1, D2, D3, and D4; and the ecdysis or molting is stage E (Fig. 11.).
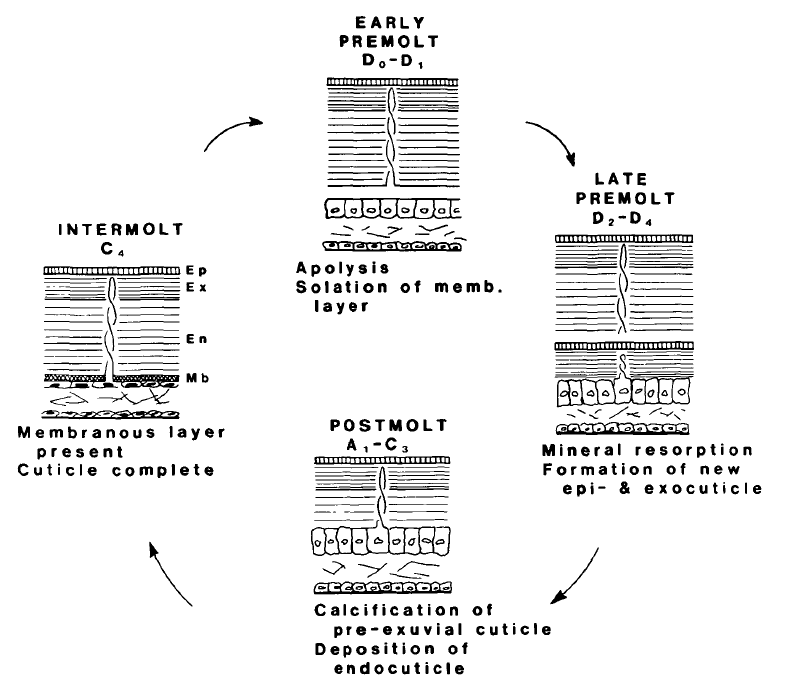
Fig. 11. The molt cycle in blue crab. The ecdysis which is not shown in this diagram is between the late premolt and the postmolt stage. The molting process is: ecdysis, postmolt, intermolt, premolt, and back to ecdysis (Roer & Dillaman, 1984).
The intermolt phase (stage C4)
An adult blue crab spends most of its life in the intermolt phase and only molts once or twice every year (Passano, 1960). During stage E, the three layers of the cuticle are completely calcified and the hypodermis, which is the cellular layer, is maximally reduced in size. The epithelial cells that are in direct contact with the endocuticle, become squamous and squeezed to only 9 µm. Moreover, the C4 phase is also characterized by a high level of RNA synthesis leading to the production of connective tissue cells to prepare for the premolt period.
The premolt phase (stage D0 – D4)
The first step of the premolt phase is apolysis, which is the separation of the hypodermis from the cuticle. The apolysis is caused by the secretion of chitinase and protease. Chitinase is an enzyme that breaks down chitin. Protease is an enzyme that catalyzes proteolysis, which is the process to degrade proteins into smaller polypeptides or single amino acids. In this case, the fibers in the membranous layer are broken down. Besides the isolation of the hypodermis, the apolysis also leads to the size increase of outer epithelial cells. The cell becomes columnar, and the height can reach about 56 µm by D3.
During the premolt phase, the new epicuticle and the new exocuticle are formed beneath the old cuticle. Specifically, the new epicuticle is deposited during the D1 stage and the new exocuticle is deposited during the D2 stage. Since the two new cuticle layers are under the old rigid cuticle, they must be soft. Thus, the new epicuticle and the new exocuticle are not yet calcified during the premolt phase and are composed of amorphous calcium carbonate. The amorphous calcium carbonate is the least stable polymorph of calcium carbonate and expresses the least rigidity. Also, it is noted that the new endocuticle is not deposited until the postmolt phase (stage B)
Another phenomenon that happens during the premolt phase is the resorption of the mineral and organic compounds of the old cuticle. It is observed that about 15 – 20% of the minerals from the old cuticle are resorbed during premolt (Travis, 1955). This resorption can be experimentally observed through the pH of hemolymph, analogous to the blood in vertebrates. The reason is during the resorption, the calcium carbonate is decomposed to Ca+ and HCO3- ions, of which the concentration increases and thus leads to a rise in pH value. The rise in pH value reaches its maximum in the D2 stage, from 7.9 to 8.1 (Travis, 1955).
The ecdysis (stage E)
The ecdysis or molting (stage E) is the process during which the blue crab takes off the old cuticle and exposes the newly formed epicuticle and exocuticle into the environment. The u.v. light will then enhance the quinone tanning. The entire process of the ecdysis only lasts for 2 hours in most cases (Roer & Dillaman, 1984).
The postmolt phase (stage A1, A2, B1, B2, C1, C2, C3)
The postmolt phase, from stage A to stage C, is the calcification of the new cuticle. The initial event is quinone tanning during stage A. The quinone is formed from dihydroxyphenol, under the enzyme called polyphenol oxidase. Polyphenol is a chemical compound composed of multiple phenol units. Polyphenol oxidase is a common enzyme in nature that catalyzes the oxidation of phenols. The product of this reaction is benzoquinone, which ensures the quinone tanning of the exocuticle (Fig. 12.). Both the dihydroxyphenol and the enzyme are transported to the cuticle from the epithelial cells via the pore canals. Since the compounds are transported through the pore canals, the lower layer, the exocuticle, is first calcified followed by the upper layer, the epicuticle.
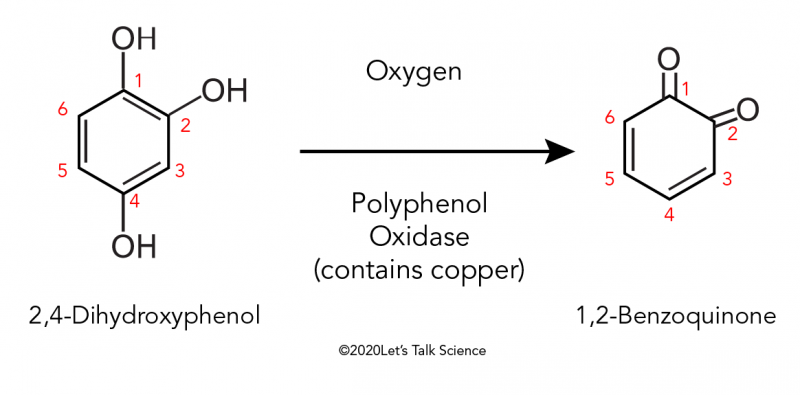
Fig. 12. Reaction scheme of the oxidation of 2,4-dihydroxyphenol which produces 1,2-Benzoquinone (Why Do Apples Turn Brown After You Cut Them?, 2019).
Stage B is marked by endocuticle deposition. It was mentioned early that the endocuticle is not deposited during the premolt phase (stage D) together with the epi- and exocuticles, but only starts to form during stage B. Here, the deposition of the endocuticle occurs simultaneously with its calcification.
Another feature of the postmolt phase is the outer epithelial cells in the hypodermis becoming small and squamous again. The size variation signifies the end of the molt cycle and occurs usually two days after the ecdysis (Roer & Dillaman, 1984).
Pigmentation of carapaces
Pigment production in lobster carapaces
In an attempt to evade any predators, many organisms rely on their ability to camouflage to mask their identity and location. Crustaceans possess an array of pigments in their carapace, which are speculated to play a significant role in photobiological functions (Zagalsky, 1982), such as light sensitivity, as well as other physiological processes, including carapace molting, hormone regulation and camouflage. Due to limited research, scientists have developed a keen interest in the photochemistry involved in pigment production in lobsters. While lobsters generally appear to have dark coloration, there is a distinct coloration difference between shallow and deep-water lobsters, the former having blue-tinged appearances and the latter having yellow/calico hues. This difference in coloration depends solely on the amount and distribution of yellow and blue pigmented protein complexes, containing astaxanthin, a keto-carotenoid.
Analysis of pigments present in carapace using resonance Raman spectra
Commonly found in plants and marine organisms, carotenoids are hydrophobic molecules that function as primary pigments. These typically combine to form protein complexes (carotenoproteins) such as astaxanthin and crustacyanin, which are responsible for the carapace color in the H. americanus lobster. Several studies, including the ‘Resonance Raman’ experiments (Salares et al., 1977) on lobsters, provide information about pigment distribution in the carapace along with the energy-transfer capability of excited electrons and the role of pigments in light harvesting systems. The absorption spectrum of astaxanthin aggregate is characterized by an intense absorption peak at roughly 600 nm, whereas the resonance Raman spectra of α, β and γ-crustacyanin (Fig. 13.) exhibit intense absorption peaks at 1500-, 1150 and 1000 cm-1. The data proves that the blue lobster shell contains α-crustacyanin and other carotenoprotein complexes.

Fig. 13. Resonance Raman spectra with 647.1 nm excitation of crustacyanin in (a) 0.1M phosphate, pH 7 and (b) blue lobster shell [Adapted from Salares et al. 1977].
Possible photobiological function of yellow protein
The degree of absorption at shorter wavelengths decreases with respect to the resonance Raman spectra of yellow-proteins at 25°C and -90°C and vice versa. A small frequency change is also observed however this is reproducible and reversible upon altering the temperature.
In comparison with the absorption spectra (Fig. 14.) of yellow proteins, temperature has a negligible effect, except for a minor red shift of 6 nm. As the transmitted light at great depths underwater is mainly blue, which corresponds to the same spectral region as yellow protein absorption, lobsters found at such depths typically have a yellow carapace, whereas shallow-water lobsters appear blue.

Fig. 14. Absorption spectra of the yellow protein in 0.1M glycerol phosphate (pH 7) (1:1 Volume) at (a) 25°C and (b) -90°C (Salares et al. 1977).
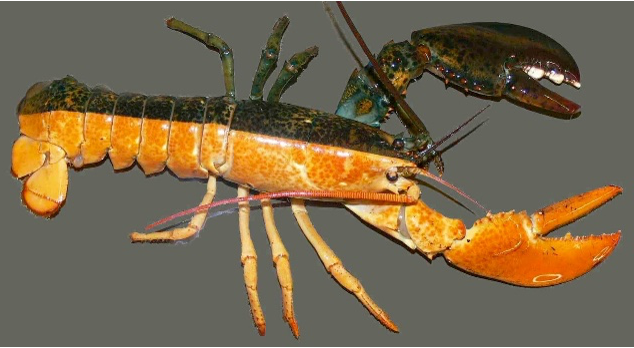
Fig. 15. Gynandromorph lobster, yellow male on bottom half and green-brown female on top half (Courtesy of A.R. Palmer, 2008).

Fig. 16. Representation of the genitalia of a hermaphroditic lobster, with male and female swimmerets (Courtesy of Marine Science Center of Northeastern University, 2012).
The large separation in wavelength between the yellow protein (400 nm) and the crustacyanin (600 nm) allows for selective resonance (Salares et al., 1977). Although blue coloration may not be visible in yellow carapaces, the Raman spectra (Fig. 17.) indicates the presence of blue crustacyanin. Hence, this study suggests that both yellow and blue proteins are present in different colored shells to varying extents. A blue lobster is typically seen as such because it is either transporting all the pigments into that layer or because it has an abundance of proteins in that layer, thus making the second layer the most dominant color. Having conducted further experiments, scientists inferred that the detection of the yellow protein spectrum from a blue pigmented shell suggests that the yellow protein is present in high concentrations in the outermost layers/surface of the carapace. Although such theories are circumstantial due to a lack of extensive research, protein pigments are said to provide dermal light sensitivity in crustaceans, which is used to stimulate hormonal activity which, in turn, leads to seasonal migration, carapace molting, etc.

Fig. 17. Raman spectra in the C=C stretching region of (a) yellow shell with 441.6 nm excitation, (b) yellow shell with 647.1 nm excitation and (c) blue shell with 647.1 nm excitation (Salares et al. 1977).
Phenotypic coloration of lobster carapace
According to the University of Maine’s Lobster Institute, lobsters have three layers of colors: yellow (outermost layer), blue and red (innermost layer). Depending on the frequency of light absorbed and transmitted by each of the pigments/proteins present, live lobsters are brown and mottled, as seen by the human eye. However, when cooked, as proteins begin to denature and astaxanthin is released from its former protein-bound state, lobsters change color from blue to vivid red. Further studies (Tlusty & Hyland, 2005) were carried out to investigate the effect of genetic and dietary mechanisms on pigment production in American lobsters. Carotenoprotein formation was assessed in lobsters rendered white by a diet low in astaxanthin, by feeding them different concentrations of the protein over a period of 110 days. The degree of redness/blueness in their appearance was used to qualitatively determine the ratio of proteins present in the carapace. When combined with crustacyanin, astaxanthin, which is typically red, undergoes a bathochromic shift (absorption of longer wavelengths/lower frequency), thereby producing purple, blue and yellow hues, in α, β and γ-crustacyanin, respectively. As a result, the extent of colorization also provided insight into the ratio of free and protein-bound astaxanthin and their relative concentrations in various parts of the body. Although lobsters that were fed an excess of astaxanthin were predominantly red, they began to display gradual changes in color. Such variation occurs due to differences in the uptake of astaxanthin or the rate of carotenoprotein complexing. The results of these experiments showed that the phenotypic coloration of lobsters was solely due to diet (Fig. 18.). Other mottled appearances of lobsters were caused by the uneven distribution of protein complexes and the interference of thick cuticles which masked the color.

Fig. 18. Juvenile Homarus americanus lobster fed an astaxanthin-deficient diet exhibits minimal pigmentation (bottom) compared to mature lobster fed a normal diet containing sufficient astaxanthin (top). Note that the difference in size is only due to age and not diet (Courtesy of Dr. Tlusty, 2005).
While astaxanthin deficiency is responsible for the white translucent appearance of lobsters, this is not always the case. Leucism is a result of a genetic mutation that inhibits the production of melanin in specific bodily regions and is characterized by reduced or randomized pigmentation. This is not to be confused with albinism, for which partial loss of pigmentation does not exist. Lobsters with leucism (Fig. 19.) have slightly different traits, such as iridescent carapace and colored eyes.
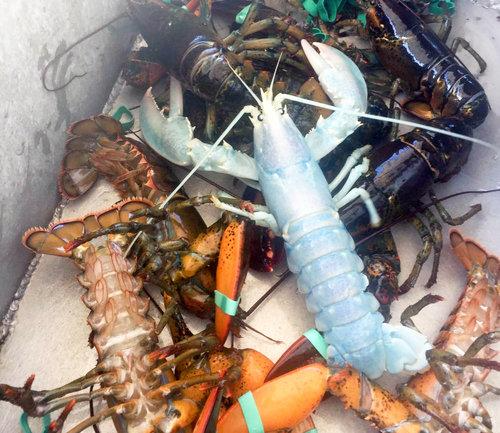
Fig. 19. Image of iridescent blue lobster (center) suffering from leucism (partial loss in pigmentation). (Courtesy of Alex Todd, 2017)
Decoration of carapace to aid camouflage
Similar to lobsters, other crustaceans, such as crabs exhibit variation in carapace color. Certain species, such as spider crabs of the Majoidea family (Fig. 20) and sponge crabs of the Dromiidae family (Fig. 21), can camouflage themselves and escape predators. In addition to decorating their carapace with colors that resemble the background/surrounding environment, crabs and lobsters alike can also enlarge themselves in terms of size. Since crustaceans typically shed their carapace during the molting process, they don a larger, more threatening carapace after renewal/recalcification. Not only does this allow them to avoid detection, but it also allows them to respond to spatial and temporal changes in the environment (Mynott et al., 2018). While studying the role of pigment production in camouflage is useful in terms of understanding interspecific interaction, it is also an effective tool to support the conservation of species. As the breeding stocks of lobsters and crabs are diminishing due to overfishing, captive breeding and stocking programs can be implemented, using this research, to improve prior techniques. For instance, knowing whether juvenile lobsters can camouflage to match the habitat allows scientists to determine the most appropriate release site to ensure their survival. An experiment exposing juvenile lobsters to white and black backgrounds showed that there is no change in coloration for short-term exposure. This explains the increased mortality of lobsters upon release into the wild. Alternatively, lobsters that were exposed to dark environments for prolonged periods exhibit gradual changes in color, thus reflecting an ontogenetic change (development during juvenescence). This concludes that pigment production plays a crucial role in the physiological functions of many organisms, including crustaceans. Conducting extensive studies to understand the processes and mechanisms involved will be beneficial in terms of ensuring their survival.
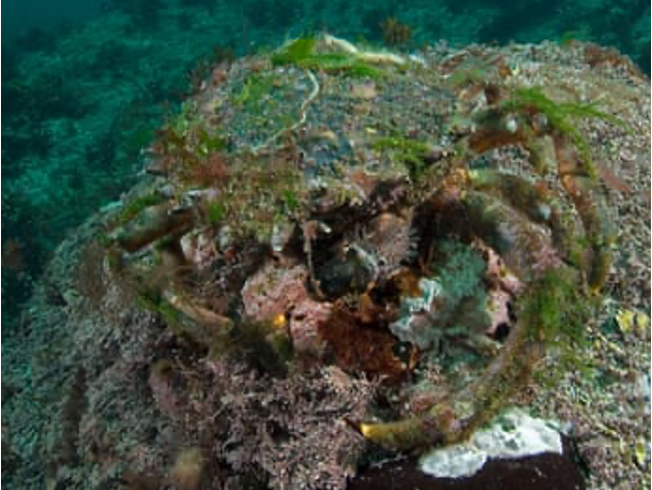
Fig. 20. Photograph of well-disguised spiny spider crab (Courtesy of Paul Naylor)

Fig. 21. Image of bristled sponge crab wearing a sponge disguise over the carapace. The sponge emits noxious chemicals to ward off predators (Courtesy of Fred Bavendam).
Fluorescence in scorpions
In 1954, it was discovered that the majority of scorpions exhibit fluorescence. Fluorescence light emission, by definition, occurs when some compound absorbs a photon resulting in electrons reaching an excited state. Almost immediately after being excited, the electrons will return to their original energy state therefore emitting a photon of visible light (Volschenk, 2005). In the case of scorpions, this happens when exposed to ultraviolet radiation. It is their epicutile, a thin layer covering their exoskeleton, that exhibits this property of emitting a blue-green color (López-Cabrera et al., 2020).
Various factors in the epicuticle can contribute to the fluorescence intensity in scorpions. Firstly, there are chemical compounds, called fluorophores, that are responsible for such phenomena. Specifically, β-carboline and 4-methyl-7-hydroxycoumarin. These molecules are involved in the sclerotization process of the carapace, which is “the process of hardening and tanning of the chitin” (López-Cabrera et al., 2020). However, the direct cause and effect relationships between fluorophores and fluorescence intensity remain unclear as fluorescence intensity may additionally be impacted by high absorption of radiation by melanin and other pigment molecules (López-Cabrera et al., 2020). It has further been found that the thickness of the epicuticle can increase the fluorescent emission. This is the result of a study on scorpion carapace molting. In fact, after molting, scorpions remain unable to emit fluorescence for 48 hours as the fluorophores remain in the exoskeleton that is removed (Li et al., 2022). This can be seen in Figure 22 where the number 1 is 8 days before moulting, number 2 is 2 days before moulting, numbers 3-7 represent the moulting process, and numbers 8-13 respectively starting from 12 hours up to 4 days after the molting process is complete. Lastly, it has been hypothesized that fluorophores are brought into the carapace by the epicuticle’s impermeable property that similarly allows the transport of polysaccharides and lipids into the exoskeleton. This may thus further impact the fluorescence intensity by increasing the concentration of fluorophores in the carapace (López-Cabrera et al., 2020).
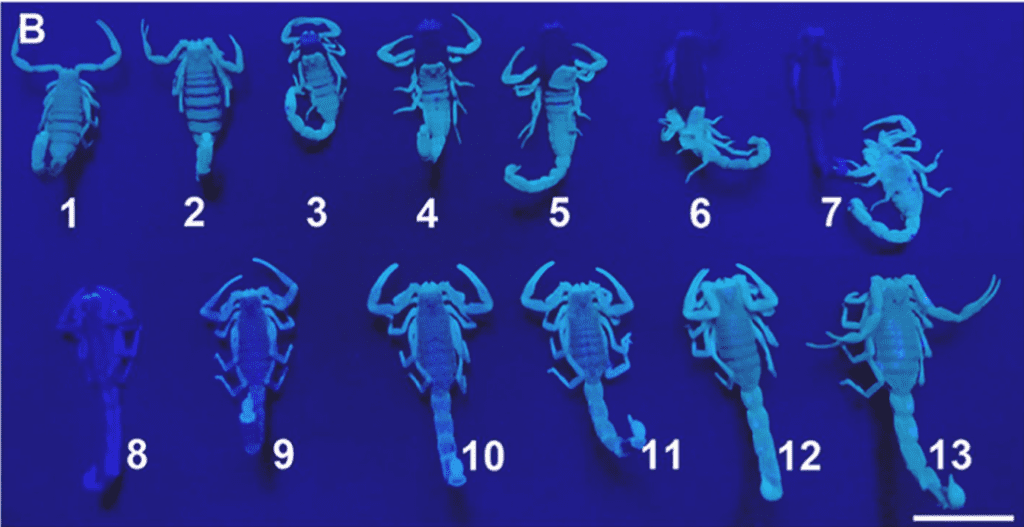
Fig. 22 Third molting process of the scorpion M. Martensi under ultraviolet radiation, 1; 8 days before moulting, 2; 2 days before moulting, 3-7; moulting process, 8-13; 12h, 18h, 24h, 32h, 40h, 4 days after moulting, respectively; Scale bar: 1 cm. [Adapted from Li et al., 2022].
However, fluorescence in scorpions is not only impacted by their exoskeleton epicuticle, but also by their carapaces’ color tonality. In a study performed by López-Cabrera et al. (2020), they tested the correlation between various scorpions’ color and their fluorescence by calculating the reflected light per unit area when white light shines on them. It was found that scorpions with a lighter complexion have a higher light reflectance compared to darker colored scorpions. They further tested, with the use of ultraviolet radiation, the intensity of fluorescence across the same different species. With the results from both, López-Cabrera et al. (2020) found a linear relationship between the intensity of reflected white light and the intensity of fluorescence. As can be observed in Figure 23, the lighter the scorpion carapace color tone, the higher the reflected white light intensity it has, and therefore the greater the fluorescence intensity is.

Fig. 23. Dispersion graph showing a linear relationship between the intensity of fluorescence as a function of the intensity of reflected white light per unit area [Adapted from López-Cabrera et al., 2020].
While the exact function of fluorescence in scorpions is yet to be found, various reasons for the presence of this chemical property have been hypothesized. The first hypothesis is that their fluorescence ability does not serve any functional purpose, and thus is only a metabolic byproduct (Gaffin et al., 2011). However, a different hypothesis suggests that this ability can affect the scorpion’s locomotion and ability to find refuge. It has been discovered that scorpions are specifically sensitive to UV and green light, therefore suggesting that the carapace is used to detect the presence of UV light as this is when they emit a photon of visible light. Since they mainly travel at night when shorter wavelengths of light are emitted, this sensitivity can help them find an adequate refuge where they are less exposed to UV light (López-Cabrera et al., 2020). While there are multiple other hypotheses, scorpions’ fluorescence function remains unknown. Further research on this can be done and may result in engineering new materials and technologies inspired by their fluorescence ability or light sensitivity for example.
Conclusion
The carapace is an impressive biological structure with many functions. It may serve as inspiration for a variety of materials. The carapace is found in a wide variety of animals including mammals, arthropods, and reptiles and the carapace itself varies greatly among them. This variety begins when considering the formation of the carapace. In turtles, the carapace originates from an integrated rib cage whereas, in crustaceans, the carapace originates from mineral deposition and calcification. These differences allow the carapace to have many possible functions depending on the organism. The turtle carapace’s keratin coating enhances the toughness and structural integrity of the carapace. The softer layer of a lobster’s carapace is shed and reformed with calcification. The lobster carapace has an additional camouflaging function. Similarly, the scorpion’s carapace has a fluorescent photobiological function. A greater understanding of the composition of the carapace as a material has helped to uncover the mechanisms behind its function. Understanding these mechanisms may help in reverse engineering processes to solve challenges, innovate and improve materials. These biological mechanisms may inspire future materials in the field of biomimetics and materials engineering.
References
Achrai, B., Bar-On, B., & Wagner, H. D. (2015). Biological armors under impact–effect of keratin coating, and synthetic bio-inspired analogues. Bioinspiration & biomimetics, 10(1). https://doi.org/10.1088/1748-3190/10/1/016009.
Achrai, B., & Wagner, H. D. (2017). The turtle carapace as an optimized multi-scale biological composite armor: A review. Journal of the Mechanical Behavior of Biomedical Materials, 73, 50-67. https://doi.org/10.1016/j.jmbbm.2017.02.027.
Bavendam, F. [Sponge crab wearing a hat of yellow sponge for camouflage] Minden Pictures/Nat Geo Image Collection. https://www.mindenpictures.com/stock-photo/sponge-crab-(austrodromidia-octodentata)-wearing-a-hat-of-yellow-sponge-for/search/detail-0_00150704.html
Chen, I. H., Kiang, J. H., Correa, V., Lopez, M. I., Chen, P.-Y., McKittrick, J., & Meyers, M. A. (2011). Armadillo armor: Mechanical testing and micro-structural evaluation. Journal of the Mechanical Behavior of Biomedical Materials, 4(5), 713–722.https://doi.org/10.1016/j.jmbbm.2010.12.013
Gaffin, D. D., Bumm, L. A., Taylor, M. S., Popokina, N. V., & Mann, S. (2011). Scorpion fluorescence and reaction to light. Animal Behaviour, 83(2), 429–436. https://doi.org/10.1016/j.anbehav.2011.11.014
Gilbert, S. F., Cebra-Thomas, J. A., & Burke, A. C. (2008). How The Turtle Gets its Shell. Biology of Turtles, 1–16. https://doi.org/10.1201/9781420004977-5
Gilbert, S., Loredo, G., Brukman, A., & Burke, A. (2001). Morphogenesis of the Turtle Shell: The development of a novel structure in tetrapod evolution. Evolution and Development, 3(2), 47–58. https://doi.org/10.1046/j.1525-142x.2001.003002047.x
Hegdahl, T., Gustavsen, F., & Silness, J. (1978). The Structure and Mineralization of the Carapace of the Crab (Cancer pagurus L.) 3. The epicuticle. Zoologica Scripta, 6(3), 215–220. https://doi.org/10.1111/j.1463-6409.1978.tb00772.x
Horizons, I. L. (2022). Shellfish information-Blue Crab Molting. Retrieved October 30, 2022, from https://barnegatshellfish.org/blue_crab_molting.htm
Jackson, D. C. (2000). How a turtle’s shell helps it survive prolonged anoxic acidosis. Physiology, 15(4), 181–185. https://doi.org/10.1152/physiologyonline.2000.15.4.181
Lawrence, E.M. (2014). Structures and Properties of Keratin-Based and Related Biological Materials. In Handbook of Materials Structures, Properties, Processing and Performance (1-24). Springer, Cham. https://doi.org/10.1007/978-3-319-01905-5_28-1.
Li, S., Kim, C., Zong, S., Liu, Y., Kang, J., Pak, C., Cao, Z., & Wu, Y. (2022). Dynamic synthesis and transport of fluorescent substances from moulting scorpions. Journal of Photochemistry and Photobiology B: Biology, 234, 112511. https://doi.org/10.1016/j.jphotobiol.2022.112511
López-Cabrera, D., Ramos-Ortiz, G., González-Santillán, E., & Espinosa-Luna, R. (2020). Characterization of the fluorescence intensity and color tonality in the exoskeleton of scorpions. Journal of Photochemistry and Photobiology B: Biology, 209, 111945. https://doi.org/10.1016/j.jphotobiol.2020.111945
Mynott, S., Stevens, M., Daniels, C., & Widdicombe, S. (2018). Using camouflage for conservation: colour change in juvenile european lobster. Biorxiv, (2018 10 01). https://doi.org/10.1101/431692
Naylor, P. [Photograph of spiny spider crab covered in algae creating camouflage] https://marinephoto.co.uk/
Passano, L. (1960). Molting And Its Control. Metabolism and Growth, 473–536. https://doi.org/10.1016/b978-0-12-395628-6.50021-x
Rhee, H., Horstemeyer, M., & Ramsay, A. (2011). A study on the structure and mechanical behavior of the Dasypus novemcinctus shell. Materials Science and Engineering: C, 31(2), 363–369. https://doi.org/10.1016/j.msec.2010.10.012
Roer, R. D., & Dillaman, R. M. (2018). The Initiation and Early Stages of Postmolt Mineralization in the Blue Crab, Callinectes sapidus. Frontiers in Marine Science, 5. https://doi.org/10.3389/fmars.2018.0015
Roer, R., & Dillaman, R. (1984). The Structure and Calcification of the Crustacean Cuticle. American Zoologist, 24(4), 893–909. https://doi.org/10.1093/icb/24.4.893
Salares, V. R., Young, N. M., Bernstein, H. J., & Carey, P. R. (1977). Resonance raman spectra of lobster shell carotenoproteins and a model astaxanthin aggregate. a possible photobiological function for the yellow protein. Biochemistry, 16(21), 4751–6. https://doi.org/10.1021/bi00640a034
Schoch, R. R., Klein, N., Scheyer, T. M., & Sues, H.-D. (2019). Microanatomy of the stem-turtle Pappochelys Rosinae indicates a predominantly fossorial mode of life and clarifies early steps in the evolution of the Shell. Scientific Reports, 9(1). https://doi.org/10.1038/s41598-019-46762-z
Tlusty, M., & Hyland, C. (2005). Astaxanthin deposition in the cuticle of juvenile american lobster (homarus americanus): implications for phenotypic and genotypic coloration. Marine Biology : International Journal on Life in Oceans and Coastal Waters, 147(1), 113–119. https://doi.org/10.1007/s00227-005-1558-0
Travis, O. F. (1955). The Molting Cycle of The Spiny Lobster, Panulirus Argus Latreille. Ii. Pre-Ecdysial Histological and Histochemical Changes In The Hepatopancreas And Integumental Tissues. The Biological Bulletin, 108(1), 88–112. https://doi.org/10.2307/1538400
Todd, A. (2017). [Photograph of iridescent white female lobster with notched tail] https://www.willbyers.com/blog/white-lobster-cocaine-leucism
Volschenk, E. S. (2005). A new technique for examining surface morphosculpture of scorpions. Journal of Arachnology, 33(3), 820–825. https://doi.org/10.1636/s03-047.1
Wang, B., Yang, W., McKittrick J., & Meyers., M.A. (2016) Keratin: Structure, mechanical properties, occurrence in biological organisms, and efforts at bioinspiration. Progress in Materials Science, 76, 229-318. https://doi.org/10.1016/j.pmatsci.2015.06.001.
Why Do Apples Turn Brown After You Cut Them? (2019, August 21). Let’s Talk Science. https://letstalkscience.ca/educational-resources/stem-in-context/why-do-apples-turn-brown-after-you-cut-them
Zagalsky, P. F. (1982). A study of the yellow astaxanthin-proteins of lobster carapace. Comparative Biochemistry and Physiology Part B: Comparative Biochemistry, 71(2), 243–247. https://doi.org/10.1016/0305-0491(82)90248-6