An Overview of Transport-Related Interspecies Relationships
Dorothy Ma, Beth Cushnie, Anh Ngo, Tanoulu Li
Abstract
There are many different types of interspecies interactions, and they can benefit or harm the organisms involved. This paper briefs several cases where organisms interact with each other in ways that involve transportation, namely using other species for the purpose of transport. The theme of transport focuses on the physical aspects that propel these biological relationships as opposed to chemical cues. The interspecies relations investigated include barnacles attaching to dolphins to feed more efficiently, the goby fish and pistol shrimp working together for housing and sight respectively, a fungus taking control over the body of its host to facilitate its own growth and fitness, a flatworm modifying the appearance of a snail to resemble a caterpillar, and more. While exploring these relationships, one can see similarities and differences, in both the desired outcomes of the interactions, and the methods employed by the organisms, whether parasitic, commensal, or mutualistic.
Introduction
An essential part of any ecosystem is the interactions between species. These symbiotic interactions can be beneficial or negative for the survival of the organisms involved. Three common types of symbiotic interactions are mutualism, commensalism, and parasitism. A mutualistic relationship benefits both species, while commensal and parasitic relationships both benefit one species while the other species sustains no noticeable effect or is harmed, respectively (Bronstein & Holland, 2008).
These relationships develop by species trying to survive and reproduce: a small fish needing a safe home, or a parasitic flatworm trying to reproduce and grow its population. Given that most organisms need to move to find food, reproduce, grow their habitat, and survive, transportation is often a focus for these relationships. In this paper, different types of symbiotic relationships with aspects specifically related to the transportation of one of the species are examined to observe how organisms use each other to survive.
Commensalism: Barnacle Efficiently Filter Feeds Via Attachment to Cetacean
The goal of barnacles is to attach to somewhere favourable for reproduction and feeding. As free-floating organisms with planktonic larvae at the mercy of ocean currents, different barnacle species attach themselves to various substrates to secure food availability and survival. The Xenobalanus globicipitis stands out amongst barnacles, as they secure themselves not to mossy rocks or stationary coral, but to cetaceans. This section will focus on the commensal relationship between the X. globicipitis and the various dolphin species it chooses to attach to, namely the Tursiops truncatus, or the bottlenose dolphin. The barnacles attach themselves to the dolphin and are transported around the ocean, allowing them to filter feed. This relationship with the bottle-nosed dolphins highlights two points of interest regarding barnacle attachment which are not observed in other relationships: the attachment mechanisms to the surface of an organism that is constantly sloughing their skin, and the hydrodynamic factors that influence attachment position on a dynamic, fast-swimming organism.
Mechanism for Attachment on Substrate
While the substrate of barnacles varies greatly, the mechanism for attachment is unanimously observed across species. X. globicipitis, like all barnacles cyprids, attach themselves to their substrate using a cement protein, which polymerizes with sea water, securing the cyprid to its substrate (Dreyer et al., 2020). This, in combination with the presence of dense villi, helps increase the surface area of chemical secretion and allows for barnacles to attach to a variety of surfaces, including the bottlenose dolphin (Dreyer et al., 2020). This process is observed to be highly effective, as barnacles were able to successfully attach even to blue whales, who have been observed to sustain speeds up to 33 km/hr, suggesting that the speed or movement of the substrate does not necessarily factor into the successful attachment of a barnacle (Kane et al., 2008).
What differs in X. globicipitis from other barnacles is their development on the surface of the cetacean. Bottlenose dolphins have been observed to slough their outermost cell layer 12 times a day on average, which poses a problem for an organism that needs to be attached to its surface (Hicks et al., 1985). To counteract this, X. globocipitis have adapted a specific mechanism that allows it to hold onto the dolphin. The cyprid develops with six footplates at the basal attachment, radiating from the pedicle at the centre (Pugliese et al., 2012). Concentric layers of rings form from the bottom of the plate, with the newest ring deepest in the skin, and the oldest near the surface (Fig. 1) (Pugliese et al., 2012). This allows for the oldest rings to be shed with the skin while keeping the attachment secure.
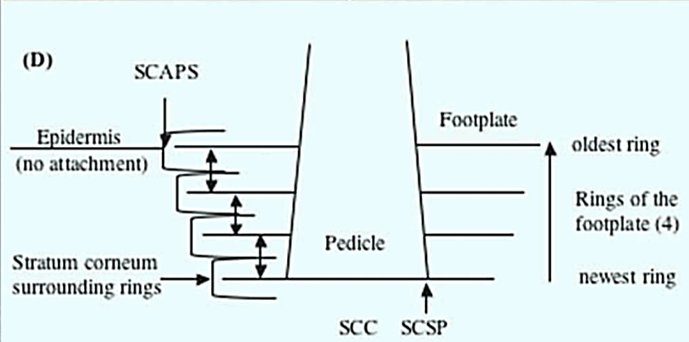
The attachment of the barnacle also inhibits the skin directly underneath from being sloughed, with observations of significantly thicker stratum corneum and the presence of layers of dead skin cells and keratinization directly beneath the pedicle of the barnacle (Pugliese et al., 2012). As new rings grow underneath, the unshed layers of stratum corneum present between the ring layers further anchor the barnacle to the skin of the dolphin.
Favourable Attachment Locations Based on Dolphin Hydrodynamics
As free-floating cyprids, unsettled barnacles have little control over where they will attach on the substrate. As such, a point of interest is how strongly the factor of hydrodynamics of the dolphin influences the location at which the barnacle attaches. The physical build of X. globicipitis is has adapted under hydrodynamic pressures. It has a smooth, linear, flexible, and elongated body in order to successfully filter feed while withstanding strong currents (Moreno-Colom et al., 2020; Pugliese et al., 2012).
X. globicipitis is most observed in the trailing edges of the host's appendages, and most commonly on the tail, or fluke, of the dolphin (Carrillo et al., 2015; Moreno-Colom et al., 2020; Pugliese et al., 2012). This is unsurprising, as regardless of where the barnacle makes contact with the substrate, the currents flowing around the swimming dolphin would lead the barnacle to trail to the edges. However, this would likely only be the case for short distances, as the unidirectional current flow around the body is strong relative to the small cyprid (Carrillo et al., 2015). Attachment success relies on lower water flow, such that the barnacle will have enough time and contact with the substrate to attach itself. Knowing this, the fin and fluke are the few areas of the dolphin that create vortices and eddies (Carrillo et al., 2015; Pugliese et al. 2012). Vortices circulate the water around the fin, allowing the same body of water that may contain the larvae to make more contact with the substrate (Carrillo et al., 2015). This prediction is consistent with the fact that barnacles are most common on the fluke where two vortices are created through the dolphin's lift and thrust movement, as compared to the fins that create just one (Carrillo et al., 2015).
Additional Hydrodynamics Factors of the Fluke
More careful observations found barnacles are most dense near the centre on the upper, or dorsal, side of the fluke (Carrillo et al., 2015; Moreno-Colom et al., 2020). This is because the tail fins are not rigid, therefore, the bending of the edges will curve the fluke; this effect is known as cambering, and is found to be most extreme at the centre (Fish et al., 2006). It is suggested that the water from the camber will preferentially flow through the notch on the tail, providing more contact with the water and possibly larvae near the centre (Carrillo et al., 2015). Additionally, the dorsal side of the fluke is observed to be twelve times more likely to host barnacles compared to the bottom, or ventral, side (Moreno-Colom et al., 2020). This suggests that the bottlenose dolphins exhibit a more forceful down stroke compared to the lift of the fluke (Moreno-Colom et al., 2020). The imbalance in the thrust causes both larger vortices above the fluke, encouraging more barnacle settlement on the dorsal side, while also making the ventral side experience more aggressive shakes and stronger current.
Barnacles are also seen to exhibit aggregate behaviour (Fig. 2), with whales commonly observed to have exclusively no barnacles or three or more (Kane et al., 2008). Further evidence shows that the first nearest neighbour in a group of trailing barnacles is closer together than would be expected from random chance (Moreno-Colom et al., 2020). These observations imply that barnacles recruit other larvae indirectly through physical or chemical means. The attached trailing barnacles will generate drag on the dolphin, producing more eddies (Carrillo et al., 2015). This helps increase the larval contact near the initial barnacle, thereby promoting other barnacles to attach (Carrillo et al., 2015). It should be noted that there is a chemical factor involved, where attached barnacles will produce chemicals to attract newly recruited cyprids to approach their side of the fluke (Moreno-Colom et al., 2020). However, the initial aggregation is largely hydrodynamics based, with chemically encouraged movements once the cyprid has already made contact with the substrate.
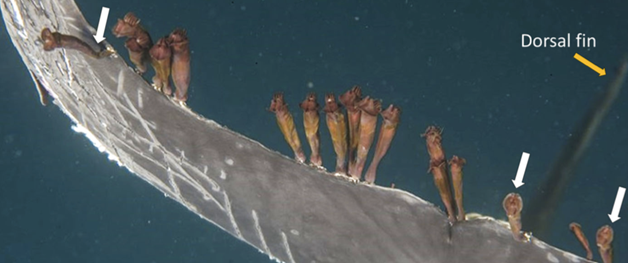
The commensal relationship between X. globicipitis and its cetacean hosts highlights the physical factors that affect the barnacle's attachment to its host, both those within its control such as the mechanisms and adaptations to attach to a sloughing, dynamic host, as well as those without, such as the hydrodynamics of the host.
Mutualism: Goby Fish and Pistol Shrimp: Perfect Partners
In the oceans, goby fish, the Gobiidae, and pistol shrimps, the Alpheidae, form a famous symbiotic relationship. The pistol shrimps and goby fish usually begin to live together when they are young and stay in pairs when becoming adults (Karplus, 1992). They forage together and live in a burrow (Fig. 3). Pistol shrimps only leave their burrow with the gobies, and neither shrimp nor goby fish stays alone in a burrow (Karplus, 1992). In the symbiotic pair they form, their relationship is beneficial to both.
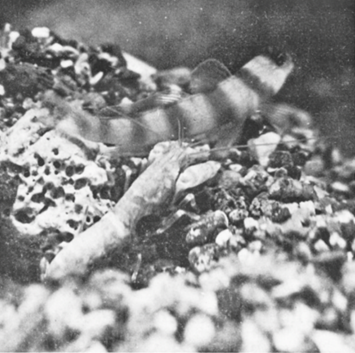
As its name indicates, one of the claws of the pistol shrimp is strong, which is used to build burrows as habitats. The shrimps constantly maintain the burrows and hide the burrows at night (Karplus, 1992; Karplus & Thompson, 2011). In contrast, the goby fish cannot build a good burrow. Therefore, living in the burrow with the shrimps provides the goby fish a safe place to sleep. Additionally, gobies will have a safe place for mating, which takes a long time (Karplus & Thompson, 2011).
Though pistol shrimps are good at building burrows, they are almost entirely blind, and thus the environment outside their burrows is hazardous for them (Zeng & Jaafar, 2012). When they leave their burrows for foraging, it is difficult for them to spot the predators. However, the goby fish can help the shrimps when they are together as the goby has good sight (Karplus, 1992; Zeng & Jaafar, 2012). The gobies always keep looking out for any potential dangers at the burrow entrances and outside. They will alert the shrimps in the presence of danger (Karplus, 1992).
The Communication System Between Pistol and Shrimps
Due to the terrible eyesight of the pistol shrimps, tactile communication is the primary way for them to communicate with each other. When they leave their burrows, the shrimps are constantly touching the goby fish with their antennae to keep contact with the gobies (Fig. 4) (Preston, 1978). When gobies spot dangers, they will flick their tail to alert the shrimps of the danger, and the shrimps will go back to the burrows with the gobies immediately (Karplus, 1992; Preston, 1978).
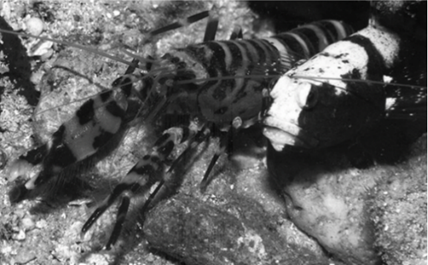
Mutualism: Hawaiian Bobtail Squid and Their Lightbulb
The Euprymna scolopes, commonly known as the Hawaiian bobtail squid, is a small creature that lives in shallow waters of the Pacific Ocean (McFall-Ngai, 2008). During the day they hide from predators, and they hunt for food, namely shrimp, at night (McFall-Ngai, 2008). They are therefore classified as nocturnal animals (McFall-Ngai, 2008).
By going out for food at night, the squids put themselves in danger, exposed to predators because of the shadow cast by the moon. However, they have a light organ that generates a bioluminescent glow, imitating natural light like that from the stars or moon, and this helps the squid hide from predators and prey alike. Because their visibility will be different depending on the position of the predators, the squids, therefore, need to adjust their bioluminescent glow. To make predators underneath mistake them as the moonlight, the squids have a tissue composed of reflectins – protein-based reflectors – which direct the light downwards (Crookes et al., 2004). For predators above, this creature has an ink sac situated above its light organ, which helps absorb all the light generated upward, thus making the animal appearing dark and blending in the dark ocean floors (Ruby, 1996). This strategy is called counter illumination, a method widely used by marine animals for active camouflage; they blend in with their backgrounds, both in terms of brightness and wavelength (Fig. 5).
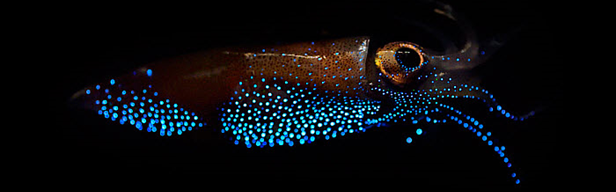
The Truth About This ‘Lightbulb'
Surprisingly, the luminescence emitted from the squid's light organ is not produced by the squid itself, but by handy microscopic organisms, Vibrio fischeri. These creatures create this light through the course of bioluminescence (Jones & Nishiguchi, 2004). A newly hatched Hawaiian bobtail squid is completely free of bacteria, but within a few hours, this Vibrio fischeri colonizes its light organs (Fig. 6) and ensures its correct development (Jones & Nishiguchi, 2004). In return for their bioluminescence, the squid supplies supplements, like sugars and amino acids, and welcomes the microorganisms like guests in their home (Jones & Nishiguchi, 2004).
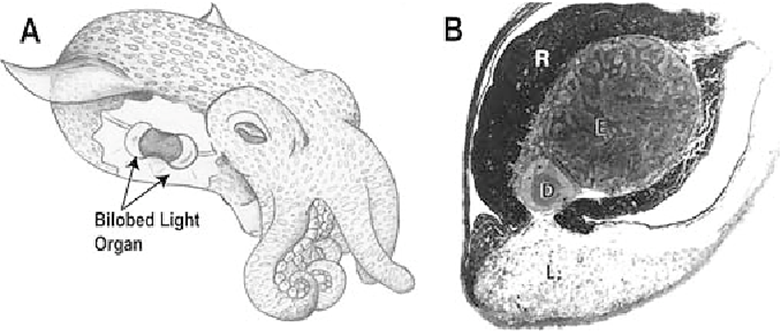
As the moon does not shine continuously throughout the night, the squid must stay alert and modify the bacteria's bioluminescence. To reduce the light, the squid has three behavioral responses (Jones & Nishiguchi, 2004). Firstly, it can utilize its ink sac like an iris to adjust the light intensity (Jones & Nishiguchi, 2004). Secondly, yellow filters covering the squid's ventral surface can modify the wavelength of the light (Jones & Nishiguchi, 2004). Lastly, the squid can reduce the oxygen concentration in its gas bladder, leading to a slow fall off in light production, though scientists are still unable to explain why this phenomenon happens (Stabb, 2005). Beyond that, the bacteria themselves release molecules, called N-acyl homoserine lactone (HSL) Autoinducers (Kolibachuck & Greenberg, 1993). These interact with a regulator, LuxR, to control the expression of the genes involved in the production of blue-green light (Kolibachuck & Greenberg, 1993).
The number of bacteria also varies depending on the time of day (Lee & Rugby, 1994). At dawn, by thinning the tissue in its light organ, around 95% of the bacteria in the light organ are ejected, because having a lot of bacteria in the light organ is very costly in terms of metabolism. Each light-emitting organ contains 10⁷ to 10⁹ bacteria (Lee & Rugby, 1994). During daytime hours, the quantities of microorganisms gradually increase from the leftover 5%, and, by nightfall, when bioluminescence is required for hunting, the light organ is almost full of bacteria (Lee & Rugby, 1994).
Parasitism: Fungus Invades Ant Muscle Functionality
Of species that use another species for transport, few do so as forcibly and directly as the parasitic fungus of the genus Ophiocordyceps with the ant. This section will focus specifically on Ophiocordyceps unilateralis and its parasitic relationship with the Camponotus carpenter ant. Both species are commonly observed in tropical forest ecosystems, while preferring relatively opposite environments. Carpenter ants live in the hot, dry canopies, occasionally venturing into the humid understory, which is the optimal growing environment for the fungus (Hughes et al., 2011).
Although O. unilateralis targets carpenter ants specifically, the ant colony does not present a sustainable environment for its growth and reproduction. The fungus uses the ant to transport and secure itself to an ideal location for its growth and spore dispersal: a few centimetres off the ground, on a stem under a leaf, where there it can flourish under constant low temperatures and high humidity (Andersen et al., 2009). The fungus does so by invading the ants' muscle fibres, thereby controlling its movements, then binding itself permanently to the stem of choice using the ants' mandibles and killing the host for its nutrients in development.
Invasion of Ant Muscle Cells from Fungal Hyphal Bodies
Looking inside the ant's body showed that the fungal cells were numerous in the muscle fibers, and particularly in the head, although never entering the brain nor the nervous system (Fredericksen et al., 2017; Mangold et al., 2019). This shows that the fungus can control the ant's movement, down to the functionality of its jaws through the manipulation of muscles. Serial block-face scanning-electron microscopy (SEM) revealed that the spaces between muscle fibres were larger because fungal cells surrounded them (Fig. 7), displacing the muscle cells (Fredericksen et al., 2017).
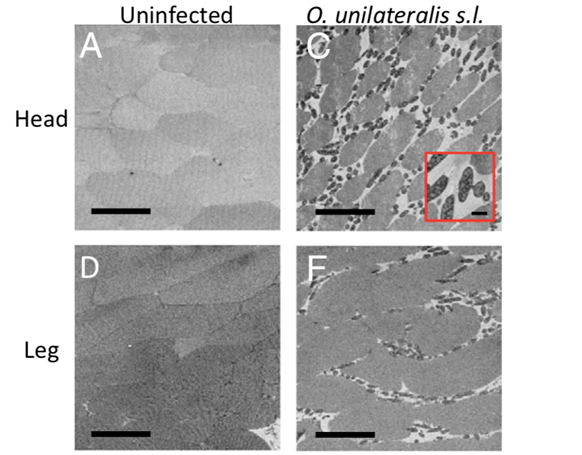
Furthermore, 77% of all hyphal bodies (fungal cells that populate the cell by budding) observed were in direct contact with the muscle fibres, with a few even penetrating the muscle cell (Fig. 8) (Fredricksen et al., 2017). 59% of hyphal bodies were interconnected to an extensive network that could share cell content and information between neighbouring cells, forming a mycelium, with 75% of the hyphal bodies not in direct contact with the muscle cells to have indirect contact through the network (Fredericksen et al., 2017; Mangold et al., 2019).
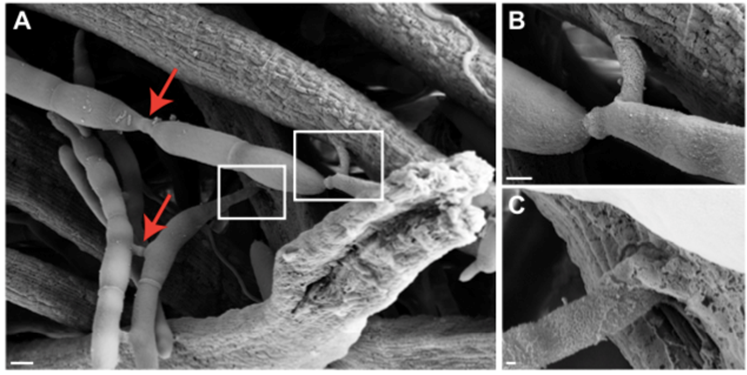
Behavioral Changes Induced by Fungal Invasion
The effects of the physical invasion of fungal cells on the ant's muscles is observed externally through unusual behaviour exhibited by the ant. Carpenter ants occasionally venture from their canopy to the understory but will stay on a designated trail and return up the trunk shortly after; when ants from the study were purposefully placed off trail, they quickly and immediately returned to their usual track (Hughes et al., 2011). However, infected ants were observed to walk alone, wandering to the extent that they would traverse twice as many leaves as uninfected ones (Hughes et al., 2011). Such irregular behaviour shows that the infected ant is not in control of its movement. This is most evidently exhibited when the ants experience full body convulsions and repeatedly fall into the understory as they were on track to return to the canopy (Hughes et al., 2011). This is likely a way to keep the ant in the understory until the fungus is ready to continue to its dispersal stage and kill the host.
Precise Mandible Muscle Control
Once the fungus is ready to start its external growth and spore dispersal, it guides the ant to a favourable location on the stem of a plant in the understory and fixes itself with the fittingly named “death grip” using the ant's mandibles. This requires the fungus to inhibit the ant's mandibles from reopening and releasing. There are two theories proposed as to how the fungus does this: atrophy of the mandible muscles after biting, or the hypercontraction of the mandible muscles.
Both derive from similar observations in the muscle fibres of infected ants. As mentioned earlier, the presence of the fungal cells creates larger spaces between muscle fibres, both displacing the cells, but also reducing the available space for mitochondria and sarcoplasmic reticulum, organelles that provide ATP and Ca++ ions respectively (Hughes et al., 2011). Additionally, the fungal network in contact with the cell is also likely consuming the nutrients from the mitochondria and sarcoplasmic reticulum in those cells, significantly reducing their number and functionality. The control and production of ATP and Ca++ are essential for muscle contraction and relaxation. The z-lines (“anchor points for actin-myosin cross linking” in muscles) were also found to be irregular, swollen, or disconnected (Hughes et al., 2011; Mangold et al., 2019). Many of the mandible muscle fibres, which are normally attached to the head, were disconnected or stretched, suggesting atrophy of the mandibles (Hughes et al., 2011). Meanwhile, other observations of muscle cells suggest the mandible dysfunction is a result of hypercontraction. Namely, the swelling of z-lines, the shortening of sarcolemma, and the formation of myelin-like structures in the infected muscles are observed as responses to hypercontraction in humans and rats respectively (Mangold et al., 2019). In both cases, the physical accumulation of the hyphae body in the ant results in a disconnected muscle unit from the rest of the organism, which is how the ant remains fixed even after death.
The parasitic relationship between Ophiocordyceps unilateralis and the carpenter ant presents a case where control of the host does not involve taking over the brain of the species, but the muscles through physical invasion and manipulation.
Parasitism: Behaviour Changes to the Intermediate Host of Leucochloridium
Trematoda is a class within the phylum Platyhelminthes that consists entirely of parasitic flatworms. Some of the species are of the genus Leucochloridium, a pulsating broodsac that infects snails and birds.
Snails of the family Succineidae, known as amber snails, ingest the eggs of the Leucochloridium, and become intermediate hosts for the parasite. The Leucochloridium eggs reproduce asexually inside the snail and grow into branching sporocysts, and then into colourful, pulsating, mature broodsacs. The broodsacs will grow into one or more of the snail's eyestalks, causing it to look like a caterpillar, which increases the probability of the snail being eaten by a bird (Fig. 9). When the bird eats the snail, it ingests the broodsacs. Leucochloridium are hermaphroditic, so they produce eggs in the bird, which are then released in the bird's feces. The new eggs are ingested by a new snail, and the process repeats itself (Mehlhorn, 2016; Nakao et al., 2019; Wesołowska & Wesołowski, 2014).
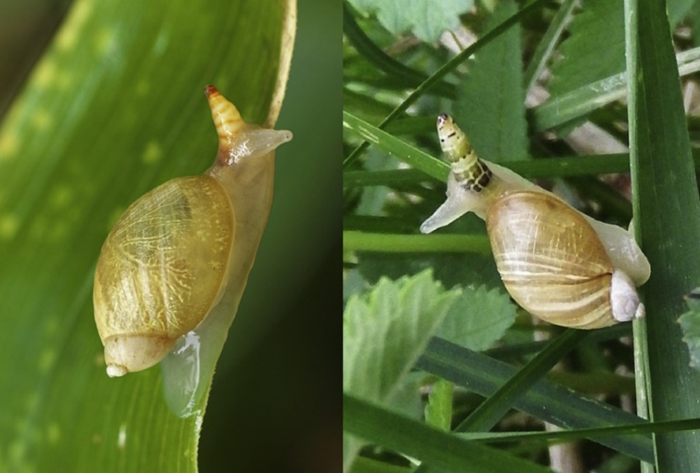
This process is highly detrimental to the snails. First, broodsacs can occupy a significant part of the body cavity of the snail, which severely suppresses its sexual maturation, and they steal energy from the snail (Moore, 2002; Nakao et al., 2019). The main problem for the snail, however, is that for the Leucochloridium life cycle to continue, the snail must be eaten. So, like most intermediate host parasites, the trematodes change the behaviour of their hosts to increase their probability of being eaten (Moore, 2019; Wesołowska & Wesołowski, 2014).
As mentioned previously, the broodsac's colour causes the snails to look more similar to caterpillars. However, infected snails were also found to stay in more open, well-lit spaces, were found higher above the ground, and moved around more (Fig. 10). This behaviour makes them more visible to birds, and therefore increases their chances of being eaten, and allows the flatworm to lay its eggs and continue its life cycle (Wesołowska & Wesołowski, 2014). Unfortunately, it is not clear how exactly these behavioural changes are produced (Moore, 2019). Despite all this, the final hosts, the birds, are relatively unaffected (Mehlhorn, 2016).
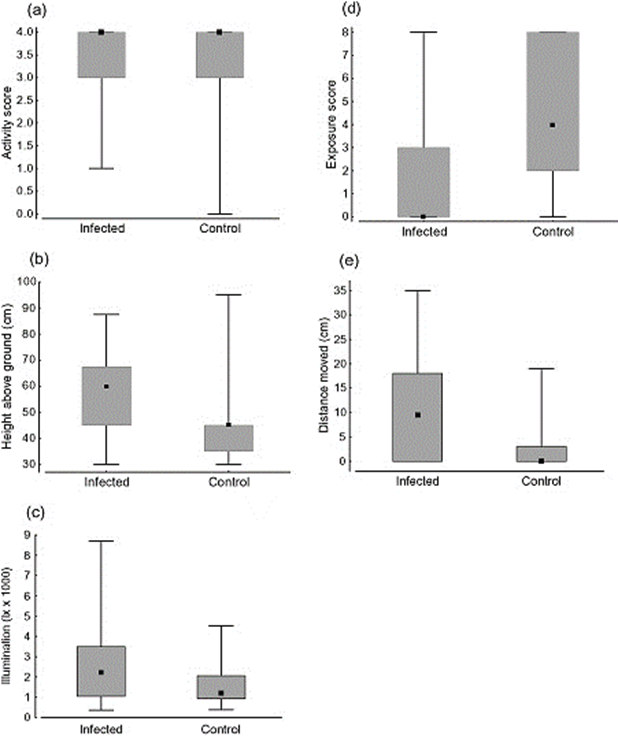
Range of Leucochloridium
There are many different species of Leucochloridium, and they have been found all over the world, including Asia, Europe, and North America, Brazil, and Australia. The life cycle and general morphology of different species is the same, although color of the broodsac can differ, as well as size. Species are not confined to one location; L. paradoxum and L. perturbatum were found in both Europe and Japan, despite the distance between them (Nakao et al., 2019).
The habitat of the Leucochloridium is linked to the habitat of its host, and amber snails are found all over the world. Thus, it makes sense that Leucochloridium are also widespread. Additionally, their final hosts, birds, can travel large distances, and are usually migratory birds (Nakao et al., 2019). If a bird is infected with a broodsac, then travels to a new location with amber snails, the cycle will start again in this new location, increasing the range of the Leucochloridium. The prevalence of a specific species of Leucochloridium can be hypothesized to be determined based off the preferences of local birds, as they may prefer different colors or sizes (Nakao et al., 2019). The bird's favourite caterpillar look-alike will be eaten more, and their eggs will spread more.
Parasitism: Aphids and Plants
Aphids, the Aphididae, are a large family of insects. They are all tiny and soft-bodied and form parasitic relationships with many different species of plants (Kennedy & Stroyan, 1959). One such example is the black bean aphid, (Aphis fabae Scopoli) and its host plant, the snowball (Viburnum opulus L.) (Döring, 2014). Nowadays, aphids are common and severe agricultural pests due to their high reproductive capacity and the serious harm they cause to their host plants (Kennedy & Stroyan, 1959).
In the parasitic relationship, the whole life cycle of aphids is closely related to their host plants (Moran, 1992). The sap of the plants is only the food resource for aphids, particularly for their larvae (Goggin, 2007). Furthermore, the aphid larvae, including those born by both parthenogenetic and sexual generations, are usually left on the plants to grow after their mothers' reproduction (Goggin, 2007; Moran, 1992). In short, without their host plants, aphids cannot live.
However, as these are parasitic relationships, aphids only bring negative impacts to plants. Although the aphid infestation may not lead to serious symptoms to their host plants, in the cases where many aphids stay on the plants after reproduction, significant harm is caused to the plants (Goggin, 2007). The common symptoms caused by aphid feeding include chlorosis, wilting, stunted shoots, and poor plant growth (Fig. 11) (Goggin, 2007). Also, aphis may spread the plant viruses to their host plants. These viruses may lead to serious plant diseases that may significantly affect the growth of the plants negatively. (Döring, 2014; Goggin, 2007).
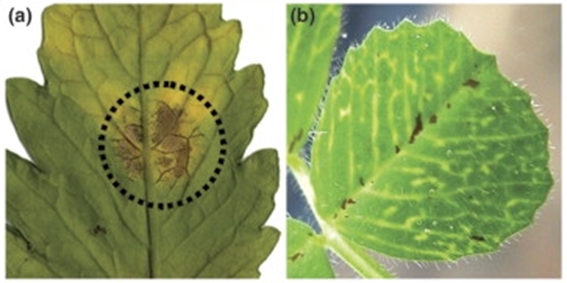
How do Aphids Damage the Host Plants?
Aphids have well-adapted piercing-sucking mouthparts (Fig. 12), which are used to extract sap after penetrating the sieve tubes or other parts of tissues in the host plants (Forbes, 1977). When they are piercing into the plant tissues, their saliva, consisting of numerous enzymes that can damage the host plants, is injected (Goggin, 2007). Therefore, both the saliva and the mechanical damages made by mouthparts will harm the plants. (Forbes, 1977; Goggin, 2007) Additionally, the transmission of plant viruses happens when the plant is pierced. After piercing a plant that has plant viruses, the viruses will be carried by their mouthparts; when they have a new plant host and pierce it, they spread the plant viruses to the new host (Döring, 2014; Goggin, 2007).
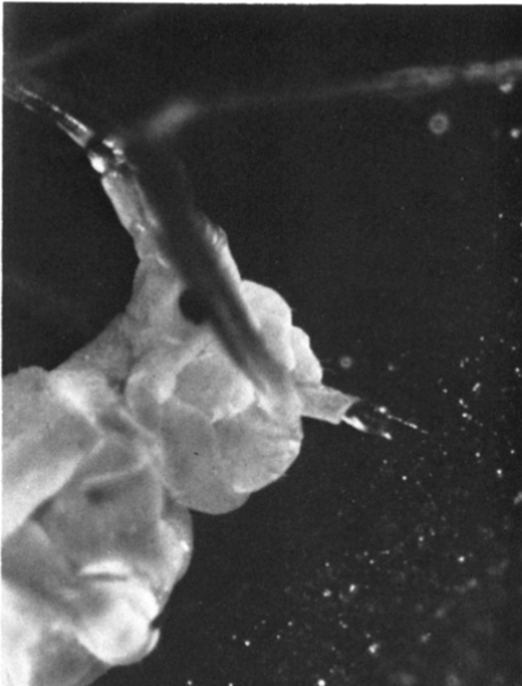
Sexual Parasitism: The Deep-Sea Angler Fish
The deep-sea anglerfish, the Ceratioidei, are marine creatures that live up to 5,000 meters below the surface. There are distinct differences between the males and females of this type of fish, and the latter are certainly the more formidable. The females have a huge head, a jaw sporting dagger-like teeth, and a very long chin (Hartel, 2010). Living in an environment where light does not penetrate and food is scarce, female anglers have a pole with a lamp, located at the end of their mouth (Fig. 13) (Hartel, 2010). Like a neon, it emits bioluminescent radiation, allowing them to attract food and the males (Dickson & Pierce, 2018).
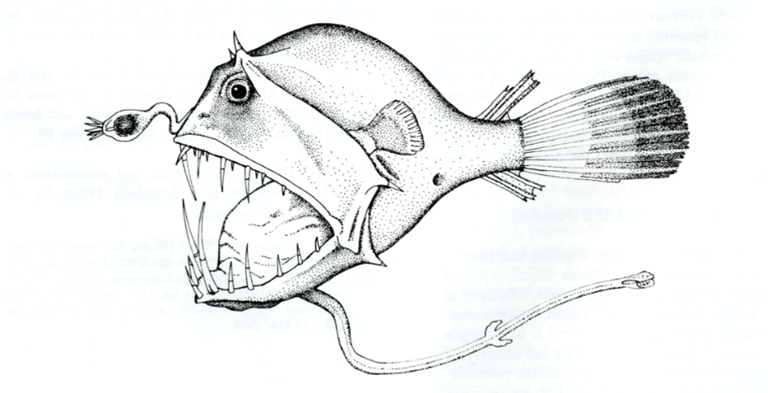
The male anglerfishes, on the other hand, are minuscule and utilize their acute sense of smell and their highly developed eyes to find a mate. Apart from that, they have no teeth, though they can hang onto the female by using special hooks located on their snouts (Hartel, 2010). Pietsch (1975) explains that after finding a mate, whom may be up to several times his size, the male will nip at her stomach. At this point, his mouth ejects a sort of chemical substance to glue him to his bioluminescent partner (Pietsch, 1975). Slowly, his blood and tissues will mix with hers, and the male essentially transforms into a sperm-creating parasite (Pietsch, 1975). Sometimes, the contact is brief — the young males bounce on and off quickly – while with others they become long-lasting appendages on the females. This occurrence is quite rare and is known as sexual parasitism.
How do Angler Fish Mate?
To scientists, sexual parasitism is a bizarre phenomenon, as it should not be possible for two hereditarily different anglerfishes, regardless of how much they resemble each other physically, to blend their tissues without complications (Winter, 2020). For organ transplants in other animals, the transplanted organs are usually rejected by the recipient, and a lot of work is needed for the foreign tissue to be accepted (Winter, 2020). Furthermore, some male anglerfishes can blend their tissue with the female's immediately and become ‘life joiners'. The question of how they manage to do this regardless of their genes, then, has been the subject of much debate.
In their quest to find answers, Bordon (2020) has sequenced anglerfishes' genes and analyzed them. He found that some females or males (either gender of the couple) lose some important genes involved in encoding the production of antibodies and T cells. These are the principal parts of the immune system which help the organism to identify its body cells and eliminate any pathogens or foreign cells. By dropping its immune system, then, the anglerfishes are no longer able to recognize unfamiliar tissue, and thus they can fuse their own tissue with that of their mate. At the same time, however, they are left defenseless against pathogens as they are no longer able to fight them. This is a huge sacrifice for any creature to make for reproduction.
Conclusion
In these examples, we can see similarities between relationships, while also admiring their uniqueness. All of the parasitic relationships revolved around reproduction, but different aspects of it, be it an energy source for their young, or a way to effectively grow and disperse its eggs. The mutualistic/commensal relationships had a wider range and included relationships like the goby fish and pistol shrimp, where reproduction is not even mentioned and focused on overall survival. Different species had different strategies to fulfill their side of the interaction, but some employ the same strategy, like a parasite changing the behavior of its intermediate host to increase its chances of being eaten. Despite the focus on transportation, it is evident that interspecies relationships are incredibly diverse, but all include the same desire to survive.
Many of these unlikely relationships also provide insight into useful mechanisms that may be adapted and further researched. Technology developed based off the cloaking from the Hawaiian bobtail squid can be used for airplane camouflage or radar. The ability of anglerfish to blend their tissue with their mate can be used in tissue engineering, to help prevent graft rejection responses.
References
Andersen, S. B., Gerritsma, S., Yusah, K. M., Mayntz, D., Hywel-Jones, N. L., Billen, J., Boomsma, J. J., & Hughes, D. P. (2009). The Life of a Dead Ant: The Expression of an Adaptive Extended Phenotype. American Naturalist, 174(3), 424-433. https://doi.org/10.1086/603640
Anderson, M. E., & Leslie, R. W. (2001). Review of the deep-sea anglerfishes (Lophiiformes: Ceratioidei) of southern Africa. JLB Smith Institute of Ichthyology.
Bordon, Y. (2020). Loss of immunity lets a sexual parasite hold on tight. Nature Reviews Immunology, 20(10), 590-591.
Bronstein, J. L., & Holland, J. N. (2008). Mutualism. In S. E. Jørgensen & B. D. Fath (Eds.), Encyclopedia of Ecology (pp. 2485-2491): Academic Press.
Carrillo, J. M., Overstreet, R. M., Raga, J. A., & Aznar, F. J. (2015, Jun). Living on the Edge: Settlement Patterns by the Symbiotic Barnacle Xenobalanus globicipitis on Small Cetaceans. Plos One, 10(6), Article e0127367. https://doi.org/10.1371/journal.pone.0127367
Crookes, W. J., Ding, L. L., Huang, Q. L., Kimbell, J. R., Horwitz, J., & McFall-Ngai, M. J. (2004). Reflectins: the unusual proteins of squid reflective tissues. Science, 303(5655), 235-238.
Dickson, B. V., & Pierce, S. E. (2018). How (and why) fins turn into limbs: insights from anglerfish. Earth and Environmental Science Transactions of the Royal Society of Edinburgh, 109(1-2), 87-103.
Döring, T. F. (2014). How aphids find their host plants, and how they don't. Annals of Applied Biology, 165(1), 3–26. https://doi.org/10.1111/aab.12142
Dreyer, N., Zardus, J. D., Hoeg, J. T., Olesen, J., Yu, M. C., & Chan, B. K. K. (2020, Jun). How whale and dolphin barnacles attach to their hosts and the paradox of remarkably versatile attachment structures in cypris larvae (vol 23, pg 861, 2020). Organisms Diversity & Evolution, 20(2), 251-251. https://doi.org/10.1007/s13127-020-00450-3
Fish, F. E., Nusbaum, M. K., Beneski, J. T., & Ketten, D. R. (2006, Dec). Passive cambering and flexible propulsors: cetacean flukes. Bioinspiration & Biomimetics, 1(4), S42-S48. https://doi.org/10.1088/1748-3182/1/4/s06
Forbes, A. R. (1977). The mouthparts and feeding mechanism of aphids. Aphids As Virus Vectors, 83–103. https://doi.org/10.1016/b978-0-12-327550-9.50008-2
Fredericksen, M. A., Zhang, Y. Z., Hazen, M. L., Loreto, R. G., Mangold, C. A., Chen, D. Z., & Hughes, D. P. (2017, Nov). Three-dimensional visualization and a deep-learning model reveal complex fungal parasite networks in behaviorally manipulated ants. Proceedings of the National Academy of Sciences of the United States of America, 114(47), 12590-12595. https://doi.org/10.1073/pnas.1711673114
Goggin, F. L. (2007). Plant–aphid interactions: Molecular and ecological perspectives. Current Opinion in Plant Biology, 10(4), 399–408. https://doi.org/10.1016/j.pbi.2007.06.004
Hartel, K. E. (2010). Oceanic Anglerfishes: Extraordinary Diversity in the Deep Sea. Copeia, 2010(1), 181-182.
Hughes, D. P., Andersen, S. B., Hywel-Jones, N. L., Himaman, W., Billen, J., & Boomsma, J. J. (2011, May 9). Behavioral mechanisms and morphological symptoms of zombie ants dying from fungal infection. BMC Ecol, 11, 13. https://doi.org/10.1186/1472-6785-11-13
Jones, B. W., & Nishiguchi, M. K. (2004). Counterillumination in the hawaiian bobtail squid, Euprymna scolopes Berry (Mollusca: Cephalopoda). Marine Biology, 144(6), 1151-1155.
Kane, E. A., Olson, P. A., Gerrodette, T., & Fiedler, P. C. (2008, Oct). Prevalence of the commensal barnacle Xenobalanus globicipitis on cetacean species in the eastern tropical Pacific Ocean, and a review of global occurrence. Fishery Bulletin, 106(4), 395-404.
Karplus, I. 1987. The association between gobiid fishes and burrowing. alpheid shrimps. Oceanography and Marine Biology Annual Review 25: 507-562.
Karplus, I., & Thompson, A. R. (2011). The partnership between gobiid fishes and burrowing alpheid shrimps. The Biology of Gobies, 559–607. https://doi.org/10.1201/b11397-100
Kennedy, J. S., & Stroyan, H. L. (1959). Biology of aphids. Annual Review of Entomology, 4(1), 139–160. https://doi.org/10.1146/annurev.en.04.010159.001035
Kolibachuk, D., & Greenberg, E. P. (1993). The Vibrio fischeri luminescence gene activator LuxR is a membrane-associated protein. Journal of bacteriology, 175(22), 7307-7312.
Lee, K. H., & Ruby, E. G. (1994). Effect of the squid host on the abundance and distribution of symbiotic Vibrio fischeri in nature. Applied and Environmental Microbiology, 60(5), 1565-1571.
Mangold, C. A., Ishler, M. J., Loreto, R. G., Hazen, M. L., & Hughes, D. P. (2019, Jul). Zombie ant death grip due to hypercontracted mandibular muscles. Journal of Experimental Biology, 222(14), Article jeb200683. https://doi.org/10.1242/jeb.200683
McFall-Ngai M. (2008). Hawaiian bobtail squid. Current biology: CB, 18(22), R1043–R1044. https://doi.org/10.1016/j.cub.2008.08.059
Mehlhorn, H. (2016). Leucochloridium Species. In H. Mehlhorn (Ed.), Encyclopedia of Parasitology (pp. 1468-1469). Springer Berlin Heidelberg. https://doi.org/10.1007/978-3-662-43978-4_4006
Moore, J. (2002). Parasites and the Behavior of Animals. Oxford University Press on Demand.
Moore, J. (2019). Intermediate Host Behavior. In J. C. Choe (Ed.), Encyclopedia of Animal Behavior (Second Edition) (pp. 658-662). Academic Press. https://doi.org/https://doi.org/10.1016/B978-0-12-809633-8.20827-3
Moran, N. A. (1992). The evolution of aphid life cycles. Annual Review of Entomology, 37(1), 321–348. https://doi.org/10.1146/annurev.en.37.010192.001541
Moreno-Colom, P., Ten, S., Raga, J. A., & Aznar, F. J. (2020, Jul). Spatial distribution and aggregation ofXenobalanus globicipitison the flukes of striped dolphins,Stenella coeruleoalba: An indicator of host hydrodynamics? Marine Mammal Science, 36(3), 897-914. https://doi.org/10.1111/mms.12691
Nakao, M., Sasaki, M., Waki, T., Iwaki, T., Morii, Y., Yanagida, K., Watanabe, M., Tsuchitani, Y., Saito, T., & Asakawa, M. (2019). Distribution records of three species of Leucochloridium (Trematoda: Leucochloridiidae) in Japan, with comments on their microtaxonomy and ecology. Parasitology International, 72, 101936. https://doi.org/10.1016/j.parint.2019.101936
Pietsch, T. W. (1975). Precocious sexual parasitism in the deep sea ceratioid anglerfish, Cryptopsaras couesi Gill. Nature, 256(5512), 38-40.
Preston, J. L. (1978). Communication Systems and social interactions in a goby-shrimp symbiosis. Animal Behaviour, 26, 791–802. https://doi.org/10.1016/0003-3472(78)90144-6
Pugliese, M. C., Bottger, S. A., & Fish, F. E. (2012, Apr). Barnacle bonding: Morphology of attachment of Xenobalanus globicipitis to its host Tursiops truncatus. Journal of Morphology, 273(4), 453-459. https://doi.org/10.1002/jmor.20006
Ruby, E. G. (1996). Lessons from a cooperative, bacterial-animal association: the Vibrio fischeri–Euprymna scolopes light organ symbiosis. Annual review of microbiology, 50(1), 591-624.
Stabb, E.-V. (2005). Shedding Light on the Bioluminescence “Paradox”. ASM News, 71(5), 223-229
Wesołowska, W., & Wesołowski, T. (2014). Do Leucochloridium sporocysts manipulate the behaviour of their snail hosts? Journal of Zoology, 292(3), 151-155. https://doi.org/10.1111/jzo.12094
Winter, C. (2020, September 11). Angler Fish Reproduction: When Two Become One. Imperial Bioscience Review. https://imperialbiosciencereview.com/2020/09/11/angler-fish-reproduction-when-two-become-one/
Zankl, S. (2018, August 31) Firefly squid (Watasenia scintillans) emitting light from photophores, Toyama Bay, Japan. This form of bioluminescence is used as counter illumination camouflage. nature picture library. https://www.naturepl.com/stock-photo-nature-image01601191.html
Zeng, Y., & Jaafar, Z. (2012). Visual acuity of the goby-associated shrimp, Alpheus Rapax Fabricius, 1798 (Decapoda, Alpheidae). Crustaceana, 85(12-13), 1487–1497. https://doi.org/10.1163/15685403-00003128