A Portfolio of The Physical Peculiarities of Animal Sleep Behaviour
Kelliane Beland, Taisei Fu, Andres Gonzalez, Grace Shi
Abstract
Sleep is a phenomenon that has been observed to naturally recur in all animals, and which is crucial to their functioning, as it has been posited that it serves to help the animal recover, recuperate and develop. To accommodate their sleep requirements and environmental conditions, certain animals have developed remarkable evolutionary adaptations which confer advantages to the species in question. These perks can advantage the animal thermodynamically, as is the case with chimpanzees and cetaceans. Additionally, animals such as horses, flamingos, and ruminants have evolved their sleep habits to optimize their relationship with the gravity force, whether to mitigate its effects or to maximize its beneficial impacts. Thus, these examples of evolutionary design can serve as the model for other engineering and design initiatives, particularly in cases of static equilibrium.
Introduction
Due to the utmost importance and necessity of sleep for animals, it inevitably leads to the exploration of how different animals sleep and the physics responsible for their sleeping behaviours. Sleep can be loosely generalized and defined as a state of unconsciousness with minimal movement and reaction to external stimuli (Nicolau et al., 2000). Its functions for organisms include energy conservation, recuperation, memory consolidation, and development (Siegel, 2005). Thus, it is valuable to explore these various mechanisms, habits and physiologies evolved to facilitate sleeping, such as how animals use or compensate for gravity while they sleep. As with all systems found in nature, these systems are incredibly energy efficient, and inspiration can be taken from these systems in engineering designs.
Flamingo Sleep Behavior
It is hypothesized that flamingo's incredible ability to stand on one leg is meant to decrease muscular energy expenditure (Chang & Ting, 2017). Since small muscular effort is required to perform the stance, flamingos can easily sleep maintaining this posture. As most birds, flamingos engage in unihemispheric sleep (Anderson et al., 2018). While on one leg and sleeping, flamingos balance their center of pressure strategically to stand in equilibrium (Chang & Ting, 2017). With the right configuration, as illustrated in Fig. 1, they can limit the effects of gravity and stay with minimal effort in a one-legged stance.
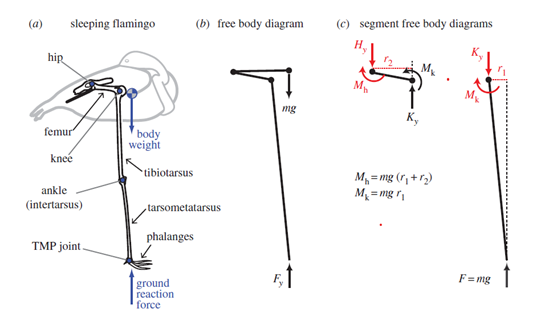
Surprisingly, flamingos control better their center of pressure while resting than when awake. In fact, their center of pressure changes positions greatly when awake than when sleeping in the same position (Chang & Ting, 2017). Their center of pressure stays mostly beneath the TMP joint, which makes sense, since this allows for a gravitational force that has minimal x components.
There are three conditions that need to be met in order to achieve a unidirectional, passively engaged gravitational stay (Chang & Ting, 2017). First, the center of mass must be located anterior to the knee joint which creates passive gravitational knee extensor and hip flexor moments. Second, the anatomical configuration of knees and hip limit movement, which opposes these moments. Finally, the foot must be placed medially to the hip joint in the frontal plane to maintain this joint configuration. Failure to meet these conditions allows for free joint movement, which would require energy to maintain in equilibrium.
The balance behavior of flamingos on one leg resembles the behavior of an inverted pendulum (Chang & Ting, 2017), which is achievable with little energy. It is suggested that adopting a one-leg stance is even more energetically efficient than standing on two legs.
Cetacean Sleep Behavior
Cetaceans, unlike terrestrial mammals, present an unusual form of sleep behavior. All cetaceans studied to date engage in USWS (unihemispheric slow wave sleep), which is characterized by one hemisphere undergoing the usual SWS, while the other hemisphere is relatively active (Mukhametov, 1984). This form of sleep represents more than 70% of the sleep amount time, while the remaining 30% is characterized by bilateral SWS, but highly different from any other mammal species (Lyamin et al., 2008). There is no evidence that cetaceans experience REM (rapid eye movement) sleep (Mukhametov, 1995), but one cannot fully reject this possibility, as it might exist in a completely different form.
The practice of USWS leads to the more unusual behaviors in cetaceans. In fact, USWS allows them to swim while sleeping, or slow swimming and sleep on the surface of water. Slow swimming has been observed along all categories of cetaceans, especially in rather small cetaceans such as river dolphins. Slow swimming might be analogous to bird's resting behavior, since cetaceans such as killer whales have been observed slow swimming in tight formation, synchronizing their breaths and dives (Ford, 2009). This form of sleep allows small mammals to fight the current, as their weight alone can't oppose water's strength. Moreover, slow swimming helps heat production in small cetaceans as muscle activity is greater compared to motionless resting (Lyamin et al., 2008). The need to constantly generate heat in these species is particularly crucial as their surface to volume ratio is higher compared to large cetaceans, which leads to a larger loss of heat (Manger, 2006). Larger creatures like whales do not face the same challenges as their body is heavier and more efficient at heat conservation. This is the reason resting on the surface is mainly achieved by larger cetacean creatures.
Sleeping on the surface is the closest to familiar mammalian sleep that cetacean achieve. During this time, these marine creatures simply float on the surface with minimal movement and often with one eye open, suggesting USWS. Gray whale calves were observed sleeping on the surface mainly during the night in time lapses of 3 min to 98 min (Lyamin et al., 2001). During this form of sleep, their respiration slows down, and many species become completely quiescent.
USWS and slow swimming have evolved to allow mammalian sleep under aquatic conditions. There are three main hypothesis that explain this unusual form of sleep: it facilitates respiration (Mukhametov & Polyakova, 1981); it is a result from predation pressures (Lilly, 1964); it facilitates heat regulation (Manger, 2006).
Cetaceans exhibit a complex mechanism that prevents water into going into their lungs. If both hemispheres were to fall asleep, respiration would be jeopardized, as the creature needs to provide a “conscious” effort to breathe. In fact, anesthesia inhibits dolphin autonomic breathing. During bilateral SWS, the dolphin fails to breath. Slow swimming, on the other hand, seems necessary to come to the surface and breathe during sleep time, and to maintain proper breathing posture (Lyamin et al., 2008). USWS might then be a response to the challenges of breathing in aquatic environments during sleep.
The most plausible answer to the existence of USWS and slow swimming in cetaceans is the need to regulate their bodies temperature. Marine mammals live in extreme thermal conditions as conductive heat loss in water is 90 times greater than in air. This is particularly true in small cetaceans, where the thermoregulatory challenges are greater due to their high surface to volume ratio (Manger 2006). It is then obvious that constant movement generates heat, which leads to a better temperature regulation. USWS also allows constant production of noradrenalin, which in result keeps muscle tonus and increases glia metabolism (regulates brain temperature) (Fig. 2) (Lyamin et al., 2008).
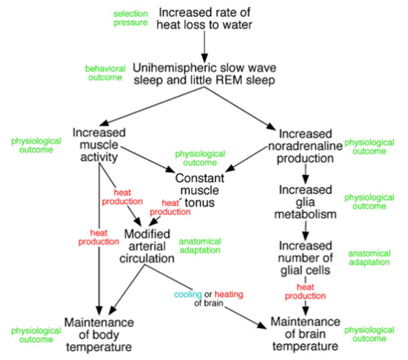
Chimpanzees
Sleep is essential and animals, depending on their morphology, environment, and behaviors, all make sure in their own way that they have a safe and comfortable place to rest. Some animals have a peculiar way of sleeping and thus have complex bed-building behaviors. In fact, they always make sure they have either a nest, a bed or a platform for the nighttime or the day napping. One of those species is the family of chimpanzees. They are impressive with their highly structured community and the technology they can develop with the materials they have to provide as much comfort and protection as possible to the members of their group. One amazing and highly important thing they manage to do is making a secured nest with comfort and thermoregulation out of the simple materials they have under their hands (Stewart et al., 2018).
Studies showed that wild-born and captive-born chimpanzees have different sleeping habits and use techniques that vary in complexity; “the complex techniques (weaving and bending techniques) were executed mostly by wild-born chimpanzees, whereas air-bedding behaviors only occurred among captive-born individuals, especially those born in biomedical facilities” (Yamanashi et al., 2020). Weaving and bending techniques (Fig. 3) provide many advantages for their bed such as thermal insulation properties and pressure distribution (Anderson et al., 2019). This textile crafting when combined with more complex materials can even be used to make mattresses. Thus, depending on the materials chimpanzees choose and weave, they are provided more comfort and even thermoregulation. Weaving is a well-known technique using different kind of yarns that are interlaced together tightly (Cieślak et al., 2021). For apes, the materials they can use to make textile are all naturals and found in their environment. Examples of useful materials for them are grass, liana, and shrub (Stewart et al., 2018).
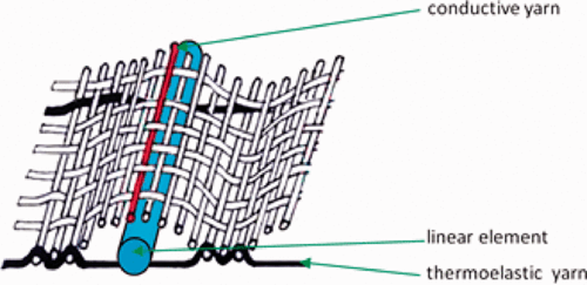
The choice of the material is crucial since it influences the air permeability and the heat transfer of the textile or the surface crafted; “it was observed that the thermal characteristic depends on the raw materials of components, structure, thickness, and mass per unit area of the fabrics” (Cieślak et al., 2021). In the nests of chimpanzees, thermoregulation has been proven to be a significant part of the overall quality of their sleep and weaving textiles are one of the best ways to provide thermoregulation to a nest (Cieślak et al., 2021).
Beds also need to provide a safe place; they need to be protected from the weather changes as well as the predators. To do so, chimpanzees created nest with a structure adapted to the environment in which they sleep. First, they build their nest by taking in consideration the temperature changes and therefore provide insulation to counter it. An experimental study by Stewart et al. (2018) showed that heat loss during a climate change was greater outside than inside a nest. Chimpanzees will also adapt the structure and the materials used for their nest as the weather changes. For example, they adapt the chosen heigh in correlation with the level of humidity of their environment and the number of rainfalls which are depending on the season (Stewart et al., 2018). Apes are known as an endotherm specie or warm blood. Therefore, they “must maintain body temperature within a neutral range while minimizing metabolic costs of doing so” (Verzuh et al., 2021). As mentioned, thermoregulation and insulation in nests can prevent chimpanzees from having to either exceed their neutral body temperature when the weather is warmer or to have to provide heat to themselves by eating more for example. Consequently, the site chosen for the nest must either minimize the heat loss or gain (Verzuh et al., 2021). For high temperatures, the best choices are to locate the nest in an area where shadow is dominant or in an elevated site to balance the heat augmentation. They can also provide cooling during their sleep by convection or conduction if the nest is located in an area where vegetation is scattered or by putting cool or wet materials on the ground or on the platform used as a bed (Verzuh et al., 2021).
Primates either build their nest high in trees or on the ground and the structure of it depends on this choice. Nests are “usually bowl-shaped, oval in shape, with mean length about one fifth longer than width” (Stewart et al., 2018) but ground and tree nest differ from the materials used. High tree nests must support a certain load and therefore have a solid base to rest on. Ground nests obviously do not have this problem and can have a less robust base and focus more on comfort than support. Measurements of different nests show that ground nest are usually smaller and thinner than tree nest by comparison of their dimensions and depth (Fig. 4). A test was made between tree and ground nests and compared their bend support and break support capacities. This test ran by Stewart et al. (2018) showed that those values were lower for the ground nests. This was expected since ground nests are in majority made from grass and other nonwoody materials.
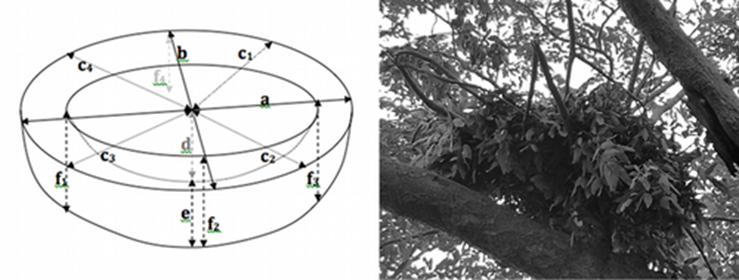
Factors affecting mammalian sleep positions within herbivores
Terrestrial mammals may employ one of several different positions when sleeping or at rest. Among these positions, sternal recumbency and lateral recumbency are among the most widely seen across mammals; the former consists of the animal lying on its sternum, illustrated in Fig 5, while the latter involves the animal lying on its side, as illustrated in Fig. 6. For each mammal, there are several factors that may influence its sleep position. Some of these factors are intuitive. For instance, the general shape of the organism such as relative torso breadth and limb length can impact whether it is feasible to choose one position over the other. In addition, the environmental conditions such as the space available, the presence of supports or substrates on which to sleep on, and the temperature may also impact the choice between lateral and sternal recumbency (Pucora et al., 2019). However, one less intuitive physiological factor for sleep position is the result of the relationship between gravity and the organism's digestive system. Indeed, studies have suggested that the structure and organization of the digestive systems of certain terrestrial mammals affects their primary rest positions (Pucora et al., 2019).
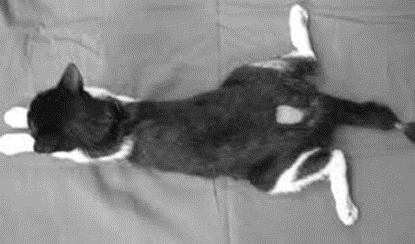
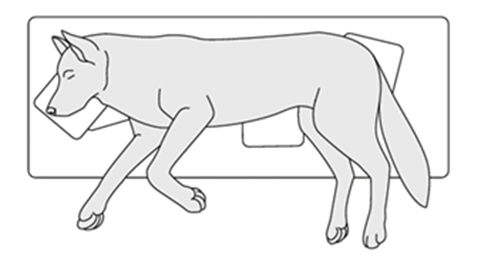
Sleep positions in ruminants
For instance, ruminants are a suborder of herbivores that are characterized by a stomach divided into four compartments which are, in order, the rumen, reticulum, omasum, and abomasum (Swanson, 2019). Thus, ruminants are foregut fermenters, which is to say that fermentation occurs, in the rumen, prior to digestion by the glandular stomach, or the abomasum. Therefore, the rumen and the reticulum, known together as the reticulorumen, form a sorting system to separate foods that necessitate different degrees of digestion; this results in the distinct stratification of material into layers of gas, particles, and fluids in the gut, as illustrated in Fig. 7 (Clauss et al., 2010). As a result of this explicit separation, the ruminant is able to clear the rumen of digested food while continuing to ferment larger particles that require further fermentation, regurgitation or mastication (Clauss et al., 2010). By clearing the rumen, the ruminant can then intake more food.
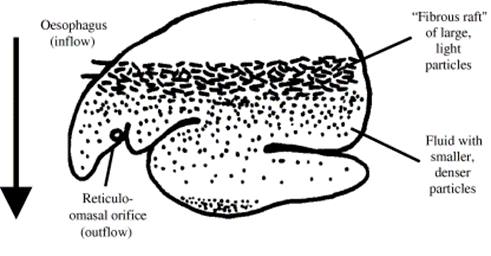
To stratify the food particles, the digestive system uses the gravity vector to form a density gradient: smaller, denser, digested particles sink to the bottom of the rumen where they are passed through the reticulo-omasal orifice, located near the bottom of the reticulum. Eventually, particles passed through this orifice advance to the glandular stomach, while larger, low-density particles float and continue to ferment in the reticulo-rumen. Therefore, to maintain this stratification, and to prevent the premature outflow of large particles through the reticulo-omasal orifice, ruminants are theoretically and practically limited to sternal recumbency when sleeping because they must maintain the orientation of the gut with respect to the gravity vector.
In addition, the restriction to sternal recumbency is not observed in hindgut fermenters such as horses, whose fermentation takes place after the glandular stomach, often in the large intestine or cecum, and which does not usually require stratification (Pucora et al., 2019). This supports the correlation between digestive system and sleep position, as the presence of a sorting mechanism and foregut fermentation is correlated with the animal sleeping in sternal recumbency to maintain the stratification of the digestive system.
Horses
For the majority of their lives, including during portions of their sleep periods, horses are in a standing position. For most species, this amount of standing is impossible due to the continuous effects of the force of gravity, especially significant for larger animals, which eventually fatigues the limb muscles causes joints such as the knee and the stifle to collapse (Hermanson & MacFadden, 1996).
Thus, horses have developed an adaptation to their limbs in order to counteract the muscle fatigue caused by the compression of the limb by the animal's weight and the ground normal force while standing. They employ a mechanism, known as the stay apparatus, which is a combination of ligaments, tendons, and muscles, and allows for significant energy conservation while standing. Notably, in the hind limbs, a crucial phenomenon that occurs is patellar locking in the stifle joint, which is illustrated in Fig 8.
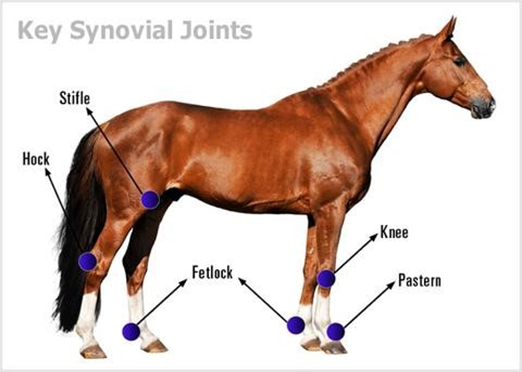
As illustrated in Fig. 9, when the horse activates the stay apparatus, the patella is pulled behind the femoral trochlea; this displacement then prevents the stifle from flexing, which in turn also prevents the hock joint from flexing (Schuurman et al., 2003). The only active muscle in this process is the vastus medialis, which only provides the traction necessary to keep patella in place while locked and does not expend much energy. Thus, this mechanism allows horses to exert over 98% less force than they would if they lacked this evolutionary adaptation since they do not have to expend energy to hold themselves standing.
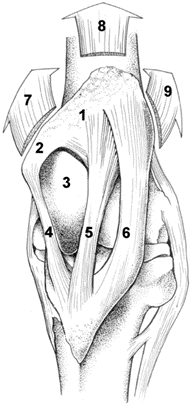
Bats: Their Peculiar Inverted and Upright Sleeping Postures
Of all mammalian species, bats possess an especially distinctive way of sleeping which is generally characterized by their upside-down roosting behavior. Nearly all species of bats (chiroptera) sleep in this manner, with the only exceptions being species of the families Thyropteridae and Myzopodidae which roost upright, often in furled leaves (Riskin & Fenton, 2001). Despite their differences, all bats have evolved unique physical structures to satisfy the mechanics of their peculiar roosting positions including the tendon locking mechanism (TLM) present in many inverted roosting species as well as the suction disks characteristic of upright roosting bats.
Digital Tendon Locking Mechanism
The most prominent mechanism in bats, digital TLM, is comprised of two connected components on the digital tendon which lock the digit in a closed and flexed position (Simmons & Quinn, 1994). This allows the bat to hang inverted for sustained periods when sleeping while inducing minimal muscular strain, thus expending little energy. The first component is a tuberculated fibrocartilage cell patch located on the ventral flexor tendon and the second component consists of a folded area of an adjacent flexor tendon sheath as shown in Fig. 10 (Simmons & Quinn, 1994). The TLM is engaged when the digit is flexed, specifically by pulling proximally on the flexor tendon which settles the tubercules in place with the sheath (Quinn & Baumel, 1993). These components are locked in place as the size of crests in the tubercule patches match the trough sizes of the adjacent folded tendon sheath (Bennett, 1993). Once these two parts are joined, the weight of the suspended chiropteran's body provides tension on the already flexed digits and claws ultimately maintains the locked position (Simmons & Quinn, 1994). A detailed diagram of the digital TLM system can be seen in Fig. 11.
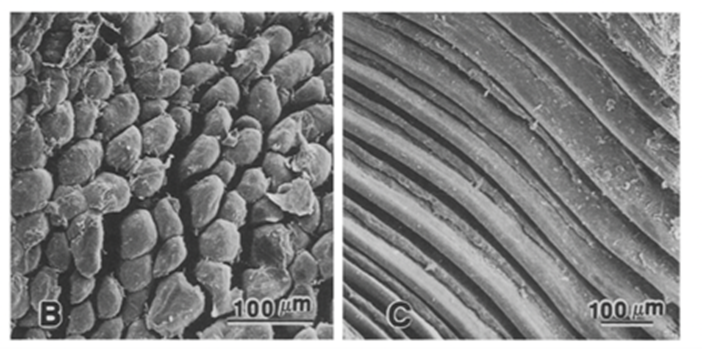
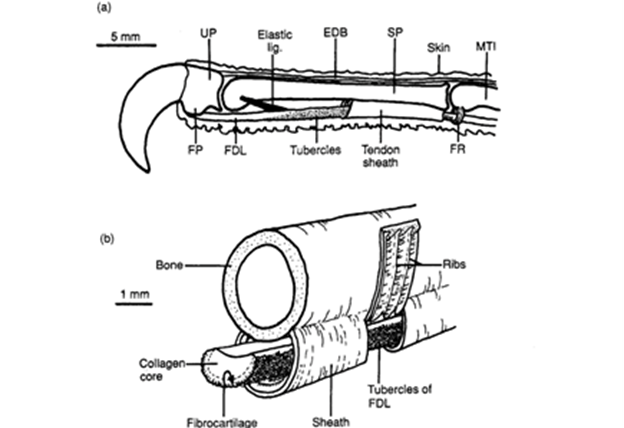
Furthermore, the structure of a hanging bat and the composition of the fibrocartilage tubercules compliment the locking mechanism. The ventral angulation of the bones at the metacarpophalangeal joint also pushes the flexor tendon against the plicated section of the tendon sheath which helps to keep the mechanism locked (Quinn & Baumel, 1993). Observing the fibrocartilage material mechanically, it is a stiffer material than tubercules made of collagen, so this would allow the tubercules to withstand larger forces and prevent deformation of the TLM (Bennett, 1993). Additionally, the locking mechanism is proven to be effective as the body weight of the bat can be supported even with only one limb and no muscular energy since dead bats have been observed to have remained hanging (Bennett 1993).
The reason for the inverted hanging sleeping position of bats and the corresponding digital TLM can be explained by the evolutionary factors and advantages which it provides. One hypothesis is that bat evolution involved the shrinking of limbs to reduce the load during flight causing the legs to withstand less compression over time; therefore, hanging became an advantageous position for roosting (Howell & Pylka, 1977). Additional advantages of this roosting position that have been hypothesized include the facilitation of a quick flight take-off for fleeing (Howell & Pylka, 1977). Interestingly, the evolutionary history of this TLM is rather complex because not all inverted sleeping bats are equipped with this mechanism. Bats lacking TLM are observed to have a thicker annular sheath over the flexor tendon. Friction between the thickened sheath and the tendon may help to restrain the extension of the distal phalanx while the bat is in a hanging position (Simmons & Quinn, 1994).
Upright Roosting Mechanisms
Completely different to the inverted roosting bats are six species of the Thyropteridae and Myzopodidae families, which have evolved disk-like structures at the base of their wrists and ankles that allow them to roost upright by attaching themselves to smooth surfaces. These bats do not possess a TLM as they roost vertically rather than hanging, usually clinging to furled leaves facilitated by either a wet adhesion mechanism, a suction mechanism, or possibly a combination of both (Riskin & Fenton, 2001). Wet adhesion is defined by two solids being connected via an intervening liquid layer, while suction occurs when there is a partial vacuum created between the surface-body area (Riskin & Fenton, 2001). Forces in static opposition to gravity must be applied by these mechanisms to prevent the bat from sliding or falling off the smooth surfaces of leaves while they roost.
For the Thyropteridae tricolor (a species of disk-winged bats), experiments have concluded that suction is the main mechanism allowing the bats to adhere to smooth surfaces, while wet adhesion plays a secondary role (Riskin & Fenton, 2001). The disk's structure is shown in Fig 12. and can be described as a cartilaginous plate connected to tendon and muscle beneath the disk, specifically at the metacarpophalangeal joints of the thumbs and anchylosed digits (Riskin & Fenton, 2001). These disks are concave and always moist to facilitate suction and wet adhesion (Boerma et al., 2019). Elasticity of the disk in T. tricolor is not only important for creating a vacuum under the disk, but suction requires continuous utilization of muscles, so it also works to save energy for the bat (Riskin & Fenton, 2001). The wet adhesion mechanism conserves energy too as it requires no expenditure of energy. It relies on the fact that the two adhering surfaces are so close in proximity that the viscosity of the liquid in between the surfaces prevents their separation (Smith, 1992).
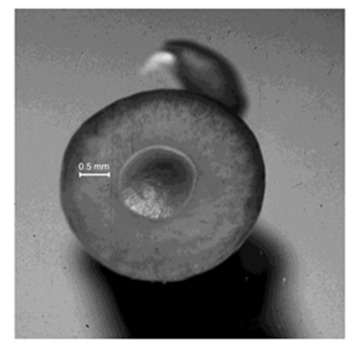
Much like disk-winged bats, sucker-footed bats (Myzopoda aurita) also possess specialized pads. (Fig. 13). Contrary to its name, experimental results suggest that M. aurita use wet adhesion, not suction, as the primary mechanism of roosting upright (Riskin & Racey, 2010). This is concluded since their pads exhibit the typical characteristic of wet adhesion in biological systems, where the adhesive force is much larger parallel to the surface than perpendicular to it (Peattie, 2009). Upright roosting loads the pads in pulling shear where attachment is strongest, whereas, pushing shear in an inverted posture would lead to the passive detachment of the pads (Riskin & Fenton, 2001). This justifies the upright sleeping posture of the sucker-footed bats. Again, wet adhesion does not require work from muscles which is energetically advantageous for these bats.
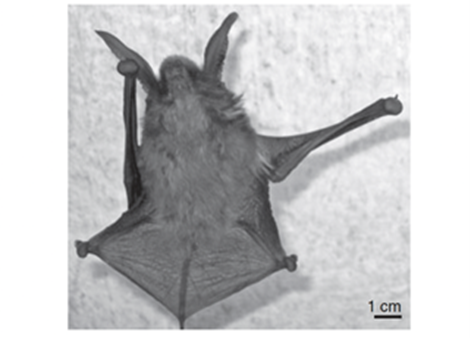
These upright roosting bat species differ drastically from the inverted hanging bats in that they roost in rolled smooth leaves, as shown in Fig. 14. In this case, the entrance to the leaves is from the top, so an upright roosting position proves advantageous when the bat needs to flee quickly from its roosting environment in case of emergency (Riskin & Racey, 2010). Another selective advantage to evolving upright roosting in leaves is the shelter and protection which the leaves provide for the bat (Fenton et al., 1994). Although the inverted and upright roosting bats sleep in opposite positions, the one commonality between their different mechanisms involved is that they are energy conserving devices.
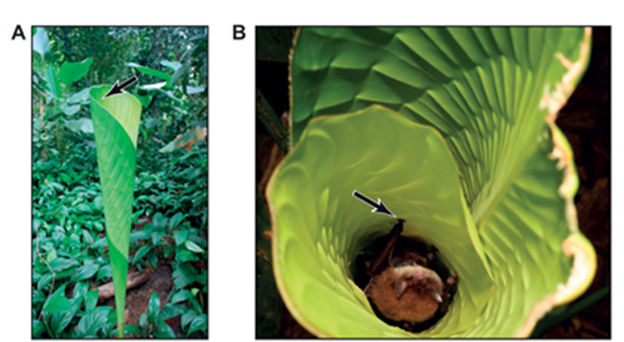
Discussion
Given the necessity of sleep for these animals, it is apparent that they have developed a broad range of adaptations unique to each species' anatomical and behavioural features. The advantages these changes offer can be thermodynamic, such as in cetaceans and chimpanzees, or aid the animal to maintain static equilibrium while accommodating for gravity, such as with bats, horses, ruminants, and flamingos. Thus, this collection of sleep adaptations illustrates the unique solutions that evolution can create as a response to the inevitability of sleep, and that can be modelled as designs used for other purposes.
Conclusion
To summarize, animals have adapted in diverse ways to integrate sleep with their anatomies, environments and behaviours. For instance, flamingos elegantly use their body weight to stand with minimal muscular effort while sleeping. The simplicity of the structure of their legs allows an equilibrium position that doesn't need external forces to maintain. This system might inspire a new generation of mechanical legs or arms. Additionally, due to the challenges of thermoregulation, cetaceans have adopted an unusual form of sleep mainly seen in birds. This function allows them to continue swimming while sleeping, which not only generates heat, but also might be responsible for an efficient respiratory mechanism. Cetaceans brave these difficult thermoregulatory conditions as no other mammal, with minimal effort during sleep. Furthermore, chimpanzees have developed many techniques over the years to make their sleep as undisturbed as possible and to have a comfortable and safe bed. With the few simple materials they have, they can build thermoregulated nest on the ground or high in the trees depending on the localization, the weather, and the predators around. Also, in bats, the physical mechanism and structures ultimately allow for their exceptional sleeping positions. The TLM relies on the fastening of bat tendons suited for inverted hanging bats, while wet adhesion and suction allow some species to remain vertically stationary on smooth surfaces. These vastly different mechanisms all help to reduce the work done by the muscles of a sleeping bat. Moreover, for ruminants, the particularities of their digestive system, notably in the reticulorumen, restricts them to sternal recumbency, to avoid disturbing the delicate equilibrium that exists in the stratification of stomach contents in harmony with gravity. Finally, horses can be found sleeping while standing, a feat that would require tremendous amounts of energy from a different organism. Thus, equids have adapted by developing a stay apparatus that allows them to stand in a near-effortless fashion for long periods of time, with the help of a locking mechanism in the stifle. The evolution of this mechanism is a remarkable feat in nature's engineering with the goal of energy conservation.
References
Anderson, J. R., Ang, M. Y. L., Lock, L. C., & Weiche, I. (2019). Nesting, sleeping, and nighttime behaviors in wild and captive great apes. Primates, 60(4), 321-332. https://doi.org/10.1007/s10329-019-00723-2
Anderson, M. J., Jones, A. G., Schlosnagle, A. P., & King, M. L. (2018). Examining unihemispheric sleep and its potential relation to lateral resting behaviour and unipedal resting stance in Caribbean Flamingos. Avian Biology Research, 11(2), 74-79. https://doi.org/https://doi.org/10.3184/175815618X15204318491767
Bennett, M. B. (1993). Structural modifications involved in the fore- and hind limb grip of some flying foxes (Chiroptera: Pteropodidae). Journal of Zoology, 229(2), 237-248. https://doi.org/https://doi.org/10.1111/j.1469-7998.1993.tb02633.x
Boerma, D. B., Barrantes, J. P., Chung, C., Chaverri, G., & Swartz, S. M. (2019). Specialized landing maneuvers in Spix's disk-winged bats (Thyroptera tricolor) reveal linkage between roosting ecology and landing biomechanics. J Exp Biol, 222(Pt 20). https://doi.org/10.1242/jeb.204024
Chang, Y.-H., & Ting, L. H. (2017). Mechanical evidence that flamingos can support their body on one leg with little active muscular force. Biology Letters, 13(5), 20160948. https://doi.org/doi:10.1098/rsbl.2016.0948
Cieślak, M., Gromadzińska, E., Kamińska, I., Witczak, E., & Śledzińska, K. (2021). The impact of textile relief structures on pressure distribution and heat transport. Textile Research Journal, 91(9-10), 1009-1019. https://doi.org/10.1177/0040517520970170
Clauss, M. (2004). The potential interplay of posture, digestive anatomy, density of ingesta and gravity in mammalian herbivores: why sloths do not rest upside down. Mammal Review, 34(3), 241-245. https://doi.org/https://doi.org/10.1111/j.1365-2907.2004.00039.x
Clauss, M., Hume, I. D., & Hummel, J. (2010). Evolutionary adaptations of ruminants and their potential relevance for modern production systems. Animal, 4(7), 979-992. https://doi.org/10.1017/s1751731110000388
Cliffe, R., Avey-Arroyo, J., Arroyo, F., Holton, M., & Wilson, R. (2014). Mitigating the squash effect: Sloths breathe easily upside down. Biology Letters, 10, 20140172. https://doi.org/10.1098/rsbl.2014.0172
DeRossi, R., Benites, A. P., Ferreira, J. Z., Neto, J. M., & Hermeto, L. C. (2009). Effects of lumbosacral epidural ketamine and lidocaine in xylazine-sedated cats. J S Afr Vet Assoc, 80(2), 79-83. https://doi.org/10.4102/jsava.v80i2.175
Fenton, M. B., Rautenbach, I. L., Smith, S. E., Swanepoel, C. M., Grosell, J., & van Jaarsveld, J. (1994). Raptors and bats: threats and opportunities. Animal Behaviour, 48(1), 9-18. https://doi.org/https://doi.org/10.1006/anbe.1994.1207
Ford, J. K. B. (2009). K – Killer Whale: Orcinus orca. In W. F. Perrin, B. Würsig, & J. G. M. Thewissen (Eds.), Encyclopedia of Marine Mammals (Second Edition) (pp. 650-657). Academic Press. https://doi.org/https://doi.org/10.1016/B978-0-12-373553-9.00150-4
Hermanson, J. W., & MacFadden, B. J. (1996). Evolutionary and Functional Morphology of the Knee in Fossil and Extant Horses (Equidae). Journal of Vertebrate Paleontology, 16(2), 349-357. http://www.jstor.org/stable/4523724
Horse Anatomy. (n.d.). Mobility Health. Retrieved October 6, 2021, from https://mobility-health.com/pages/horse-anatomy
Howell, D. J., & Pylka, J. (1977). Why bats hang upside down: a biomechanical hypothesis. J Theor Biol, 69(4), 625-631. https://doi.org/10.1016/0022-5193(77)90371-x
Lilly, J. C. (1964). Animals in aquatic environments: adaptation of mammals to the ocean. Handbook of Physiology–Environment, 741-747.
Lyamin, O., Mukhametov, L., Siegel, J., Manger, P., & Shpak, O. (2001). Resting behavior in a rehabilitating gray whale calf. Aquat. Mamm., 27.
Lyamin, O. I., Manger, P. R., Ridgway, S. H., Mukhametov, L. M., & Siegel, J. M. (2008). Cetacean sleep: an unusual form of mammalian sleep. Neurosci Biobehav Rev, 32(8), 1451-1484. https://doi.org/10.1016/j.neubiorev.2008.05.023
Manger, P. R. (2006). An examination of cetacean brain structure with a novel hypothesis correlating thermogenesis to the evolution of a big brain. Biol Rev Camb Philos Soc, 81(2), 293-338. https://doi.org/10.1017/s1464793106007019
Mossor, A. M., Austin, B. L., Avey-Arroyo, J. A., & Butcher, M. T. (2020). A Horse of a Different Color?: Tensile Strength and Elasticity of Sloth Flexor Tendons. Integr Org Biol, 2(1), obaa032. https://doi.org/10.1093/iob/obaa032
Mukhametov, L. M. (1984). Sleep in marine mammals. Exp Brain Res, 8, 227-238.
Mukhametov, L. M. (1995). Paradoxical sleep peculiarities in aquatic mammals. Sleep Res. A, 24, 202.
Mukhametov, L. M., & Polyakova, I. G. (1981). Egg investigation of the sleep in porpoises (phocoena-phocoena). ZHURNAL VYSSHEI NERVNOI DEYATELNOSTI IMENI IP PAVLOVA, 31(2), 333-339.
Nicolau, M. C., Akaârir, M., Gamundí, A., González, J., & Rial, R. V. (2000). Why we sleep: the evolutionary pathway to the mammalian sleep. Prog Neurobiol, 62(4), 379-406. https://doi.org/10.1016/s0301-0082(00)00013-7
Peattie, A. M. (2009). Functional demands of dynamic biological adhesion: an integrative approach. J Comp Physiol B, 179(3), 231-239. https://doi.org/10.1007/s00360-008-0310-8
Peirone, B. (2015). Lateral recumbency position. Surgery Reference. Retrieved September 29, 2021, from https://surgeryreference.aofoundation.org/vet/dog/femoral-shaft/preparation/lateral-recumbency-position
Pucora, E., Schiffmann, C., & Clauss, M. (2019). Resting postures in terrestrial mammalian herbivores. Journal of Mammalogy, 100, 1-12. https://doi.org/10.1093/jmammal/gyz044
Quinn, T. H., & Baumel, J. J. (1993). Chiropteran tendon locking mechanism. J Morphol, 216(2), 197-208. https://doi.org/10.1002/jmor.1052160207
Riskin, D. K., & Fenton, M. B. (2001). Sticking ability in Spix's disk-winged bat, Thyroptera tricolor (Microchiroptera: Thyropteridae). Canadian Journal of Zoology, 79(12), 2261-2267.
Riskin, D. K., & Racey, P. A. (2010). How do sucker-footed bats hold on, and why do they roost head-up? Biological Journal of the Linnean Society, 99(2), 233-240.
Schuurman, S. O., Kersten, W., & Weijs, W. A. (2003). The equine hind limb is actively stabilized during standing. Journal of anatomy, 202(4), 355-362.
Simmons, N. B., & Quinn, T. H. (1994). Evolution of the digital tendon locking mechanism in bats and dermopterans: a phylogenetic perspective. Journal of Mammalian Evolution, 2(4), 231-254.
Smith, A. M. (1992). Alternation between attachment mechanisms by limpets in the field. Journal of Experimental Marine Biology and Ecology, 160(2), 205-220.
Stewart, F. A., Piel, A. K., Azkarate, J. C., & Pruetz, J. D. (2018). Savanna chimpanzees adjust sleeping nest architecture in response to local weather conditions. American Journal of Physical Anthropology, 166(3), 549-562.
Swanson, K. C. (2019). Small Intestinal Anatomy, Physiology, and Digestion in Ruminants☆. In Reference Module in Food Science. Elsevier. https://doi.org/https://doi.org/10.1016/B978-0-08-100596-5.22638-3
Verzuh, T. L., Hall, L. E., Cufaude, T., Knox, L., Class, C., & Monteith, K. L. (2021). Behavioural flexibility in a heat-sensitive endotherm: the role of bed sites as thermal refuges. Animal Behaviour, 178, 77-86.
Yamanashi, Y., Bando, H., Matsunaga, M., Tanaka, M., Nogami, E., & Hirata, S. (2020). Development of bed-building behaviors in captive chimpanzees (Pan troglodytes): Implication for critical period hypothesis and captive management. Primates, 61(5), 639-646.