Bioadhesives for Animal Construction in Nature: A Collection of Case Studies
Yuheng Liu, Berine Wehbeh, Sara Fraser, Adi Orlov
Abstract
From adhesive pads used by reptiles to climb against gravity, secretion from birds to construct their nests, to adhesions used by spiders to catch prey, animals in nature have evolved many unique methods of adhesion long before the human industry. It is the application of the adhesive techniques that enabled some animals to survive in a world built on “survival of the fittest.” This essay compiles different animal homes and the chemistry behind the various well-designed mechanisms of adhesion. In nature, animals use an extensive selection of adhesive techniques with complex implementation of chemistry, and much can be learned by investigating these naturally evolved adhesive techniques optimized for each of their unique habitats.
Introduction
Adhesion is the physical attraction or binding of two dissimilar substances that is caused by mechanical interlocking, physical bonding, or chemical bonding. In nature, various animals have developed abilities to produce adhesives to construct their homes, escape from predators, find food, and travel against gravity or inertia. Through the course of evolution, these animals have developed intricate and efficient methods of producing adhesives. For example, different types of spiders use different adhesives to capture prey through webs and protect their eggs by wrapping silk around them (Römer & Scheibel, 2008). Beehives are constructed with adhesive propolis or “bee glue”, which is manufactured by bees (Saccardi et al., 2021), while wasps secret special saliva to reinforce their nests by employing adhesives (Schmolz et al., 2000). Caddisfly larvae use adhesive silk filaments to build their portable protective casing (Wang et al., 2015), and sandcastle worms mix granular material from the environment with their secreted adhesive to build a sessile shelter structure (Stewart et al., 2017). This paper aims to highlight the complex chemical properties used in these fascinating animal architectures. Correspondingly, this essay attempts to explain these different mechanisms of adhesion with various aspects and topics from chemistry.
Spider Webs
Spiders, the largest order of arachnids, are found in nearly every habitat on every continent except Antarctica (Zschokke & Logunov, 2010). Their home, the spider web, is made of spider silk. It is manufactured within the spider's major ampullate gland (Blamires, 2019). The silk proteins are first secreted as a concentrated liquid crystalline solution. Then, by changing the pH level and salt concentration during the fibre spinning process, the proteins fold into various secondary structures. Lastly, the gel-like proteins flow through a narrowing duct. The shear stress generated by the duct then results in further folding of the proteins, which leads to the formation of spider silk.
The silk has evolved to have unique chemical properties to help with several applications such as protection for their eggs, escape from predators and capture prey. Although a thread from spiderweb, about 5 micrometers, is more than 10 times thinner than human hair, it has strong adhesive properties and a tensile strength comparable to high-grade alloy (Römer & Scheibel, 2008). Furthermore, different spiders use different adhesion methods. This section will discuss different silk fibres, cribellate and ecribellate, that are produced by two different types of orb-weaver spiders.
Orb-weaver spiders are the most common spiral wheel-shaped builders. Like most spiders, they produce various types of silks that are designed to perform different functions. For example, dragline silk makes the radii of the web, ampullate silk accompanies dragline silk, and flagelliform silk forms the core filament of the capture threads. There are two types of capture threads used by orb-weaver spiders: cribellate silk and ecribellate silk (Fig. 1). Cribellate silk is usually used by web spinning spiders. Ecribellate silk is evolved more recently and used by most modern orb-weaving spiders (Sahni et al., 2011).

Structure of a Spider Web
Silk fibres from a spider web primarily consist of proteins that are made of nonpolar and hydrophobic amino acids. Additionally, the protein's core domain features highly repetitive sequences (Fig. 2). These long repetitive sequences can produce weak but numerous intramolecular and intermolecular interactions between neighboring domains, thus resulting in the formation of secondary, tertiary, and quaternary structure (Römer & Scheibel, 2008). The nonrepetitive regions are located in the protein's termini. These regions, which show well-defined secondary and tertiary structures in solution, are crucial for assembling silk proteins into fibres. They can induce intermolecular disulfide bonds and stabilize dimers and multimers, thus initiating the production of silk fibres from proteins (Römer & Scheibel, 2008). In the quaternary structure of silk proteins, areas of high electron density are embedded in areas of low electron density. It is these high electron density regions that enable silk fibre's strong mechanical strength. Similarly, the low electron density regions are responsible for the silk's elasticity. Upon tensile loading, these regions characterized with few secondary or supersecondary structures can deform partially like a protein hydrogel, increasing their resilience and flexibility (Hayashi et al., 1999).
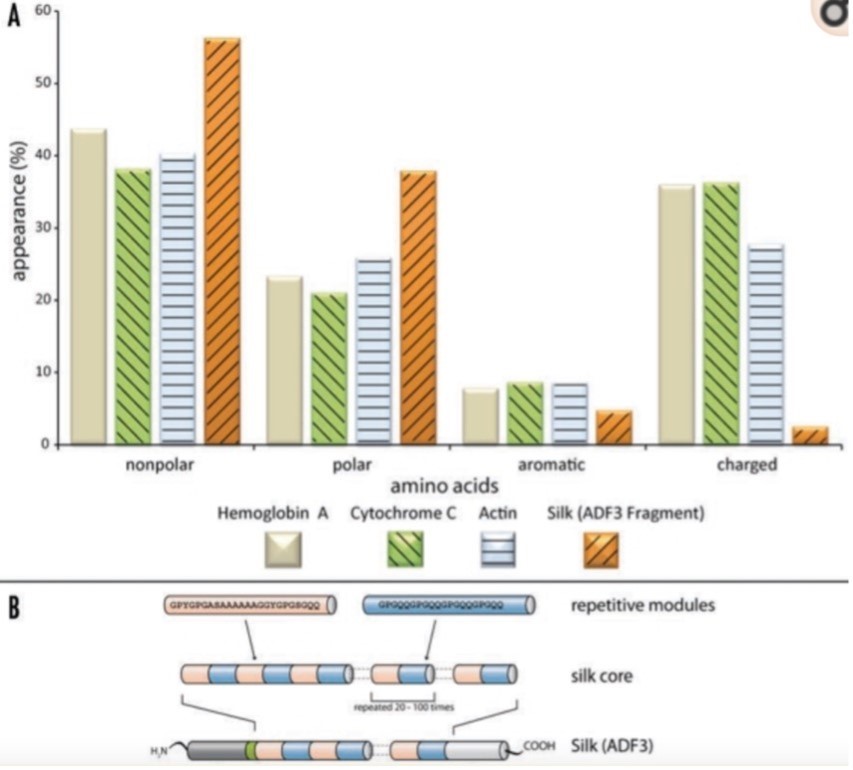
Adhesive properties of a Spider Web
In order for the spiders to hunt for food, capture prey, escape from predators and protect their eggs, spider's silk fibre has evolved significantly and developed various adhesive techniques for defense, locomotion, and capturing prey. For the orb-weaver spiders, their silk evolved from cribellate silk to ecribellate silk. Cribellate silk is spun laboriously by the spider's cribellum and calamistrum (Fig. 3). It uses a mesh of thin, dry nanofibers around its axial threat to achieve adhesion. However, this method is very time and energy consuming for the spiders, and ecribellate spiders have evolved a new method of making silk. These modern web-weavers use an aqueous based glue on their silk fibres to achieve adhesion (Sahni et al., 2011). Thus, a major difference between these two methods is that cribellate silk uses dry-adhesion and ecribellate silk uses wet-adhesion.

Cribellate Silk
A couple adhesion mechanisms are used for the cribellate silk. Firstly, physical interlocking retains the captured prey like Velcro as the nanofibers on the spider thread's surface snag onto the insect (Sahni et al., 2011). Furthermore, due to the relatively large surface area provided by nanofibers arranging in puffs and intermediate zones, van der Waals forces are created to enhance the silk's adhesion (Bott et al., 2017). This is the reason why the fibre structure also adheres to smooth surfaces such as glass and graphite.
Ecribellate Silk
Instead of using cribellum, ecribellate spiders use an aqueous solution that forms droplets on the capture fibres. By using nuclear magnetic resonance (NMR) spectroscopy, a study found these glue droplets contain a concentrated solution of hygroscopic components such as GAB-amide, choline and betaine. Interestingly, the water-soluble fraction does not contain any polymer. In fact, glycoproteins are presented in the inner drops, and they are the primary reason for ecribellate fibre's adhesive properties (Fig. 4) (Sahni et al., 2011).Due to the sufficiently long side chains of glycoproteins, the polymers act as the adhesive glue. Furthermore, these glycoproteins also make the droplets viscoelastic. At slow extension rates like the movements of trapped insects, glycoproteins deform similar to a rubber band and hold onto the trapped insects for a long period of time. At high extension rates, the adhesive forces are enhanced significantly due to the high viscous effects. This makes the capturing web hold on to fast-flying insects easier when they impact the spider web (Sahni et al., 2011).

Besides spiders, another order of insects that make use of adhesives in the construction of their homes is Hymenoptera, which includes bees and wasps.
Hymenoptera Homes
Often considered to be parasitic, Hymenopterans are an order of Arthropoda insects that ranges from ants to sawflies. While most animals display a certain level of ingenuity when it comes to building their homes, this specific order is distinguished by the intricate chemical properties and planning that go into making the structures they reside in. This can be observed in their persistent use of adhesives to reinforce these architectural masterpieces. In this section, the focus will be on two specific types of Hymenoptera: bees and wasps. These two groups of insects can also be further classified into smaller families, including honeybees, bumblebees, and leafcutter bees for the former and paper wasps and hornets for the latter. Due to this variety in bee and wasp species, it is normal – and expected – to find discrepancies in the homes built by the different groupings. However, there is a certain degree of uniformity in beehives and wasp nests (Fig. 5). The following subsections will discuss the major types of adhesives utilized by the Hymenoptera classes in the process of constructing and maintaining their homes (Schmolz et al., 2000).

Beehives
Naturally, beehives may be the first example that crosses one's mind when thinking about animals and adhesives. They contain a substance called propolis, often referred to as ‘bee glue'. As the name implies, propolis is a type of adhesive, a sticky material that various bees produce in order to properly shut out outside threats, such as harmful microbes, from their homes (Saccardi et al., 2021). It is mostly used by honeybees to seal potentially problematic holes in the beehive, i.e., openings that are not meant to be there. Moreover, it also plays a role in regulating the heat and moisture levels inside the hive (Huang et al., 2014).
Composition of Propolis: In general, propolis is reported to be exactly half plant resin. The other half is made up of wax (30%), different kinds of oils (10%), and pollen (5%), with the last 5% distributed between various organic compounds (Huang et al., 2014; Saccardi et al., 2021). Further delving into the chemical composition of this adhesive illustrates that more than 300 individual compounds were identified in different propolis samples, out of which at least 112 were flavonoids. These mostly consist of flavones, chalcones, dihydrochalcones, and isoflavones among many others. While flavonoids may be the most characteristic components, the compounds in propolis with the highest abundancy are sesquiterpenes (Fig. 6). These belong to a broader class of chemicals called terpenes, of which monoterpenes are also found in bee glue. The other major constituents are phenolics, sugars, and hydrocarbons (Huang et al., 2014). The discrepancy in composition can be attributed to the fact that the resin component of this substance varies greatly depending on the tree it was extracted from.
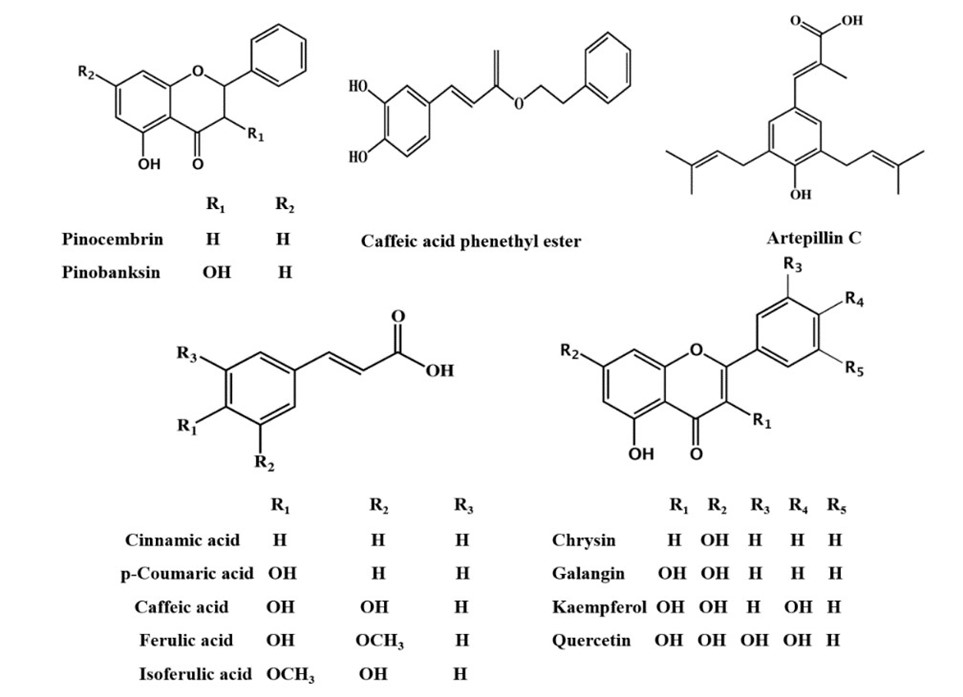
Plant Source Selection: For the most part, bees extract the resin they require for propolis synthesis from the bud of their tree of choice. As resin is quite a substantial constituent of bee glue, the exact kind will have a notable impact on the product. Resin acquisition is naturally influenced by the available flora in a given region, as bees will seek to adapt to the environmental conditions. The trees most commonly associated with propolis resin are the alder, ash, aspen, beech, birch, elm, horse-chestnut, oak, pine, and poplar trees. Different types of bees utilize vastly different plant sources, sometimes sampling multiple ones in the same propolis batch.A 2016 study by Isidorov et al. treated samples of propolis with diethyl etherand pyridine to determine which buds were favoured by European bees in their selection process (Fig. 7). These were then compared to bud resin samples from different sources. Despite having a wide variety of trees at their disposal, the experiment indicated that bees tend to resort to specific species of poplin, birch, and aspen trees for resin. This was determined by matching the chemical components found in propolis to those of the known bud resins. Further analysis suggests that this is due to the ability of different resins to shield the hive from microorganisms (Isidorov et al., 2016).

Adhesive Properties: The resin utilized by the bees undergoes such an extensive selection process to ensure that the propolis meets the demands of the hive. The most prominent of said requirements is the ability of propolis to act as an adhesive. In fact, bee glue has an unmatched ability to adhere strongly to a variety of surfaces and withstand substantial stress from unideal conditions (Fig. 8). Propolis adhesion to glass, typically reported to be around 3 J/m2, might decrease when submerged in fluids, but still does not cede. This trait is rarely observed in industrial glues, thus enhancing the uniqueness of bee glue. Said substance can also preserve its adhesion to a multitude of substrates despite experiencing significant changes in temperature (Saccardi et al., 2021). All the characteristics mentioned above contribute to the protection of the beehive. They also match the requirements for adhesives in the biomedical field, resulting in the frequent usage of propolis in bioengineering ventures.

Propolis and Biomedical Engineering: For bees, propolis serves as an adhesive that seals their hive and contributes to thermoregulation, moisture control, and social immunity. However, the fact that it is rich in antifungal, anti-inflammatory, antibacterial and antiviral properties makes it a subject of interest for humans too. These attributes allow propolis to have an extensive role in the pharmacology field. It is thus used for a wide range of medical treatments, such as wound-care and preservation, and potentially as a glue for bioengineering application. (Saccardi et al., 2021). Furthermore, electrospun polyurethane nanofibers laced with propolis were analyzed using a variety of tools: infrared spectroscopy, electron microscopy, and thermal gravimetric analysis, among others. The tests concluded that propolis likely increased the cell-compatibility of these fibers (Kim et al., 2014). Consequently, the aforementioned traits allow propolis to ameliorate the biocompatibility of materials and can prove to be a gamechanger for bioengineering, particularly in the field of tissue engineering.
Wasp Nests
Role of Adhesives in the Making of Wasp Nests: While wasps build vastly different homes than their cousins the bees, they too employ adhesives to reinforce the structure of their nests. Typically, these insects construct paper nests, whose primary material is dead wood. The name can be attributed to the paper-like texture of the structure that results from the fibers gathered from rotten wood and plant stems. Nonetheless, these fibers alone are insufficient to properly assemble the nest, and so adhesives are used. The wasps use their own saliva, notably a chitin adjacent substance, to bind the components together. Unlike bees, wasps do not gather materials to make an adhesive, they secrete it themselves. The mucoproteins in the spittle, added to the mixture as the insects are chewing the wood, act as a glue of sorts. As the nest dries, the saliva hardens, binding the fibers together and behaving like an adhesive (Schmolz et al., 2000).
It is interesting to note the parallelism between wasp nests and those of mud nester birds, as the latter use saliva as a binding agent in their homes too. Similarly, the use of adhesives in shelter-building is not limited to Hymenoptera only, as this can also be observed in other insects such as the larvae of caddisflies.
Caddisfly larvae
Protecting vulnerable offspring is a challenge faced by all animals. Caddisflies are the largest aquatic insects (Tsukada et al., 2010) and they have evolved a very unusual strategy to protect their larvae. These larvae build a protective casing out of any suitable materials they can find in their environment, sticking them to their soft body using adhesive silk filaments (Wang et al., 2015). Figure 9 shows examples of these casings using materials from different aquatic environments.
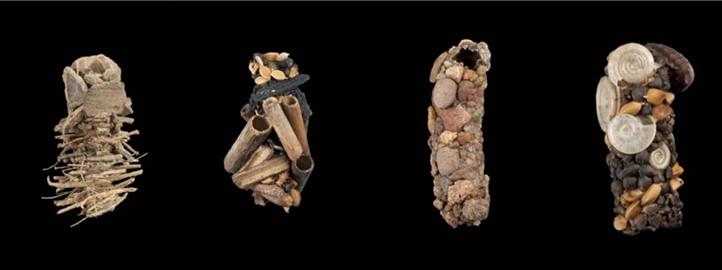
The aquatic environment imposes certain challenges; for example, the adhesive filaments must be insoluble in water (Bhagat et al., 2016). However, this strategy also has advantages as it allows the larvae to protect themselves without having to spend energy creating an exoskeleton early in their life cycle (Wang et al., 2015). The use of materials from the environment also constitutes the most effective form of camouflage.
Core Silk Fiber
Caddisflies are found in freshwater habitats such as lakes and creeks, where they have many predators and can be tossed around by moving currents. Therefore, their silk fibers must be strong to hold their protective casing together (Wang et al., 2015). The filaments are made of double-stranded protein fibers with thin longitudinal striations. The macrostructure can be observed using a scanning electron microscope (Fig. 10) (Tsukada et al., 2010).
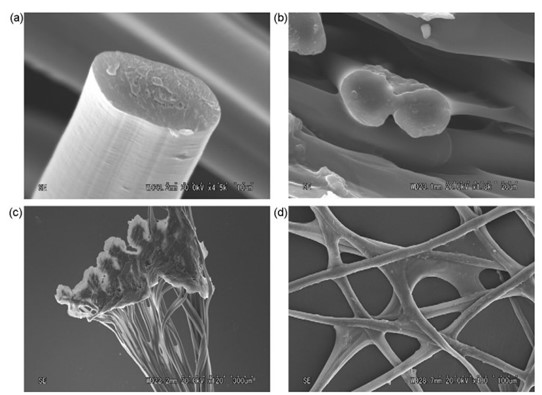
The fiber core is made primarily of H-fibroin polymers (Wang et al., 2015) containing phosphoserine and valine amino acids which are strengthened by ions such as Ca2+ and Mg2+. This is due to strong electrostatic interactions between these cations and the negatively charged phosphate groups. The resulting polymer is water insoluble, which is important for a material designed to be used underwater (Bhagat et al., 2016). This tough fiber has strain energy dissipating properties and non-linear viscoelasticity allowing it to return to its initial state after experiencing tensile deformation. Specifically, cross-linked calcium ions with phosphoserine unfold when strained, increasing the amount of bearable strain, like a slinky (Wang et al., 2015).
Mechanism of Adhesion
When one wishes to build a shelter by affixing many heterogenous materials to one's body, an important design question arises: how does one create a material capable of adhering to surfaces with different properties, such as shells, pebbles, plant matter and tree bark pieces? The answer lies in humic acid and other surface-active polyphenolic compounds. They are produced from the breakdown of plant materials, and are abundant in natural waters, coating every submerged object. Therefore, the silk must only adhere to these substances in order to stick to any wet object. The peripheral layer of caddisfly larva filaments is made of glycoproteins that contribute to underwater adhesion as shown by the periodic acid-Schiff (PAS) stain assay in Figure 11 (Wang et al., 2015).

One of these glycoproteins is the enzyme peroxinectin (csPxt), which catalyzes dityrosine cross-linking between itself and the peripheral layer of the filament. Additionally, H-fibroin (which is the main component of the fiber core) is a candidate substrate of csPxt, so this enzyme also cross-links the peripheral layer to the fiber core (Fig. 12). This stabilizes the entire structure and helps it remain insoluble in water (Wang et al., 2015).
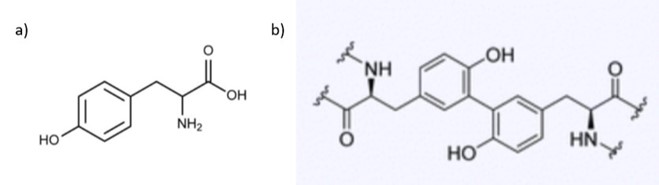
Most importantly, csPxt catalyzes dityrosine cross-linking between silk and the previously mentioned polyphenolic compounds that coat materials in natural water. This is how the larvae can stick to any submerged surface. Another enzyme in the peripheral layer, superoxide dismutase 3 (SOD3), catalyzes the conversion of reactive oxygen species (ROS) in water into H₂O₂, which is needed by csPxt to accomplish its task (Fig. 13) (Wang et al., 2015).
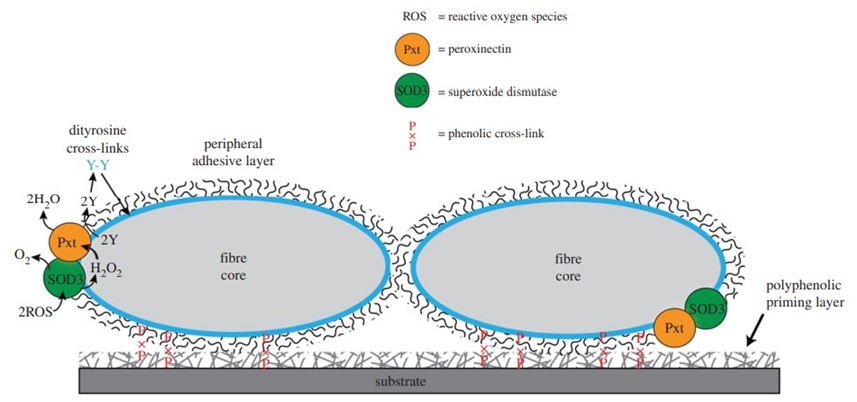
This strategy is cheap, as caddisfly larvae must only produce silk from internal glands and expel it through spinnerets. Then, the outer adhesive layer will cross-link with water-submerged materials, allowing the larvae to assemble a protective casing out of recycled materials in the environment (Wang et al., 2015). Research is ongoing to develop bio-inspired adhesives like the ones used by caddisflies in applications such as surgical glue, dentistry, and water cements (Tsukada et al., 2010). However, caddisflies are not the only animals to employ adhesives underwater: sandcastle worms also have strategies of their own to build aquatic shelters.
Sandcastle Worms
Phragmatopoma californica, also commonly referred to as sandcastle worms, can be found along the western coast of North America and are part of the marine Sabellariidae polychaetes family. These worms are known for their construction of reefs (as seen in Fig 14) that allow entrapment and purification of sediment as well as a home for other living species. The process of building such maritime structures requires the invertebrates to build a tubular shell around themselves while collecting grains of sand or shell fragments of adequate size with their ciliated tentacles and eventually mixing it with their unique bioadhesive, characterized by its separation of opposite charges into separate macromolecules, known as complex coacervation, all while underwater (Stewart et al., 2017). These tubular shells also serve as their home and therefore are of the utmost importance for their survival. How they manage to build such homes, as well as the elements that play a role in this phenomenon, will be discussed in the following sections.
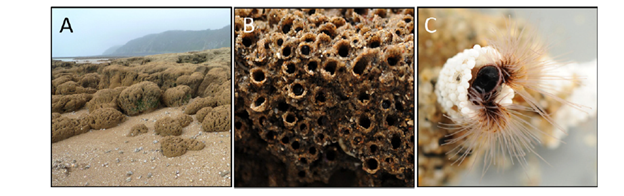
Secretion Components
Before investigating Phragmatopoma californica'ssecretion pathway that builds its honeycomb-like tubular shells, the unique components that permit its construction must be considered. As mentioned previously, these worms collect raw materials of approximately 0.5 mm in size from their environment, such as sand granules and pieces of shells, with the help of the beating of their ciliated tentacles (Fig 14. C) before mixing it with their unique bioadhesive that they secrete (Stewart et al., 2017). This glue's particularity is that it is kept in a complex coacervation state: oppositely charged macromolecules are packaged into two different types of granules, either homogeneous or heterogeneous, and secreted at different stages which with minimal blending can mix and enlarge to eventually become a solid adhesive. Figure 15 describes the abundance of each protein in sandcastle worms based on their relative expression level.

The unique components of the homogenous granules are polyanions in the form of sulphated polysaccharides, which are polymers of monosaccharide chains that contain the emerging negative sulphate groups, and polycation proteins known as Pc2 and Pc5 (Stewart et al., 2017). Conversely, unique members of the heterogeneous granules consist of a magnesium ion, a polyanion Pc3B, which consists entirely of 6-12 phosphoserine blocks separated by single tyrosine residues, polycation proteins known as Pc1 and Pc4, and a polyampholytic protein which has both polycation and polyanion blocks known as Pc3A, which consists of the N-terminus end rich in 4-6 phosphoserine separated by a tyrosine residue and C-terminus end rich in arginine, lysine and cysteine (Stewart et al., 2017; Wang & Stewart, 2012). Pc1 and Pc2 are highly repetitive and contain a significant amount of glycine while Pc4 and Pc5 may be composed of multiple residues, such as lysine, histidine, glycine and tyrosine (Stewart et al., 2017; Wang & Stewart, 2012). All these proteins are used as a collective in the regulation process of the secretion of glue and its subsequent hardening. The exact way these proteins interact with each other remains ambiguous, however it is known that they are responsible for the creation of DOPA and the walls of the solid glue, which will be covered in-depth in the next section (Stewart et al., 2017).
The tyrosine residues observed in the polycation proteins Pc1 and Pc2 will be hydroxylated to form 3,4-dihydroxyphenyl-L-alanine (DOPA) during the secretion pathway process with the help of tyrosinase, an enzyme co-secreted with the granules (Fig. 16) (Wang & Stewart, 2012). Catechol oxidase, an enzyme, is found in both types of granules and is used to covalently cross-link the DOPA sidechains together by oxidizing them into dopaquinones, eventually leading to the final structure of the solid adhesive (Stewart et al., 2017; Wang & Stewart, 2012). The DOPA structure is essential for the fundamental properties of the glue: it provides cohesiveness through cross-linking, where the creation of permanent covalent linkage occurs and mimics the effects of “curing” which produces a toughening of material, and stickiness through chemisorption, which stick the adhesive protein to surfaces (Sun et al., 2009). How the components interact together to produce tubular shells involves a multistep passage that includes the release of the adhesive and the solidification mechanisms, which will be examined in the next sections.

Secretion Pathway
The secretion process begins at the invertebrate's building organ which consists of a pair of finger-like projections just below the mouth (Fig. 17). It is here that the organ decides upon the suitability of raw materials such as sand granules or shell pieces brought up by the ciliated tentacles present on the body by considering the size, shape, and surface chemistry (Stewart et al., 2017). After this consideration, they are held up by these finger-like limbs at the end of the tube and held in such a way as to minimize spaces between what will become the following piece to that tubular shell. An electrical signal is then sent to the worm to release the adhesive through its microscopic pores situated on the ciliated surface of the sandworm. These pores each release their own set of intact granules, however, they seem to be associated in pairs to allow a consistent release of both homogeneous and heterogeneous granules (Stewart et al., 2017).
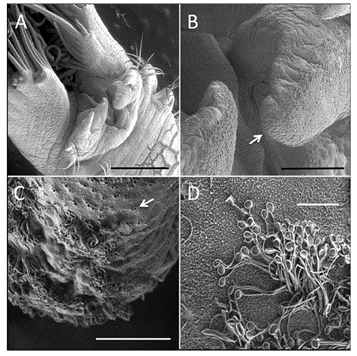
The granules must then rupture to allow the contents of both sets to mix and form the liquid adhesive. Much research has been performed to find what exactly allows the contents to mix. According to Stewart et al.'s (2017) research, it is likely that the granules rupture due to the mechanical force exerted by the worm. The cilia close to the pores on the body of the worm in close proximity to the building organ could cause a mechanical rupture of granules due to its beating (Stewart et al., 2017). Furthermore, when the Phragmatopoma californica use the adhesive to connect the materials, they perform an alternating twisting motion mechanism that can also cause the mechanical rupture of the granules. When the granules rupture, they largely remain intact while only a small amount mixes and fuses with the other granules proving that the shell of the granules is in fact used in the final product of the adhesive and will be connected during the cross-linking of the DOPA (Fig. 18).
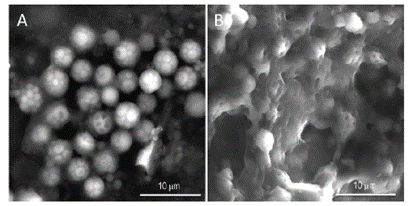
The building organ applies the contents of the mixed granules to the narrow gaps that separate the two successive blocks, effectively gluing them together. Figure 19 shows a model of how these creatures build tubular shells. Just like any other glue, for it to be effective it must solidify rapidly after being used. When this transition from liquid to solid occurs, the Mg2+ and polyanion Pc3 B swell and become the walls of the solid glue (Stewart et al., 2017). The solidification process is triggered by the contact of mixed granules with seawater. The drastic change from pH < 6 in the secretion pathway where the granules are stored and pH > 8 of the sea as well as the change in ionic composition to a ratio of 4:1 Mg2+: Ca2+ of the environment causes this initial hardening due to the insolubilization of the coacervated contents within the granules (Stewart et al., 2017). Additional research has shown that Ca2+ and Mg2+ ions play an important role in the overall stability of the adhesive structure; complete removal of these ions from the overall structure reduced the overall pulling resistance by 50% and compressive resistance by 60%, possibly due to the intermolecular and intramolecular bridges that Ca/Mg create within the cement, strengthening the structure (Sun et al., 2007).
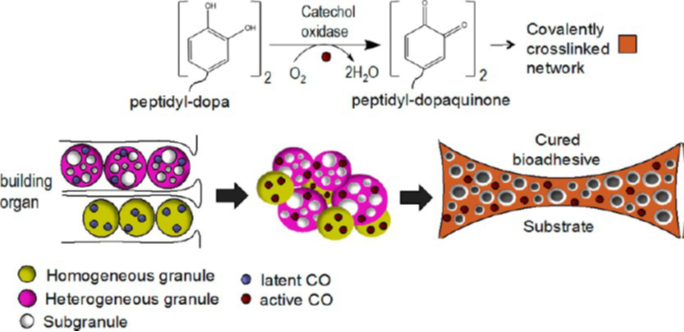
Lastly, further hardening of the glue occurs in what is known as a curing effect, where covalent cross-linking is performed to increase the overall stability of the structure since crosslinked polymers are stronger than simple intermolecular forces. This type of bonding is possible due to the content found in both types of granules, catechol oxidase, which hydroxylases DOPA to dopaquinones and enables it to covalently crosslink between each other. Furthermore, this enzyme stays active within the solidified walls of the glue long after the solidification process is done, proving that it is fact an essential element of the bioadhesive structure due to its role in crosslinking (Stewart et al., 2017).
This unique bioadhesive enables sandcastle worms to collect material around them and build the vital tubular shells that serve to protect them. Through this article, it has been demonstrated how Phragmatopoma californica form this glue and the uniqueness of its structure. This marine glue should be further investigated for other possible applications in the medical world, such as for the control of blood flow in hemorrhages, as the stickiness of the glue in liquid can be taken advantage of for its use in the liquid environment of the body (Stewart et al., 2017).
Conclusion
This essay has presented various ways that animals use adhesives to build homes. Interestingly, these structures may be multifunctional, serving not only as shelter but also as food collection mechanisms. Whether it is a spider spinning a web or larvae covering themselves in miscellaneous materials, they all share the same goal: survival. Each example discussed is a unique solution to the challenges faced by each animal, and these strategies have been perfected over the course of evolution. Due to the chemical nature of adhesion, all animal-built structures making use of these substances are bound to share similarities. However, nature's technologies are so complex and specific to each animal and environment. This leads to a stunning diversity of strategies, materials and structures that can be observed in natural habitats, each tailored to an animal's needs. Nature never disappoints: this engineering prowess is fascinating and incomparably sophisticated. Many fields of human engineering can benefit from observing the work of a true engineering master: Mother Nature.
References
Ardnt, I. (2014). Animal Architecture. Abrams, Harry N.
Bhagat, V., O'Brien, E., Zhou, J., & Becker, M. L. (2016). Caddisfly Inspired Phosphorylated Poly(ester urea)-Based Degradable Bone Adhesives. Biomacromolecules, 17(9), 3016-3024. https://doi.org/10.1021/acs.biomac.6b00875
Bott, R. A., Baumgartner, W., Braunig, P., Menzel, F., & Joel, A. C. (2017). Adhesion enhancement of cribellate capture threads by epicuticular waxes of the insect prey sheds new light on spider web evolution. Proceedings. Biological sciences, 284(1855). https://doi.org/10.1098/rspb.2017.0363
Conrad, J. (2008, September 29). Jim Conrad's Naturalist Newsletter. Backyard Nature. Retrieved October 8, 2021 from https://www.backyardnature.net/n/08/080929.htm
FPWing. Drone of bees. Adobe Stock. Retrieved October 8, 2021 from https://stock.adobe.com/ca/images/drone-of-bees/142847079?as_campaign=ftmigration2&as_channel=dpcft&as_campclass=brand&as_source=ft_web&as_camptype=acquisition&as_audience=users&as_content=closure_asset-detail-page&asset_id=111968408
Gaar, J., Naffa, R., & Brimble, M. (2020). Enzymatic and non-enzymatic crosslinks found in collagen and elastin and their chemical synthesis. Organic Chemistry Frontiers, 7(18), 2789-2814. https://doi.org/10.1039/d0qo00624f
Hayashi, C. Y., Shipley, N. H., & Lewis, R. V. (1999). Hypotheses that correlate the sequence, structure, and mechanical properties of spider silk proteins. Int J Biol Macromol, 24(2-3), 271-275. https://doi.org/10.1016/s0141-8130(98)00089-0
Huang, S., Zhang, C. P., Wang, K., Li, G. Q., & Hu, F. L. (2014). Recent advances in the chemical composition of propolis. Molecules, 19(12), 19610-19632. https://doi.org/10.3390/molecules191219610
Isidorov, V. A., Bakier, S., Piroznikow, E., Zambrzycka, M., & Swiecicka, I. (2016). Selective Behaviour of Honeybees in Acquiring European Propolis Plant Precursors. J Chem Ecol, 42(6), 475-485. https://doi.org/10.1007/s10886-016-0708-9
Kim, J. I., Pant, H. R., Sim, H. J., Lee, K. M., & Kim, C. S. (2014). Electrospun propolis/polyurethane composite nanofibers for biomedical applications. Mater Sci Eng C Mater Biol Appl, 44, 52-57. https://doi.org/10.1016/j.msec.2014.07.062
Romer, L., & Scheibel, T. (2008). The elaborate structure of spider silk: structure and function of a natural high performance fiber. Prion, 2(4), 154-161. https://doi.org/10.4161/pri.2.4.7490
Saccardi, L., Schiebl, J., Weber, K., Schwarz, O., Gorb, S., & Kovalev, A. (2021). Adhesive Behavior of Propolis on Different Substrates [Original Research]. Frontiers in Mechanical Engineering, 7. https://doi.org/10.3389/fmech.2021.660517
Sahni, V., Blackledge, T. A., & Dhinojwala, A. (2010). Viscoelastic solids explain spider web stickiness. Nat Commun, 1, 19. https://doi.org/10.1038/ncomms1019
Sahni, V., Blackledge, T. A., & Dhinojwala, A. (2011). A Review on Spider Silk Adhesion. The Journal of Adhesion, 87(6), 595-614. https://doi.org/10.1080/00218464.2011.583588
Schmolz, E., Brüders, N., Daum, R., & Lamprecht, I. (2000). Thermoanalytical investigations on paper covers of social wasps. Thermochimica Acta, 361(1), 121-129. https://doi.org/https://doi.org/10.1016/S0040-6031(00)00553-0
Stewart, R. J., Wang, C. S., Song, I. T., & Jones, J. P. (2017). The role of coacervation and phase transitions in the sandcastle worm adhesive system. Adv Colloid Interface Sci, 239, 88-96. https://doi.org/10.1016/j.cis.2016.06.008
Sun, C., Fantner, G. E., Adams, J., Hansma, P. K., & Waite, J. H. (2007). The role of calcium and magnesium in the concrete tubes of the sandcastle worm. J Exp Biol, 210(Pt 8), 1481-1488. https://doi.org/10.1242/jeb.02759
Sun, C. J., Srivastava, A., Reifert, J. R., & Waite, J. H. (2009). Halogenated DOPA in a Marine Adhesive Protein. J Adhes, 85(2-3), 126. https://doi.org/10.1080/00218460902782188
Tsukada, M., Khan, M. M., Inoue, E., Kimura, G., Hun, J. Y., Mishima, M., & Hirabayashi, K. (2010). Physical properties and structure of aquatic silk fiber from Stenopsyche marmorata. Int J Biol Macromol, 46(1), 54-58. https://doi.org/10.1016/j.ijbiomac.2009.10.003
Wang, C. S., Pan, H., Weerasekare, G. M., & Stewart, R. J. (2015). Peroxidase-catalysed interfacial adhesion of aquatic caddisworm silk. J R Soc Interface, 12(112). https://doi.org/10.1098/rsif.2015.0710
Wang, C. S., & Stewart, R. J. (2012). Localization of the bioadhesive precursors of the sandcastle worm, Phragmatopoma californica (Fewkes). J Exp Biol, 215(Pt 2), 351-361. https://doi.org/10.1242/jeb.065011
Wang, C. S., & Stewart, R. J. (2013). Multipart copolyelectrolyte adhesive of the sandcastle worm, Phragmatopoma californica (Fewkes): catechol oxidase catalyzed curing through peptidyl-DOPA. Biomacromolecules, 14(5), 1607-1617. https://doi.org/10.1021/bm400251k
Zschokke, S., & Logunov, D. V. (2010). P.A. Sebastian & K.V. Peter (Hrsg.) (2009): Spiders of India. Arachnologische Mitteilungen, 39, 45-49. https://doi.org/10.5431/aramit3907