An Analysis of the Chemistry Behind Coccolithophores
Megan Farrow, Zackary Murphy, Minh Tri Pham, Jia Yi Yu
Abstract
The world's irreplaceable calcifying organism, coccolithophore, utilizes many different chemical processes in its quest for calcification and survival, among other things. The processes and structures detailed in this paper demonstrate how coccolithophores cleverly use the readily available molecules around them to contribute to their various processes and structural demands. Some topics discussed include the role of Ca2+ ions and coccolithophore-associated polysaccharides in coccolithogenesis; the path of CO2 amidst the interacting calcification and photosynthesis processes; the damage done to proton channels in the organisms due to increasing ocean acidity levels, affecting coccolith synthesis; diploid to haploid state conversions of coccolithophores induced by viral interactions; and how the tubulin code and microtubule-associated proteins provide the microtubules of the vestigial haptonema with the flexibility and strength required to repeatedly undergo bending stresses beyond their breaking point.
Introduction
Coccolithophore, a single-celled marine photosynthetic protist belonging to the haptophyte division of Chromalveolata eukaryotes, is thought to have been around since 330 million years ago (Brownlee et al., 2015)(Ben-Joseph et al., 2023). Though tiny, ranging in size from 2.0 – 75.0 µm in cell diameter (Tyrrell & Young, 2009), these unicellular organisms have had significant impacts on the ocean environment and have even come to shape some of Earth's most astonishing landmarks such as the cliffs of Dover (Balch et al., 2016). Through a biomineralization process, coccolithophores form intricate calcite platelets called coccoliths, which, when produced and decomposed in mass, have major effects on the carbon and calcium biogeochemical cycles (Walker & Langer, 2021). In fact, producing 1.5 million tons of calcite every year, coccolithophores are the leading calcite producers of the ocean environment (Weier, 1999). Not only are coccolithophores important with respect to the geochemical cycles of the ocean, but, being primary producers, they are at the bottom of many food chains. With their ability to photosynthesize, and high lipid content, these microscopic unicellular organisms provide a high-energy food source for primary consumers such as zooplankton (Johnson et al., 2022).
Having been on Earth for so long, coccolithophores have had to endure many different environments, adapting to each one. With changes in the chemical composition of their environment, whether it be carbon flux, calcium flux, or the limitation of other major nutrient sources, coccolithophores modify their internal reactions to maximize cell efficiency. In recent years, with more and more anthropogenic sources and pollution every year, the ocean environment is changing very rapidly, posing a threat to the highly important coccolithophore. In this paper, these reactions that take place within and around coccolithophores will be discussed, with an emphasis on the chemistry that drives them.
Coccolith synthesis
Coccolithophores are unicellular phytoplanktonic organisms that are among the most prolific producers of calcium carbonate on the planet; they are responsible for the production of the colossal number of 1026 coccoliths per year (Lee et al., 2016). As seen in “The Physical Properties and Interactions of Coccolithophores”, coccoliths are composed of calcium carbonate (CaCO3) nanocrystals and they interlock in a polysaccharide matrix to form the coccosphere that encapsulates the cell body of the coccolithophore. While the multiple functions of the coccoliths were explained in the previous paper, the present paper will dive into their synthesis, notably the mechanisms behind coccolithogenesis.

Figure 1. (a) A coccolith of Emiliania Huxleyi, one of the most common species of coccolithophores. (b) A segment of a coccolith, consisting of the lower element (l.e.), the medially directed element (m.e.), the central element (c.e.), and the upper element (u.p.) (Westbroek et al., 1984).
The overall mechanism of coccolithogenesis
The synthesis of a coccolith takes place inside the cell, more precisely in a vesicle derived from the Golgi apparatus, the coccolith vesicle, as seen in Fig. 2 (Lee et al., 2016). This specialized intracellular vacuolar complex is positioned next to the nucleus (N) and it surrounds the coccolith as it grows (Westbroek et al., 1984). There is also a system of interconnected tubes called the reticular body that is continuous with the coccolith vesicle (Fig. 2) (Westbroek et al., 1984).

Figure 2. An idealized section through an E. huxleyi cell. The coccolith vacuole (c.v.) can be seen at the top-right of the cell, containing a developing coccolith (X) and the reticular body (r.b.). The nucleus (N) is right below the vacuole, and the Golgi apparatus (G) is positioned on the left side. Extracellular coccoliths are pictured in black, with their different elements denoted (Westbroek et al., 1984).
The nucleation of the CaCO3 crystals initiates on the rim of an organic baseplate (b.p.), forming the protococcolith ring (Marzec et al., 2019). These incipient crystals are in the form of rhombohedral crystallites and are situated where the central elements of the coccolith will ultimately be (Fig. 3, a) (Westbroek et al., 1984). The crystals grow out in three directions – radially, disto-radially, and medially – until they mechanically interlock (Fig. 3, b-c) (Westbroek et al., 1984). This mineralization process is able to occur with the help of molecules called coccolith-associated polysaccharides (CAPs) which are involved in the transport of Ca2+ ions to the baseplate (Marzec et al., 2019). To promote crystal nucleation, the baseplate contains positively charged primary amines and proteins while the CAPs are acidic (negatively charged); thus, electrostatic interactions occur, allowing the CAPs to recognize the baseplate and carry calcium cations to it (Marzec et al., 2019). The CAPs also regulate the rate of calcification as they interact with the calcite crystals, and their significance will be discussed later in this paper (Pomar, 2020). When the calcification process of the coccolith is finalized, the product migrates to the cell membrane and is extruded out of the cell to be incorporated into the coccosphere (Pomar, 2020).

Figure 3. Electron micrographs of a coccolith vacuole undergoing coccolithogenesis. In (a), the rhombohedral crystallites are shown in black. In (b), the incipient coccolith continues growing from the central element. In (c), the lower and upper elements are formed, while the baseplate extends towards the lower elements (Westbroek et al., 1984).
Factors affecting coccolithogenesis and crystal nucleation rates
The synthesis of coccoliths as well as their rate of nucleation are determined by several factors, many of which have a direct impact on the survival of coccolithophores and their ability to adapt to their changing environment.
Conditions necessary for calcification to occur
Visualization of E. huxleyi cells through electron microscopy shows that in the case of depletion of Ca2+ in the cell, the complex formed by the reticular body and the coccolith vesicle will degenerate; the reticular body first disintegrates, followed by the coccolith vesicle being detached from the nucleus and disappearing (Fig. 4, a-b) (Westbroek et al., 1984). The same phenomenon occurs when the cell has been subject to prolonged darkness (Westbroek et al., 1984).

Figure 4. Electron micrographs (a) and (b) show cells of E. huxleyi after decalcification and prolonged exposure to darkness. The reticular body has vanished in both cases. In (a), the coccolith vacuole is still apposed to the nucleus (N), while in (b), the coccolith vacuole is detached from the nuclear envelope. In (c), the morphology of a naked E. huxleyi cell is shown; the baseplate (b.p.) is crumbly and the coccolith vacuole (not labeled) is detached from the nucleus (Westbroek et al., 1984).
Likewise, E. huxleyi cells incapable of forming coccoliths (termed ‘naked') have similar morphology to normal coccolith-bearing cells; though, it has been seen that their coccolith vesicles are not well attached to their nuclei (Westbroek et al., 1984). In addition, their baseplate has an uneven appearance, as seen in Fig. 4, c. Thus, it is clear that the absence of Ca2+ and light inhibits the production of the calcite structures. The relationship between coccolith calcification, Ca2+ availability, and photosynthesis will be further discussed later in this paper.
Coccolith-associated polysaccharides (CAPs)
During the synthesis of the coccolith, previously mentioned molecules called coccolith-associated polysaccharides (CAPs) are found in multiple regions of the coccolith vacuole. In fact, as represented by darker areas in Fig. 3 e-f, CAPs are present in the outlines of the membranes of the coccolith vacuole (c.v.) and the reticular body (r.b.), in fine threads of amorphous material inside the lumen, in the base plate, and in a thin film surrounding the calcite crystals (Westbroek et al., 1984). These macromolecules are composed of a mannose (sugar) backbone with highly branched side chains that contain at least thirteen different monosaccharides, sulphate esters, and uronic acids (Lee et al., 2016; Westbroek et al., 1984). The uronic acids contain negatively charged carboxyl groups that give CAPs an acidic character and that allow them to bind to positively charged calcium cations in the coccolith vesicle (Lee et al., 2016). It is these chemical properties that give CAPs the ability to control the mechanism behind coccolith synthesis (Lee et al., 2016).

Figure 5. A D-Glucuronic acid molecule in pyranose form. The carboxyl group is highlighted in red (Hardinger).
To better understand the role of CAPs and their uronic acids, Lee et al. studied several coccolithophore species of two different orders: Coccolithales and Isochrysidales; although both possess coccosphere shells, the species studied exhibited different degrees of coccolith calcification, and they were ultrastructurally and physiologically diverse (Fig. 6) and present in various habitats (Lee et al., 2016). Furthermore, CAPs of both modern coccolith and coccolith fossil samples were isolated, since it was demonstrated that CAPs in sedimentary coccoliths are preserved and have a chemical composition similar to that of present-day cultures (Sand et al., 2014). Experiments conducted on these various CAPs showed that their uronic acid content (UAC) was different across the Coccolithales and the Isochrysidales and even across different strains of the E. huxleyi species, meaning that the UAC of coccolithophores is a distinct biochemical characteristic (Lee et al., 2016). Indeed, UAC could potentially be used as a biomarker to distinguish different taxonomies when morphological and DNA preservation is ambiguous (Rickaby et al., 2016).


Figure 6. Scanning electron micrographs of the coccoliths of the Isochrysidale and Coccolithale live strains that were studied (Lee et al., 2016).
Uronic acid content (UAC)
So how exactly do CAPs and their UAC regulate coccolithogenesis? The answer is that the rate of coccolith synthesis depends on the interplay between the UAC and the internal saturation state of the coccolithophore (Lee et al., 2016). The internal saturation state depends on several parameters: the utilization rate of calcium over carbon known as the PIC/POC ratio (where PIC stands for particulate inorganic carbon and POC for particulate organic carbon), the CO2 diffusion rate given by SA/V (where SA is the surface area and V is the volume of the coccolithophore), and the size of the organism (Lee et al., 2016). Bigger organisms like coccolithales have a high PIC/POC and a slow CO2 diffusion, leading to a lower saturation state, whereas in smaller organisms like isochrysidales, the PIC/POC ratio is lower and the SA/V ratio is higher, so their internal saturation state is higher; they are also capable of increasing their internal saturation state by using a carbon concentrating mechanism (Lee et al., 2016). Data has shown that at low saturation states, organisms with lower UAC have faster rates of nucleation, whereas in species with higher saturation states, a higher UAC means an increase in calcification (Lee et al., 2016). Coccolithophores exploit this characteristic by altering their UAC depending on their internal saturation state to achieve optimal calcification.
For example, by studying Coccolithale fossils, Lee et al. have shown that these coccolithophores had a high UAC when CO2 levels (pCO2) were high (Lee et al., 2016). However, when carbon availability decreased due to the decline in pCO2, the internal saturation state of the Coccolithales also decreased; so to sustain their coccolith synthesis and maintain their large size, the organisms had to reduce the UAC of their CAPs to optimize calcification in a low saturation state (Lee et al., 2016). On the other hand, in response to the decreasing pCO2, Isochrysidales have instead reduced their size, which led to the maintaining of a highly saturated environment; so, to promote calcification, the organisms had to have a high UAC (Lee et al., 2016). Therefore, by controlling their UAC such that their calcification levels were maintained, coccolithophores have adopted an ingenious strategy that maximizes their chances of survival.
Additionally, the study of fossil coccolithophores could be a promising tool in the field of biogeochemistry to estimate the change in environmental pCO2, since their CAPs and UAC are directly correlated to the changes in CO2 concentrations.
Calcification and photosynthesis
Coccolithophores are largely affected by and largely affect the ocean's pCO2 levels through their two important processes; calcification and photosynthesis. Through calcification, coccolithophores produce inorganic carbon in the form of CO2, though, consume inorganic carbon through photosynthesis, converting it to organic carbon. These two equations are found below.
(calcification from (Marsh, 2003))
(photosynthesis from (Marsh, 2003))
Photosynthesis can be said to occur in two different phases, the light-dependent energy-producing reactions, and the light-independent Calvin cycle. During the light-dependent reactions, photons are first absorbed by the complex of pigments known as photosystem II. The energy is passed from pigment to pigment through the excitation of electrons until it reaches the reaction center where it is transferred to P680, a highly electronegative molecule. P680 loses this highly excited electron to a carrier protein, and splits water, taking an electron, to replace it. The electron travels through an electron transport chain, losing energy and building a proton gradient at the same time. These protons later go on to drive ATP synthase in producing ATP (adenosine triphosphate). The now relatively low-energy electron is used by another electronegative molecule, P700, as the molecule loses an electron at photosystem I. The new energized electron is transported and used to protonate NADP+ into NADPH, an energy-carrying molecule. ATP and NADPH are then used to overcome surface activation barriers in the Calvin cycle, where CO2 is taken in and combined to form sugars (C6H12O6) through a series of reactions. These reactions are summarized in Fig. 7.
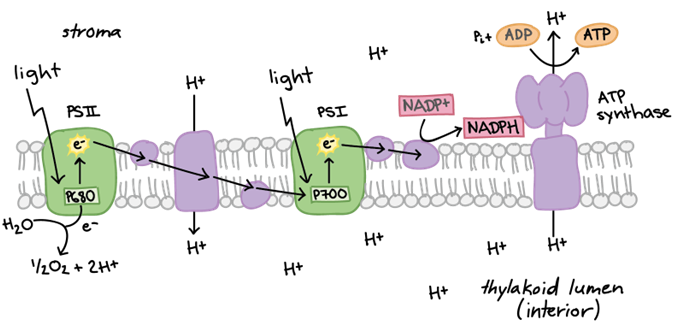
Figure 7. (A) Summary of electron transport chain in light dependent reactions (B) Summary of light dependent and independent (Calvin cycle) reactions that take place during photosynthesis (Raad & Abdulmajeed, 2018).
As is clear, photosynthesis is dependent on the cell's access to water, carbon dioxide, and UV radiation. However, what may not seem as obvious, the calcification process has been shown to also be very light-dependent, with calcification in dark environments taking place at only 10-15% the rate of calcification in light-saturated environments. Coccolith formation, requiring the synthesis of organic structures and transport of ions, depends on energy; thus, this process is most likely mediated by chloroplast pigments and the light-dependent reactions of photosynthesis. Looking at Fig.8, we see the direct correlation between the calcification rate and the relative electron transport rate (powered by photon absorption) When there is a lack of solar radiation, energy for coccolith formation is supplied by cellular respiration which is highly limited, potentially explaining the reduced rates seen in the dark (Paasche, 2001). This reduced calcification rate is most likely a design strategy of coccolithophores, saving energy for more important tasks. Described as an electron sink, calcification's use of energy can also be extraordinarily beneficial using up excess energy that can otherwise be harmful. (Zhang & Gao, 2021). This is a photoprotective design solution by coccolithophores to reduce overexcitation of the photosystems, protecting the cell by dissipating excessive energy. In fact, these cells are continuously acclimating to macro-nutrient limitations, elevated CO2 concentrations, and flux in radiation in order to achieve maximal electron transport, photosynthetic, and calcification rates (Zhang & Gao, 2021).

Figure 8. Linear relation between calcification and relative electron transport rate grown with different treatments; (Left: High Nitrogen, High Phosphate), (Middle: Low Nitrogen, High Phosphate), and (Right: High Nitrogen, Low Phosphate) (Zhang & Gao, 2021).
Photosynthesis and calcification coupling models
The two processes of calcification and photosynthesis are quite intertwined, and both heavily depend on dissolved inorganic carbon (DIC). DIC is said to be composed of carbon dioxide (CO2), bicarbonate ions (HCO3–, and carbonate ions (CO32-), whose proportions vary with pH. At the normal pH of seawater (8.1-8.2), less than 1% of DIC is present as CO2, while more than 90% is present as bicarbonate ions and the rest as carbonate ions. The affinity of Rubisco (Ribulose-1,5-bisphosphate carboxylase/oxygenase, responsible for first fixating CO2) for CO2 is remarkably poor while diffusion of CO2 into algal cells is also extremely slow (Paasche, 2001). With this being the case, how is photosynthesis able to occur with such low levels of carbon dioxide? This conundrum is solved by further connecting calcification and photosynthesis, where calcification is used to increase intracellular CO2 concentration, better-supplying CO2 to Rubisco (Paasche, 2001). This supplementary CO2 is provided within the cell by the following reaction.
(Paasche, 2001)
There are currently many different models for photosynthesis and calcification interactions, which have evolved over the past few decades. Originally, it was assumed that bicarbonate ions could be used for both photosynthesis and calcification, but that the two processes were not closely linked (Fig. 10). This was reasoned as the light saturation kinetics of the two reactions differed, photosynthetic rates did not decrease when cells were in calcium-free mediums, and light-dependent calcification was less sensitive to inhibition of photosystem II. The evolution of this model (Fig. 11) suggested that CO2 produced in calcification was used for photosynthesis.
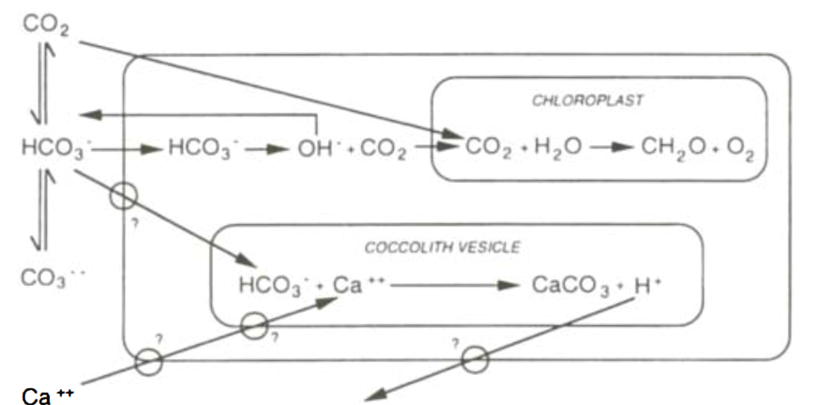
Figure 10. First prediction of calcification and photosynthesis model, with the two reactions uncoupled. Large box represents the cell, with the equilibria of external dissolved inorganic carbon system shown to the left. Potentially energy-requiring transmembrane transport sites are ringed (Paasche, 2001).

Figure 11. Later model of early bicarbonate system, showing interaction between calcification and photosynthesis, cell represented by large external box (Paasche, 2001).
Fig. 12 and 13 represent the currently accepted models for the interactions between calcification and photosynthesis. In these models, the inter-conversion of bicarbonate and carbon dioxide, mediated by CA is represented, along with the balancing of protons by hydroxyl ions, maintaining a pH of around 7.
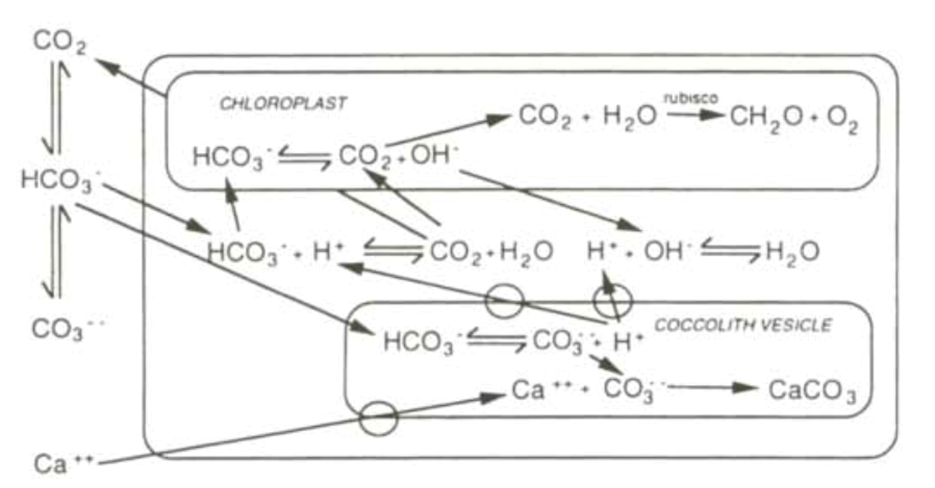
Figure 12. Currently accepted bicarbonate model, showing complex interactions between the two reactions. Cell represented by external box, and transmembrane transport cites represented by rings on membrane surfaces (Paasche, 2001).

Figure 13. Currently accepted bicarbonate model, showing the advantage of carbonic anhydrase converting HCO3– to CO2. Membranes represented by enclosed rounded rectangles, cell membrane being the most outer (Paasche, 2001).
In comparison to other CO2-concentrating mechanisms in algae, calcification could indeed provide an energy-effective method for supplying CO2 to photosynthesis. During calcification, the main energy-consuming steps are the export of protons from the coccolith vesicle and the transport of calcium ions. This ion exchange at the coccolith vesicle membrane would require a minimum of 0.24 mol ATP, per mol of calcite formed. This is equivalent to 8% of ATP required in the reduction of 1 mol of CO2 in the Calvin cycle, or 4% of ATP gained by the oxidation of 1 mol of carbon from the redox level of sugar (Paasche, 2001).
Management of reactants
In fact, the CO2 from the intracellular conversion of bicarbonate is considered the major carbon source in photosynthesis. In addition to this CO2 fostering mechanism, coccolithophores may also present carbonic anhydrase (CA), an external enzyme able to mediate the conversions between the different DIC components (CO2, HCO3–, H+, H2CO3) (Books et al.). Thus, they could increase the concentrations of carbonic acid and carbon dioxide in their immediate surroundings, improving the flow of these molecules into the cell, and increasing their intracellular concentrations. Paasche presents that CA becomes more active in the presence of lower DIC concentrations, and elevated pH (2001), increasing coccolithophore affinity for DIC, and acting as a method to ensure reactants are present for photosynthesis and calcification. Yet another great design solution.
In order to produce calcite for coccolith generation and carbon dioxide for photosynthesis, coccolithophores also require the presence of calcium ions in high concentrations. Luckily, the concentration of calcium ions (Ca2+) in typical ocean environments is in high excess (average at 10mM) compared to DIC (average at 2mM). However, the transport of calcium ions through the cytosol may present as a bottleneck in terms of coccolith formation. While Ca2+ concentration in the coccolith vesicle should be maintained to be around that of seawater in order for calcite to precipitate, concentration in the cytosol is known to be five orders of magnitude lower. To solve this issue, coccolithophores use endocytosis, a technique prevalent amongst almost all eukaryotic (Paasche, 2001). Through endocytosis, molecules from the external environment are surrounded by a portion of the cell membrane which then buds off internally, creating a pocket of membrane filled with intercellular fluid, known as a vesicle. This transport vesicle can then deliver calcium ions to the coccolith vesicle, increasing the surrounding calcium concentration and allowing for coccolith formation and precipitation. See Fig. 9 for a diagram of this process.

Figure 9. Process of endocytosis and the formation of a vesicle where squares and stars represent ions in ocean media (Villareal, 2007).
Well-preserved coccoliths can be found in sedimentary records of 220 Ma and estimates say the first calcifying haptophytes originated around 300 Ma (Ben-Joseph et al., 2023). Thus, coccolithophores have evolved under many majorly different ocean carbonate chemistry conditions than what is present today (Brownlee et al., 2015). Using their adaptable interconnected photosynthesis and calcification systems has allowed them to be adaptable to the changing environment.
Effects of ocean acidification on calcification and coccolith morphology
Ocean acidification is a phenomenon that happens as a result of increasing carbon dioxide (CO2) concentration in the atmosphere due to anthropogenic emissions. The dissolution of CO2 in seawater in recent years has made a big change to ocean carbonate chemistry, consequently decreasing the pH of seawater. The process by which CO2 dissolution modifies the ocean pH is described by the following chemical reaction:
According to the reaction above, every mole of carbon dioxide dissolved in seawater can contribute one mole of carbonate ions and two moles of hydrogen ions into the ocean. This contribution of hydrogen ions will increase acidity and decrease ocean pH. The mean global surface ocean pH is currently at around 8.2 but it is predicted that this pH will fall as low as 7.7 in 2100 (Kottmeier et al., 2022).
Ocean acidification is known to have a negative effect on the growth of many marine organisms. Although coccolithophores have adapted to numerous changing environments, as with other organisms producing calcium carbonate shells and skeletons, they experience the strongest negative impacts from ocean acidification. These impacts include reduced calcification, reduced rates of repair, and weakened calcified structures (Gattuso et al., 2015). In this section, we will explore the effect of ocean acidification on our favorite calcified organism, the coccolithophore (Fig. 14).
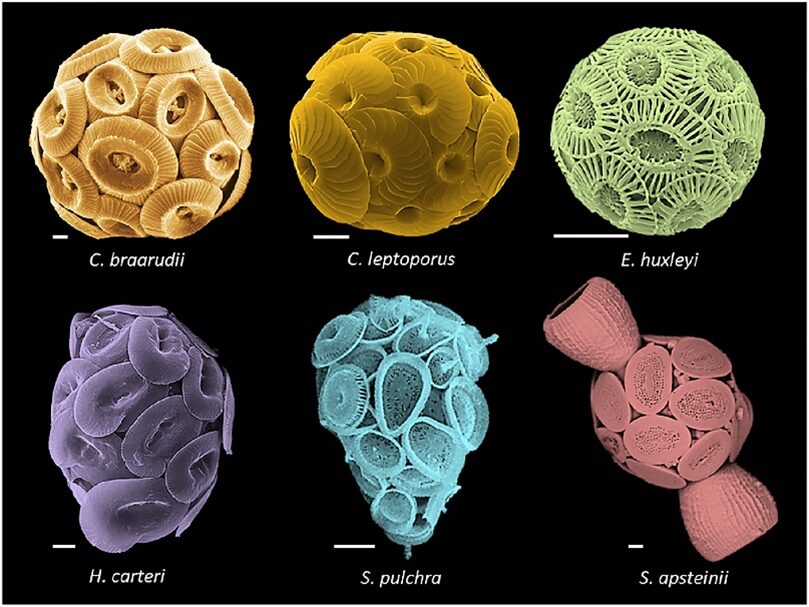
Figure 14. Coccolithophore morphologies. Top (left to right): Coccolithus braarudii, Calcidiscus leptoporus, Emiliania huxleyi. Bottom: Helicosphaera carteri, Syracosphaera pulchra, Scyphosphaera apstenii. Scale bars, 2 μm (Brownlee et al., 2021).
Unlike other calcifying organisms, such as mollusks, corals, or even foraminifera, calcification in coccolithophore happens intracellularly, within Golgi-derived coccolith vesicles. Therefore, this involves the transport of substrate from the surroundings into the site of calcification. The substrates required for calcification, bicarbonate (HCO3—) and calcium ions (Ca2+), get transported into the cell in a controlled manner as well as the removal of H+ from the coccolith vesicle and cell.
The pathway for Ca2+ delivery to the intracellular site of calcification has yet to be definitively established. Though, the passive Ca2+ entry into the cell via cation channels, and sometimes coupled with active transport, for example via a Ca2+ /H+ antiporter, is a possible route of Ca2+ entry into the coccolith vesicle, in addition to the process of endocytosis previously mentioned (Brownlee et al., 2021). On the other hand, H+ efflux happens via a voltage-gated channel (Fig. 15). The removal of H+ from the intracellular media is necessary for maintaining cytosolic pH at around 7.0. With a resting membrane potential of -46mV, there is a small net outward electrochemical gradient for H+ across the coccolithophore plasma membrane at a seawater pH of 8.15. This outward movement of H+ is facilitated by the proton motive force (pmf), the proton channel is therefore pH dependent. A decrease in cytosolic pH increases the outwardly directed pmf, resulting in channel activation and net H+ efflux. The pH dependence of voltage gating means that H+ efflux through H+ channels is almost always outward. However, this characteristic also means that the operation of H+ channels is sensitive to changes in external pH. The relationship between resting potential, cytosolic pH (pHcyt), and external pH (pHo) is described in detail in the figure below (Fig. 16).

Figure 15. The electrical control of cellular pH through the action of voltage-gated H+ channels (Brownlee et al., 2021).

Figure 16. The impact of changes in the transmembrane H+ gradient on the operation of voltage-gated H+ channels. A) Low pH internal case, B) Low pH external case (SI, Kottmeier et al., 2022).
In Figure 16A, we can see that the decrease in cytosolic pH from 7.1 to 6.8 causes a shift in the activation potential of the H+ current to more negative values (red arrows). This shift is below the resting potential of the cell (black arrow) and, therefore triggers the opening of the H+ voltage-gated channel. The decrease in cytosolic pH also increases the outward proton motive force (pmf). On the other hand, the decrease in extracellular pH appears to cause the opposite effect. In Figure 16B, as external pH decreases from 8.2 to 7.6, the activation potential is shifted to a more positive value. This inhibits the opening of the H+ channel, combining with an inward pmf, to stop the efflux of H+ from the cell. Under acidified conditions, channel-mediated H+ efflux would only occur following more substantial depolarization of the membrane potential and/or further reductions in cytosolic pH. By monitoring H+ currents, using the patch-clamp technique, recordings in C. braarudii cells previously acclimated to pHo 7.55, 8.15, or 8.75, it is shown that the mean amplitude of the outward H+ current was greatly reduced in cells that had been acclimated to pH 7.55 (Fig. 17); 52.9% of cells acclimated to pH 7.55 exhibited either greatly reduced or undetectable outward current (Kottmeier et al., 2022).

Figure 17. Cells acclimated to pH 7.55 exhibit a greatly reduced outward current, y-axis is the current density and x-axis is the voltage of cell (Kottmeier et al., 2022).
Furthermore, the reduction of external pH also causes damage to the H+ channel and leads to a disruption in coccolithophore pH homeostasis. The coccolithophore cells grown at pH 8.75 and pH 8.15 retain the ability to adjust intracellular pH rapidly when exposed to higher or lower pH, while cells grown at pH 7.55 lose this homeostasis ability. A significant number of cells acclimated at pH 7.55 exhibit a defect in H+ efflux and hence cause an unresponsive behavior in coccolithophore cells to pH change. The percentage of cells with defective pH efflux is shown below (Fig. 18) (Kottmeier et al., 2022).

Figure 18. The proportion of cells that do not show an outward current between different acclimated culture (Kottmeier et al., 2022).
The defect in the H+ channel is the cause of the coccolithophore's malformation at low pH. It is observed that coccolithophore cells grown at low pH have an abnormal morphology with malformation of coccolith. Under low pH conditions, the coccolith appears as a ring of calcite rather than a fully formed shield (Fig. 19A). This malformation of calcite plates results in the inability to maintain the structural integrity of the coccosphere. Consequently, under low pH, the coccospheres are prone to collapse (Fig.19B).


Figure 19. A) Representative scanning electron micrographs of cells acclimated to (left to right) pH 7.55, 7.85, 8.15, and 8.75. B) Shield elements are not properly formed, the coccoliths are unable to interlock in the normal manner, resulting in the collapse of the coccospheres (Kottmeier et al., 2022).
To confirm the connection between a defective H+ channel and the abnormal morphology of coccolith under low pH conditions, researchers treated coccolithophore cells with proton channel inhibitor, Zn2+, and then observed their morphology. As expected, the morphology of coccolithophore cells with Zn2+ inhibition of the proton channels shows similarity with the morphology of coccolithophore cells grown under low pH (Fig. 20) (Kottmeier et al., 2022).

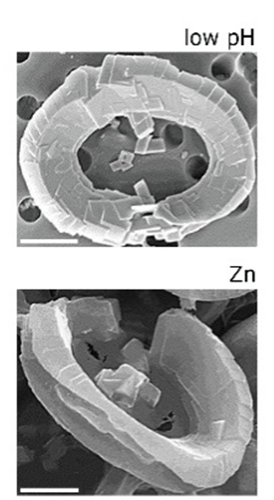
Figure 20. Similarity between the morphology of cells growth at low pH and cells that are treated with Zn2+ (Kottmeier et al., 2022).
To summarize, ocean acidification has a detrimental effect on the calcification of coccolithophores. The decrease in external pH cancels the proton motive force and increases the activation potential threshold, therefore inhibiting the efflux of hydrogen ions. Consequently, this causes damage to the proton channel and removes the ability of the coccolithophore to perform pH homeostasis. The damage to a proton channel caused by low external pH further leads to the malformation of their calcite shell. When calcite platelets are malformed, structural integrity cannot be maintained, resulting in the collapse of the coccosphere. Unfortunately, coccolithophores are yet to develop a design solution for this issue, and because the change in their environment is happening so quickly, they may not evolve in time.
Coccolithophore viral interaction
As with all other species, the possibility of disease, or viral infection poses another large threat. The cosmopolitan coccolithophore Emiliania huxleyi is the most abundant coccolithophore species in the modern ocean, responsible for vast blooms in the ocean. As we have seen in the last paper, an E. huxleyi bloom forms a milky, blue-colored Great Calcite Belt that can be observed in satellite imagery. Interestingly, these blooms are followed by a rapid demise due to viral infection. E. huxleyi is primarily infected by a lytic large double-stranded DNA coccolithovirus Phycodnavirdae, which is also called, quite originally, the E. huxleyi virus (EhV). By controlling the bloom dynamics of coccolithophores, EhV plays an important role in regulating the ocean environment. Viral infections that lead to cell lysis is estimated to turn over at least 20% of the photosynthetic biomass. Thus, viruses have a huge impact on ocean biogeochemical cycles by short-circuiting the flux of carbon and nutrients from phytoplankton and bacteria to higher trophic levels, shunting it to the pool of dissolved and particulate organic matter, and making it more available for recycling by microbial respiration (Sheyn et al., 2016). In this section, we will explore the important interaction between the coccolithophore E. huxleyi and its specific virus EhV.
The EhV-86 is a distinct virus with a 20 nm coat surrounding a nuclear central core. This coat constitutes the virus capsid. In aged virus, the membrane detaches from the capsid resulting in an enlarged virion diameter of ~250 nm (Fig 21).
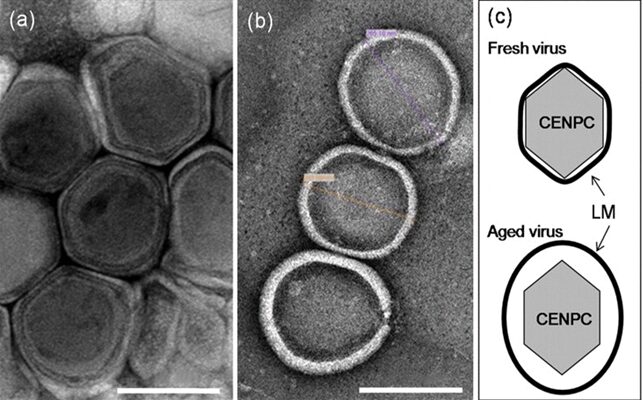
Figure 21. Electron micrographs of fresh (a) and aged (b) EhV-86. (c) Diagrammatic views of fresh and aged virus (Mackinder et al., 2009).
The viral binding of EhV to coccolithophore is a rapid process happening within 2 minutes. The virus can enter the cell via endocytosis, which is then followed by an envelope fusion with the vacuole membrane (Fig 22, step 1a-2) or by direct fusion of the viral envelope with the host membrane (Fig 22, step 1b). No matter the mechanism of internalization, the virus capsid stays intact after entering the cell. Once the virus is inside the cell, the nucleoprotein core rapidly disassembles, releasing its DNA into the host cytoplasm or directly into the nucleus (Fig. 22, step 3). The virus then uses the tools of the host cell to replicate and reproduce (Fig. 22, step 4-5), and release via budding from the cell membrane (Fig.22, step 6). Interestingly, EhV-86 infection and release mechanisms have greater similarities to animal-like viruses than viruses from its own family, phycodnaviruses (Mackinder et al., 2009).

Figure 22. Schematic of the proposed life cycle of EhV-86 (Mackinder et al., 2009).
Unexpectedly, in response to the viral infection, the coccolithophore alternates within variable life cycle phases, the haploid, and the diploid phases. In E. huxleyi, the life cycle comprises of the diploid (2N), nonmotile, coccolith-bearing phase that forms blooms, and the haploid (N) flagellated phase that possesses nonmineralized organic scales overlying the cell membrane (Fig. 23) (Mackinder et al., 2009).

Figure 23. Photograph of diploid E. huxleyi (left) and haploid E. huxleyi (right) (Eliza Strickland, on Discover Magazine).
Frada et al. show that biotic interactions with viruses play a key role in directly triggering life cycle changes in E. huxleyi. Oxidative stress in response to viral infection could be the trigger for both the peak of dead E. huxleyi 2N cells at day 6 after infection and the sexual diploid-to-haploid transition observed in an initially pure 2N culture. Unlike diploid cells that are surrounded by interlocking coccoliths and do not produce organic scales covering the cell membrane, in haploid cells, the cell surface is covered by tightly packed body scales organized in overlapping layers. This haploid cell covering efficiently inhibits viruses from connecting with surface receptors on the cell membrane, disallowing them from entering the cell (Frada et al., 2008).
The ability of haploid cells to escape viral infection allows the coccolithophore to optimize the diploid state toward growth rate and nutrient uptake to form blooms, without the need for developing resistance to EhV. If the newly formed haploid cells were not resistant to the EhV, blooming would then have no selective advantage compared with a strategy of maintaining low background diploid cell concentrations. On the other hand, transformation into a haploid phenotype invisible to the virus provides an escape mechanism that ensures that the genes of an individual (or clone) are passed on to the next generation. Consequently, this phenomenon dictates positive selection for rapid growth and meiosis in the diploid host while imposing little negative selection pressure for high infectivity of the virus (Frada et al., 2008). This unexpected viral escaping mechanism in coccolithophore is a design solution that allows its diploid cells to form blooms along the beautiful Great Calcite Belt that we can observe in the last paper.
To summarize, the E. huxleyi virus (EhV) is very effective at infecting coccolithophore diploid cells, while it develops a technique quite different from viruses of its family to directly target the E. huxleyi coccolithophore cells. Quite surprisingly, E. huxleyi did not grow resistance to this virus in its diploid state but instead focused on maximizing its growth rate to create blooms. The reason for this adaptation in the coccolithophore E. huxleyi is due to its ability to switch from a diploid to a haploid state after the infection, where the haploid state is resistant to virus infection. Thus, this ensures that genes of this coccolithophore species will be passed on to the next generation, to form another bloom in the future.
Microtubular flexibility and support in the vestigial haptonema
Coccolithophores also require the ability to evade obstacles, adhere to objects, and capture prey, to do so, they use a structure unique to haptophytes (which include coccolithophores), the vestigial haptonema (for more details, please refer to “The Physical Properties and Interactions of Coccolithophores”). To avoid obstacles, the haptonema must coil up tightly with lightning-fast speed, or gradually bend to capture its prey. This puts the substructures of the organelle under a lot of strain. The haptonema is composed of plasmalemma, which is extended from the cell, and six to seven microtubules (sometimes less depending on the species) which are enveloped by endoplasmic reticulum, also continuous from the peripheral ER of the cell (Fig. 24) (Eikrem et al., 2017).
The microtubules located in the vestigial haptonema undergo immense stresses while undergoing incredibly high-speed coiling and bending of the organelle (Fig. 25). However, microtubules are very rigid by nature, and in the haptonema, they are often bent beyond their structural bending limits (Fig. 25C). This begs the question: how can these rigid structures bend and coil repeatedly beyond their limits without breaking? The answer lies in the intricacies of the microtubular structures and other components of the haptonema.

Figure 24. Cross-sectional views of the vestigial haptonema: (a) depicts the longitudinal cross-section without microtubules; (b-d) depict the transverse cross-section, showing the microtubules enveloped by the endoplasmic reticulum. Endoplasmic reticulum abbreviated: er. (Eikrem et al., 2017).

Figure 25 The haptonema (denoted h) (A) and the microtubules that it is composed of (B, C). Figure A depicts a haptophyte and its flagellar apparatus (two flagella and a haptonema). Figure B shows the individual microtubules that make up the haptonema, in a relatively extended state. Figure C shows the individual microtubules of a coiled haptonema (Nomura et al., 2019).
Microtubules are assembled from a and b-tubulin heterodimers, which are proteins, each with various isotypes, that come together and form a rigid, hollow, cylindrical lattice (Fig. 26) (Janke, 2014). These proteins are subject to heterogeneity, which dictates the microtubule functions. Heterogeneity is generated by the expression of alternative tubulin isotypes, and by post-translational modifications (PTMs) (Janke, 2014), which are the modifications of amino acid side chains in proteins after biosynthesis (Ramazi, Shahin, and Zahiri, 2021), make up what is known as the tubulin code (Fig. 26) (Janke & Magiera, 2020). The tubulin isotypes come from the expression of different tubulin genes and are identical among a vast number of species. Tubulin PTMs, on the other hand, are incredibly vast and are often catalyzed by enzymes (Janke & Magiera, 2020). Some notable PTMs include acetylation, methylation, phosphorylation, and more. The combination of these isotypes and PTMs, however, is what allows the microtubules to be versatile and adopt so many different roles, such as cell division, motility, shape determination, intracellular transport, and more (Janke & Magiera, 2020).
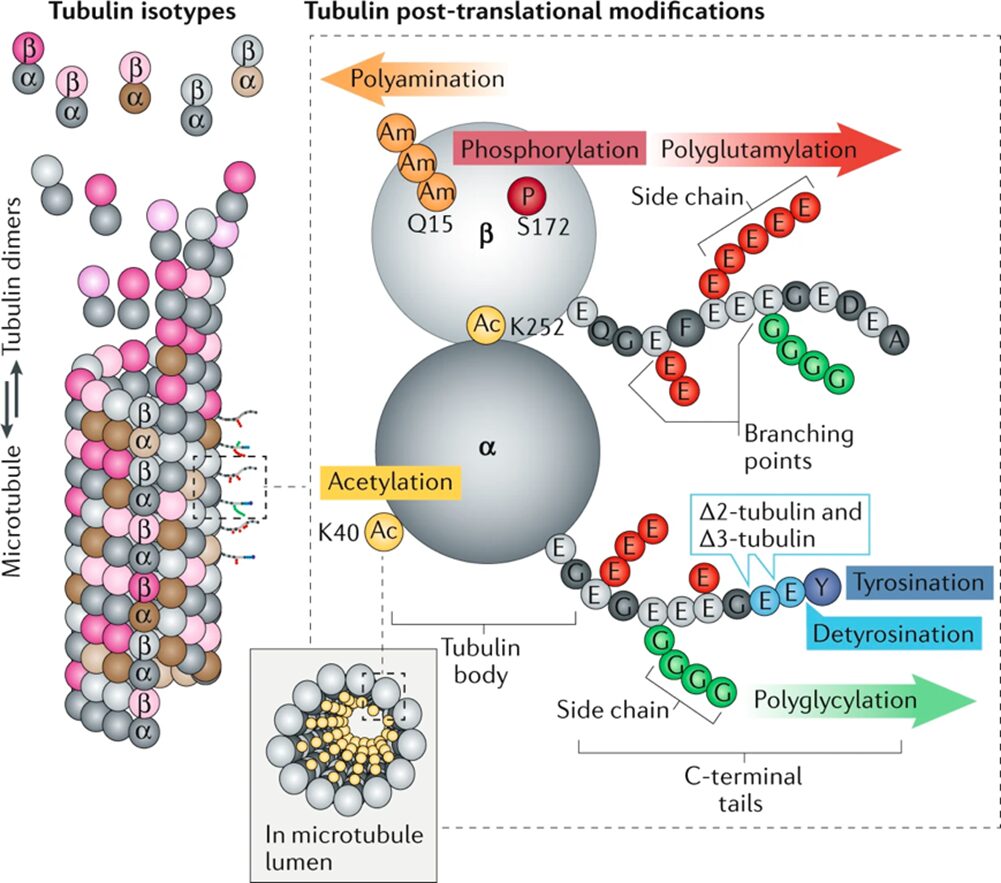
Figure 26 The elements of the tubulin code. In the top-left, some of the a-tubulin (dark grey and brown) and b-tubulin (light grey and pink) isotypes as dimers, encoded by various tubulin genes. On the left, the microtubule structure comprised of these dimers of various isotypes and post-translational modifications (PTMs). On the right, the tubulin heterodimer with the possible post-translational modifications as well as the PTMs' points of insertion (Janke & Magiera, 2020).
Recall the bending stress the microtubules in the haptonema are subject to. It makes no sense that these structures are capable of repeatedly bending past their “breaking point” and maintaining a coiled position for extended periods of time (Fig. 25C). However, as always, nature has come up with a few solutions to this dilemma. One way of reducing the rigidity of the microtubules is to acetylate them. Acetylation is the process of replacing a hydrogen atom with an acetyl group (CH3C=O) within the lumen of the microtubule (Fig. 26) The acetylation of a-tubulin at Lys40 (K40) is a PTM that decreases the flexural rigidity of the microtubule by changing the structure of the aK40 loop of a-tubulin (Fig. 27) (Janke & Magiera, 2020). This disrupts the interaction between Lys60 and His283 in the a-tubulins of the neighbouring protofilaments, which reduces the strength of the interprotofilament contacts, ultimately increasing the microtubule's resistance to mechanical bending-induced breakage and disassembly (Fig. 27) (Janke & Magiera, 2020). What is more, is that this PTM is also said to protect the microtubules from mechanical aging, which is a loss of flexural rigidity due to constant bending (Janke & Magiera, 2020). What permits the microtubule to bend in the first place is the sliding of adjacent protofilaments against one another, which is affected by non-covalent interactions between tubulin molecules (Janke & Magiera, 2020), so by weakening these interactions, there is more leeway for the protofilaments to slide against each other, leading to more flexibility in the microtubule.

Figure 27 Decrease of flexural rigidity of microtubules due to the K40 acetylation of a-tubulin. At the top, the difference between the distances of K60 and H283 in the acetylated vs non-acetylated a-tubulin loops. On the bottom, the depiction of the breakage of a non-acetylated microtubule, induced by mechanical bending vs the resistance to the mechanical bending by the acetylated microtubule (Janke & Magiera, 2020).
In addition to the structural level of the microtubules, their rigidity can be altered using other tools. One of which is the use of microtubule-associated proteins (MAPs). In haptonema, microtubules are reinforced with MAPs to help withstand the bending forces applied to them and prevent them from mechanical bending-induced breakage (Nomura et al., 2019). These microtubule-associated proteins are spread along the outside of the microtubules and bind directly to the tubulin dimers of the lattice. The way in which the MAPs add support to the microtubules is by acting as ‘glue' and ‘support beams' of sorts, which will prevent dynamic instability at various points in the microtubules (Itoh & Hotani, 1994). Dynamic instability is when individual microtubules alternate between growing and rapid shortening phases (Itoh & Hotani, 1994). This constant polymerization and depolymerization of microtubules in the absence of MAPs can sometimes lead to a complete depolymerization of the microtubule, in which all tubulin dimers detach from each other, disassembling the lattice they formed (Fig. 28). The MAPs binding to the tubulin dimers will act as a sort of glue, holding them together. Furthermore, the absence of microtubules throughout the microtubule may cause some structural “weak points” where the dimers are at a higher risk of depolymerizing, leading to a breakage in the microtubule, ultimately reducing the strength of the microtubule and subjecting it to a higher risk of breakage upon bending stresses. Think of the MAPs as beams into which drywall (tubulin dimer lattice) is screwed. Without the stability of the beams, the drywall is flimsy and can easily be broken upon application of light stress. With the beam, however, the drywall becomes sturdier and much more difficult to break. These “beams” stabilizing the microtubules add to their robustness and capability of being repetitively subjected to extreme bending forces without breaking.

Figure 28 MAPs bound to microtubules. (A) depicts the stabilized, supported microtubule to which MAPs are bound. (B) depicts the dissociation of the MAPs from the microtubule, leading to the depolymerizaton of the microtubule and reduced microtubule stability (Kavallaris et al., 2008).
The haptonema has several crucial roles to carry out in coccolithophores and to be able to do all of them, they need to be strong and flexible. The rigidity and versatility of unaltered microtubules give the haptonema the strength it requires but are prone to breakage due to the extremely high degrees of curvature they endure during the process of lightning-fast coiling for obstacle avoidance or gradual bending for prey capture. Thanks to the tubulin code and microtubule-associated proteins, however, the microtubules have added flexibility, stability, and support which changes them from a rigid structure to a flexible and strong one. This allows the microtubules to repeatedly bend beyond their limits and gives the haptonema the range of motion it requires to carry out its tasks and be an asset to the organism. The flexibility of the microtubules in the haptonema also supports the interesting fact that native state matter is more flexible than fixed organic matter, which is beyond the scope of this paper, but a fascinating observation, nonetheless.
Conclusion
The study of the chemical properties of coccolithophores provides much insight into the organism's morphology, interactions with its environment, and evolutionary adaptations. Coccolithogenesis is a complex intracellular process regulated by coccolith-associated polysaccharides and their uronic acids, which coccolithophores have used to their advantage to adapt to changing environmental conditions, such as the CO2 levels of the ocean. The calcification of the coccoliths is also closely related to the phenomenon of photosynthesis. While photosynthesis provides the energy necessary for coccolith formation, calcification supplies the quantity of CO2 necessary for photosynthesis, with calcification seen as a very energy-efficient CO2 concentrating mechanism. This relationship has allowed coccolithophores to thrive in various pH conditions and different intracellular dissolved inorganic carbon concentrations. Coccolithophores also use endocytosis to draw calcium ions from their external environment to reach the necessary concentration in their coccolith vesicle for coccolithogenesis to occur. This process is essential to coccolithophore survival and presents a truly amazing design solution. The use of calcification to form coccoliths makes use of high environmental concentrations of Ca2+, not only concentrating CO2 within the cell for use in photosynthesis but also providing a somewhat shield in the form of a coccosphere, protecting the cells from mechanical stress, as discussed in “The Physical Properties and Interactions of Coccolithophores”.
Forming vast bloom would indicate that the coccolithophores have evolved towards optimizing growth rate and nutrient intake. This would not be possible if they also need to develop virus resistance, especially against the highly infected EhV. To maximize growth rate, coccolithophores instead have adopted a “virus-escaping” skill, ensuring that their genetic material is passed on to the next generation. By transferring to their haploid state, where the cell is fully encased by an organic shielding layer, viruses are not able to penetrate the cell! How ingenious! This way, coccolithophores may focus on blooming without worry of their genetic information being completely wiped out by viral infections. Another important feature of coccolithophores is the vestigial haptonema which is used for maneuvering and food accumulation. These applications require the appendage to move swiftly, be flexible, and endure strong forces. Made of microtubules, which are very rigid in nature, the vestigial haptonema should not be able to endure this pressure, though, coccolithophores have devised numerous solutions, such as the tubulin code and integrated microtubule-associated proteins, providing the required strength and flexibility to the organelle. Without these chemical and biological augmentations, the appendage would prove useless and coccoliths would not be able to sequester needed food or avoid obstacles.
Coccolithophores have developed many amazing design solutions throughout the millions of years they have been on Earth. They have become extremely versatile and adaptable creatures, having existed in so many different oceanic environmental regimes. However, the onset of ocean acidification through the rapid production of anthropogenic CO2 poses a severe risk for the unicellular organism. Hopefully, coccolithophores will be able to adapt as they have in the past and show resiliency by modulating their internal reactions. However, with ocean acidity levels changing so rapidly, this group of species may not have the time to adapt, potentially losing their ability to form their ever-important coccolith and coccosphere, thus exposing delicate naked cells to the rough ocean environment. With the loss of coccolithophores, we lose the base of our marine food chains, we lose an integral part of the carbon and calcium geochemical cycles, and we lose an ingeniously designed, and gorgeous group of organisms.
References
Balch, W. M., Bates, N. R., Lam, P. J., Twining, B. S., Rosengard, S. Z., Bowler, B. C., Drapeau, D. T., Garley, R., Lubelczyk, L. C., & Mitchell, C. (2016). Factors regulating the Great Calcite Belt in the Southern Ocean and its biogeochemical significance. Global Biogeochemical Cycles, 30(8), 1124-1144.
Ben-Joseph, O., de Haan, D., Rechav, K., Shimoni, E., Levin-Zaidman, S., Langer, G., Probert, I., Wheeler, G. L., & Gal, A. (2023). Crystallization of Coccolith Calcite at Different Life‐Cycle Phases Exhibits Distinct Degrees of Cellular Confinement. Small structures, 4(7), 2200353.
Books, C., Corner, E., Geis, I., & Goodsell, D. Molecule of the Month: Carbonic Anhydrase.
Brownlee, C., Langer, G., & Wheeler, G. L. (2021). Coccolithophore calcification: Changing paradigms in changing oceans. Acta Biomaterialia, 120, 4-11. https://doi.org/https://doi.org/10.1016/j.actbio.2020.07.050
Brownlee, C., Wheeler, G. L., & Taylor, A. R. (2015). Coccolithophore biomineralization: New questions, new answers. Seminars in cell & developmental biology,
Eikrem, W., Medlin, L. K., Henderiks, J., Rokitta, S., Rost, B., Probert, I., Throndsen, J., & Edvardsen, B. (2017). Haptophyta. In J. M. Archibald, A. G. B. Simpson, & C. H. Slamovits (Eds.), Handbook of the Protists (pp. 893–953). Springer International Publishing. https://doi.org/10.1007/978-3-319-28149-0_38
Frada, M., Probert, I., Allen, M. J., Wilson, W. H., & De Vargas, C. (2008). The “Cheshire Cat” escape strategy of the coccolithophore Emiliania huxleyi in response to viral infection. Proceedings of the National Academy of Sciences, 105(41), 15944-15949. https://doi.org/10.1073/pnas.0807707105
Gattuso, J.-P., Magnan, A., Billé, R., Cheung, W. W. L., Howes, E. L., Joos, F., Allemand, D., Bopp, L., Cooley, S. R., Eakin, C. M., Hoegh-Guldberg, O., Kelly, R. P., Pörtner, H.-O., Rogers, A. D., Baxter, J. M., Laffoley, D., Osborn, D., Rankovic, A., Rochette, J., . . . Turley, C. (2015). Contrasting futures for ocean and society from different anthropogenic CO2 emissions scenarios. Science, 349(6243), aac4722. https://doi.org/doi:10.1126/science.aac4722
Hardinger, S. A. Uronic acid. In Illustrated Glossary of Organic Chemistry: Institute for Reduction of Cognitive Entropy in Organic Chemistry.
Itoh, T. J., & Hotani, H. (1994). Microtubule-Stabilizing Activity of Microtubule-Associated Proteins (MAPs) is Due to Increase in Frequency of Rescue in Dynamic Instability: Shortening Length Decreases with Binding of MAPs onto Microtubules. Cell Structure and Function, 19(5), 279–290. https://doi.org/10.1247/csf.19.279
Janke, C. (2014). The Tubulin Code: Molecular Components, Readout Mechanisms, and Functions. Journal of Cell Biology, 206(4), 461–472. https://doi.org/10.1083/jcb.201406055
Janke, C., & Magiera, M. M. (2020). The Tubulin Code and its Role in Controlling Microtubule Properties and Functions. Nature Reviews Molecular Cell Biology, 21(6), 307–326. https://doi.org/10.1038/s41580-020-0214-3
Johnson, R., Langer, G., Rossi, S., Probert, I., Mammone, M., & Ziveri, P. (2022). Nutritional response of a coccolithophore to changing pH and temperature. Limnology and Oceanography, 67(10), 2309-2324. Kavallaris, M., Don, S., & Verrills, N. M. (2008). Microtubule-Associated Proteins and Microtubule-Interacting Proteins. In T. Fojo (Ed.), The Role of Microtubules in Cell Biology, Neurobiology, and Oncology (pp. 83–104). Humana Press. https://doi.org/10.1007/978-1-59745-336-3_4
Kavallaris, M., Don, S., & Verrills, N. M. (2008). Microtubule-Associated Proteins and Microtubule-Interacting Proteins. In T. Fojo (Ed.), The Role of Microtubules in Cell Biology, Neurobiology, and Oncology (pp. 83–104). Humana Press. https://doi.org/10.1007/978-1-59745-336-3_4
Kottmeier, D. M., Chrachri, A., Langer, G., Helliwell, K. E., Wheeler, G. L., & Brownlee, C. (2022). Reduced H+ channel activity disrupts pH homeostasis and calcification in coccolithophores at low ocean pH. Proceedings of the National Academy of Sciences, 119(19), e2118009119. https://doi.org/doi:10.1073/pnas.2118009119
Lee, R. B. Y., Mavridou, D. A. I., Papadakos, G., McClelland, H. L. O., & Rickaby, R. E. M. (2016). The uronic acid content of coccolith-associated polysaccharides provides insight into coccolithogenesis and past climate. Nature Communications, 7(1), 13144. https://doi.org/10.1038/ncomms13144
Mackinder, L. C. M., Worthy, C. A., Biggi, G., Hall, M., Ryan, K. P., Varsani, A., Harper, G. M., Wilson, W. H., Brownlee, C., & Schroeder, D. C. (2009). A unicellular algal virus, Emiliania huxleyi virus 86, exploits an animal-like infection strategy. Journal of General Virology, 90(9), 2306-2316. https://doi.org/10.1099/vir.0.011635-0
Marsh, M. (2003). Regulation of CaCO3 formation in coccolithophores. Comparative Biochemistry and Physiology Part B: Biochemistry and Molecular Biology, 136(4), 743-754.
Marzec, B., Walker, J. M., Panagopoulou, M., Jhons, Y., Clare, D., Wheeler, A., Shaver, M. P., & Nudelman, F. (2019). Three-dimensional architecture and surface functionality of coccolith base plates. Journal of Structural Biology, 208(2), 127-136. https://doi.org/https://doi.org/10.1016/j.jsb.2019.08.007
Nomura, M., Atsuji, K., Hirose, K., Shiba, K., Yanase, R., Nakayama, T., Ishida, K., & Inaba, K. (2019). Microtubule Stabilizer Reveals Requirement of Ca2+-Dependent Conformational Changes of Microtubules for Rapid Coiling of Haptonema in Haptophyte Algae. Biology Open, bio.036590. https://doi.org/10.1242/bio.036590
Paasche, E. (2001). A review of the coccolithophorid Emiliania huxleyi (Prymnesiophyceae), with particular reference to growth, coccolith formation, and calcification-photosynthesis interactions. Phycologia, 40(6), 503-529.
Pomar, L. (2020). Chapter 12 – Carbonate systems. In N. Scarselli, J. Adam, D. Chiarella, D. G. Roberts, & A. W. Bally (Eds.), Regional Geology and Tectonics (Second Edition) (pp. 235-311). Elsevier. https://doi.org/https://doi.org/10.1016/B978-0-444-64134-2.00013-4
Raad, A., & Abdulmajeed, B. (2018). Cultivation of Microalgae Using Industrial Dairy Wastewater
Ramazi, S., & Zahiri, J. (2021). Post-Translational Modifications in Proteins: Resources, Tools and Prediction Methods. Database, 2021, baab012. https://doi.org/10.1093/database/baab012
Rickaby, R. E. M., Hermoso, M., Lee, R. B. Y., Rae, B. D., Heureux, A. M. C., Balestreri, C., Chakravarti, L., Schroeder, D. C., & Brownlee, C. (2016). Environmental carbonate chemistry selects for phenotype of recently isolated strains of Emiliania huxleyi. Deep Sea Research Part II: Topical Studies in Oceanography, 127, 28-40. https://doi.org/https://doi.org/10.1016/j.dsr2.2016.02.010
Sand, K. K., Pedersen, C. S., Sjöberg, S., Nielsen, J. W., Makovicky, E., & Stipp, S. L. S. (2014). Biomineralization: Long-Term Effectiveness of Polysaccharides on the Growth and Dissolution of Calcite. Crystal Growth & Design, 14(11), 5486-5494. https://doi.org/10.1021/cg5006743
Sheyn, U., Rosenwasser, S., Ben-Dor, S., Porat, Z., & Vardi, A. (2016). Modulation of host ROS metabolism is essential for viral infection of a bloom-forming coccolithophore in the ocean. The ISME Journal, 10(7), 1742-1754. https://doi.org/10.1038/ismej.2015.228
Tyrrell, T., & Young, J. (2009). Coccolithophores.
Villareal (2007), M. R. Pinocytosis. In.
https://commons.wikimedia.org/wiki/File:Endocytosis_types.svg
Walker, J., & Langer, G. (2021). Coccolith crystals: Pure calcite or organic-mineral composite structures? Acta Biomaterialia, 125, 83-89.
Weier, J. (1999). What is a Coccolithophore? https://earthobservatory.nasa.gov/features/Coccolithophores
Westbroek, P., De Jong, E. W., Van Der Wal, P., Borman, A. H., De Vrind, J. P. M., Kok, D., De Bruijn, W. C., Parker, S. B., Miller, A., Phillips, D. C., & Williams, R. J. P. (1984). Mechanism of calcification in the marine alga Emiliania huxleyi. Philosophical Transactions of the Royal Society of London. B, Biological Sciences, 304(1121), 435-444. https://doi.org/doi:10.1098/rstb.1984.0037 Zhang, Y., & Gao, K. (2021). Photosynthesis and calcification of the coccolithophore Emiliania huxleyi are more sensitive to changed levels of light and CO2 under nutrient limitation. Journal of Photochemistry and Photobiology B: Biology, 217, 112145.