Copepods Through the Lens of Physics
Johanna Turton, Melodi Rousset, Philippe Mercier, Tanjin Sultana
Abstract
This research paper will present copepods from a physics approach while focusing on three key aspects. To begin, the low Reynolds number environment in which copepods reside makes them face entirely different challenges than larger organisms when it comes to the basics for survival like moving, eating, detecting, and communicating. To overcome these challenges, copepods master the control of surrounding water, which allows them to create feeding currents and move in an energy-efficient manner. Secondly, the copepod's intricate optical nanostructures characterize their structural coloration. This paper will show how copepods hone the optical properties of their morphology to skillfully evade predators through camouflage and attract mates for successful reproduction. Lastly, this essay will present an in-depth explanation of hydrodynamics mechanoreception on which depends the copepod's feeding and protection strategies. To truly understand this mechanism and how copepods use their surrounding environment, we will dive into an examination of water's physical properties. The insights presented in this paper offer a comprehensive look into the distinctive design of copepods.
Introduction
At the core of the ocean's vast system of interdependence lies copepods, a group of aquatic crustaceans distinguished by their adaptable nature, morphological diversity, and ecological significance who have been roaming the oceans since the late Paleozoic Era around 400 million years ago. In the depths of the marine world, copepods can be found as free-living, symbiotic, or parasitic organisms, playing a role that goes undetected to the naked eye and is often overlooked. These creatures constitute 70 to 80% of zooplankton and are the crucial intermediary species in the food chain (Davies et al., 2022). Copepods, with more than 10 000 identified species (Walter & Boxshall, 2013), represent the link that connects the photosynthesizing phytoplankton to the remainder of larger aquatic life, upon which the marine food web depends. As our understanding of climate change deepens and the consequences intensify, the role of the ocean as a climate regulator becomes clear and copepods contribute to its efficiency (Steinberg & Landry, 2017).
To begin understanding copepods, it is important to unravel their origins. Copepods have endured 400 million years and seen the rise and fall of many species. Their estimated divergence during the Paleozoic Era was approximated through a phylogenomic approach utilizing 24 nuclear protein-coding genes, as explained by Eyun research team (Eyun, 2017). Copepods continue to evolve to this day into different orders and species within the copepod group, as illustrated in Figure 1. There is a great morphological diversity within the group of copepods which can be seen in Figure 2. Sister groups sharing common ancestors with copepods, such as Branchiopoda (comprising brine shrimp and water fleas), Insecta (encompassing fruit flies and red floor beetles), and Malacostraca (including mantis shrimp and skeleton shrimp), offer insight into the roots of copepods (Eyun, 2017; Schwentner et al., 2017). Despite their prominence, the copepod fossil record remains relatively limited, with the oldest known fragments dating back to around 303 million years ago, discovered within a bitumen clast in a glacial diamictite in eastern Oman in 2010 (Selden et al., 2010). Remarkably, these fossil findings align with the previously stated phylogenomic estimations and the current academic literature, providing a solid foundation for our understanding of copepod evolution. One common characteristic that unites all copepods is their pair of antennae that gives them the power of hydrodynamic mechanoreception. With their versatile sensory system, they detect water flow, pressure, and turbulence to adapt to their dynamic environment and survive for 400 million years ago.

Figure 1: Phylogenetic tree of copepods. (Eyun, 2017)

Figure 2: Illustration of morphological diversity of copepods. (Bron et al., 2011)
In this review paper, we will explore how copepods dominate in the pelagic water column by manipulating the physical properties of their environment to ensure not only their survival but also their prosperity. This essay will strive to explain the interactions between copepods and their surroundings and how copepods manipulate the latter to fulfill every aspect of their survival: protection, reproduction, sustenance, or navigation.
Forces acting on copepods
As we take a closer look at the numerous fascinating species of copepods, it is important to understand the forces that are acting upon them in their environment. The main forces discussed are inertial forces, viscous forces, shear forces, gravity, and drag force.
Inertial forces
Inertial forces and momentum are very closely related concepts. In fluid mechanics, inertial forces work against any accelerating force – e.g., viscous forces –, either to keep a fluid or an object at rest, or to keep a fluid or an object's momentum (Belhaj, 2023). For that reason, inertial forces are sometimes referred to as momentum forces. In other words, inertial forces are the forces that cause any object having a mass to resist to changes in its state of movement (Butto, 2021).
Shear forces
Shear forces, as their name implies, are opposite forces that create a shear stress (Alameer et al.). For example, the skin of an animal swimming in the water is subject to shear forces, since its body's inertial forces are pushing the fish and its skin forward, while the friction from the contact with water is pulling its body backwards (see Figure 3).

Figure 3:Schematic representation of shear forces on a fish's skin. (Mercier, 2023)
Viscosity/viscous forces:
Viscosity, for its part, is the resistance of a medium to gradual deformation under shear stress. Viscous forces represent the friction forces between neighbouring particles/layers of fluid, or between a fluid and a solid passing through it (Hack, 2018). They are thus parallel to the fluid's movement. Hence, the more viscous a liquid, the more friction there is between its layers. Viscosity can be measured in Newton-second per meter squared, which confirms viscous forces' proportionality to area (Hack, 2018).
Drag forces
Drag forces can be understood as being the forces working against the relative movement of an object and the fluid it is in (Maxemow, 2009). It usually regroups both the effect of the shear stress caused by friction between the object and the fluid it is in – which depends on the viscosity of the liquid – and the normal stress on the surface of the object due to pressure, called pressure drag (Wang & Wang, 2022).
The effects of living at low Reynold numbers
Copepods' size generally lies in the 0.05-2mm range (García-Comas et al., 2016), and are thus said to live at low Reynold numbers – ranging from 10-2 to 103 (Yen, 2000) – compared to bigger organisms like whales for example – which live at Re of 106-108 (Vogel, 2008). Reynold numbers represent the ratio between the inertial forces and the viscous forces in a system – which can correspond to an object moving in a liquid, for example. Denoted Re, it is given by the following equations (Purcell, 1977):
η: viscosity, ρ: fluid density, υ: velocity of the object moving through the liquid, α: dimension of the object moving through the liquid
This means that the viscous forces acting on copepods – which slow them down to rest when they stop swimming – are of much greater magnitude than the inertial forces that keeps them going forward in water after being pushed in that direction. In other words, for these animals, the concept of inertia is irrelevant: their current movement is fully determined by the forces that are applied on their body at the very moment (Purcell, 1977). If they stop swimming, the delay before they reach a resting position relative to the water around them is negligible compared to bigger organisms like whales in the same situation (Purcell, 1977). This makes sense, since after all, according to the scaling law, whales have much more mass per unit of area than copepods (Soh, 2013; “Studying Cells – Cell Size,” (2022, June 8)). In short, if we were progressively reduced to the size of a copepod, swimming through water would feel more and more like swimming through thick liquids like honey or molasses.
Furthermore, the low Reynold numbers at such small magnitudes makes it such that moving through water increases the chances of encounter with food by a lot less than it would at high Reynold numbers (Purcell, 1977). After all, if the Reynold numbers are low for copepods, they also are for the particles that they eat – even more, since what they eat is much smaller than them – from 11 to 87 micrometer (Frost, 1972). When an object moves forward through water, it pushes the water in front of it forwards and on its sides – it must take the water's place in space. The lower the Reynold number for a particle sitting in this water, the more the particle will follow the water as it moves – since low Reynold numbers mean that friction between water and the particle is much stronger than the particle's inertial forces. This means that the object will have to swim longer towards the particle before they touch as the Reynold numbers for the system get lower. Hence, not only do low Reynold numbers make it harder to swim through a liquid, but it also makes it less beneficial in terms of food encounter rate.
Living at low Reynold numbers thus pushed copepods to evolve skills other than swimming as fast and as long as possible to efficiently find and eat food. One of them is the creation of flow fields and their manipulation to detect and move food particles like algae towards and away from them, such that themselves are required to move less to eat as much.
Copepods generation of flow fields
Copepods create many types of flow fields. In fluid mechanics, flow fields describe the velocity of every particle of fluid in a region (see Figure 4).
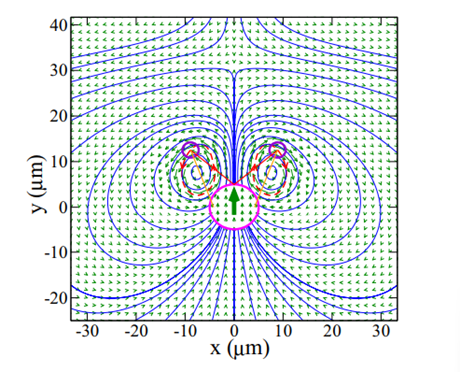
Figure 4:Flow field around a micro flagellar-beating swimmer (Jibuti et al., 2017)
The ones used to detect and capture food particles – which combine to create what is called a “feeding current” – will be discussed in this section.
To generate a feeding current, copepods use two pairs of appendages, which they move at high frequencies in a “clap and fling” pattern, which is their solution to the challenge of moving water efficiently at low Reynolds numbers (Jiang, 2002). The water movement generated by this pattern creates four vortices – see Figure 5. Together, these form a sort of funnel, where the bigger ones catch more food particles and direct them towards the smaller ones. These, which form a double shear field, in turn make the food pass close enough to the mouth parts for the copepods be able to catch and eat them (Jiang, 2002).

Figure 5: Flow field (feeding current type) around a copepod (Krils, n.d.)
It has also been observed that, even though these flows fields are affected by several variables such as external forces, body orientation, and velocity, copepods manage to keep them stable enough such that when an alga enters them, they notice the disturbance in the flow field and are able to modify it as to guide the algae more directly towards their mouth, detect predators and locate mates (Strickler, 1982). This process is called mechanoreception. Copepods have antennae with fine hair-like structures called setae that are highly sensitive to water vibrations. They can detect changes in water flow and pressure caused by nearby organisms. When changing water interacts with the setae exteroceptive transduction is initiated which is when the body obtains information from the external environment and responds to it (Shen et al., 2020). Setae contain mechano-gated microchannels which are ion channels with membrane proteins capable of the responding to the mechanical stimulation from the waves onto the setae. When this occurs, neural signals are produced and the copepod can activate its colour changing abilities to respond to the surrounding movement (Chen et al., 2018). Some species of copepod have specialised organs that offer the same detection the setae does.
“Clap and fling” mechanism
The “clap and fling” – or Weis-Fog effect – is a mechanism that has first been observed in insects so small that they face locomotion challenges similar to copepods': they live at such tiny Reynold numbers that it is completely inefficient for them to use larger organisms' tricks to move through fluid, such as larger insects or birds' way of flying. The major difference between them and copepods is that the fluid they live in is air, not water, but the logic is similar.
At low Reynold numbers, the advantage of the “clap and fling” method of moving fluid over others is that it attenuates considerably – if it does not remove – the Wagner effect (Sane, 2003). Briefly, the Wagner effect is the principle by which vortices of fluid are created at the trailing edges of wings starting to move from rest in a fluid, vortices which slow down the growth of the circulation of the fluid around the wings as they move, making the latter reach their maximum circulation slower (Sane, 2003). This sluggishness in the development of circulation of fluid around the wing slows down the whole process of moving this fluid as to allow flight or swimming in it, making these processes less efficient.
However, since wings and copepods appendices move in pair and in opposite directions, the vortices they create when starting to move from rest are also in opposite directions. Hence, if the trailing edge of the wings start to move from rest at the same place, the two vortices they create annihilate each other, and their negative effect thus vanishes (Sane, 2003). This is the particularity of the “clap and fling” mechanism. Wings functioning according to it do not stop their course like we are accustomed to wings doing it in birds: they continue until they touch each other – or barely do so, depending on the organism – which makes them start from the “same point”, cancelling the Wagner effect, and allowing circulation to build up faster around the wings – see Figure 6, E and F (Sane, 2003). This allows for a higher frequency of beating, which increases the lift generation for wings, and the propulsion for swimmers.
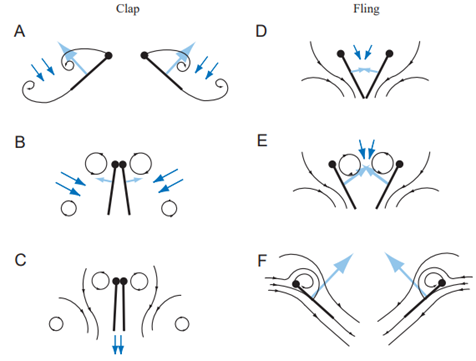
Figure 6:The “clap and fling” mechanism (Sane, 2003)
Note that the “clap and fling” method does not only generate more lift than the other conventional methods for small organisms. This is why it can be observed in some animals like pigeons during takeoff – which explains the flapping sound heard when they start flying (Le, 2012). This brings up questions as to why not all flying organisms use it. In fact, it is only used by some larger animals when they need to accomplish demanding tasks that conventional ways of flying would not allow them to do – such as taking off fast or carrying heavy loads – because while it can provide more lifting in the same amount of time, it is less energy efficient than these other flying mechanisms – like lift generation from airfoil – at high Reynold numbers (Chin & Lentink, 2016). However, as Reynold numbers decrease, the Wagner effect grows more and more intense (Sane, 2003), which means solutions such as lift generation from airfoil lose their efficacity drastically (Winslow et al., 2018), while the “clap and fling” method's advantage become more pertinent. This is why very small animals – like copepods – use it to move fluids, and through them, while bigger ones generally do not.
In short, copepods cope with low Reynold numbers by using the “clap and fling” mechanism – a way to move water that is optimized for low Re environments – to create flow fields and feeding currents, which are themselves an optimized way to increase their encounter rate with food specifically in low Re environments.
Other physical particularity of the flow field creation process at low Reynold numbers
It is also interesting to notice that, during the propulsive strokes that copepods make to move water around, their setae – which is the sort of hair-like structure present on copepods appendages – spreads out just like humans' skin hair when we get “goose bumps”. This increases the contact area with water, allowing to move more of it in a single stroke. Inversely, the setae are held close to the appendages during a recovery stroke to reduce its drag, making it less counterproductive a movement (Jiang, 2002).
However, at low Reynold numbers – where inertia can be neglected – this effect is amplified. In fact, when a body moves through a fluid, the particles of fluid that touch it are subject to friction and are pulled along with it – they do not reach the object's speed, but the get close to it. The next layer of fluid is similarly pulled, and so on until the pull is completely dissipated. This forms a layer of fluid that substantially “sticks” to the object, which is called a “boundary layer” (Koehl & Strickier, 1981). The more viscous a fluid – or the lower the Reynold number of the system –, the more friction there is between each infinitesimal layer of fluid, and so the larger this boundary layer is. In a system where two identical objects move in parallel at the same speed in water – e.g., copepods' setae –, the water will thus stop flowing between the objects if half the distance between them is smaller than their boundary layer. Since copepods live at such low Re, the boundary layers of their setae are large enough for this phenomenon to happen. Hence, when they are spread out during a propulsive stroke, copepods' setae behave like solid paddles instead of open racks (Koehl & Strickier, 1981).
This understanding of boundary layer further justifies the copepods' need for flow fields in order to manipulate and catch algae: just flapping their appendage towards food to catch it only pushes it forward, due to the very large boundary layer at low Re (Koehl & Strickier, 1981).
Being at a low Reynolds number also means that the flow created by copepods' appendage beating is laminar, due to the high level of friction between the layers of fluid relative to their inertial forces, which causes them to stay more organized. This laminar characteristic of the flow fields is useful in terms of prey detection, since it allows for less disturbance of the chemical signals made by prey – less stirring of the water in which it is –, signals which provide information about their location (Koehl & Strickier, 1981). This will be further discussed in a companion paper (Sultana et al., 2023).
Copepods through the lens of optics and waves:
When looking at copepods through the lens of optics and waves we see how their biology and behaviour is deeply connected to the physics of light and water. They are a relatively translucent species which allows them to change colour in a variety of methods. Their specific refractive index and structure effect the behaviour of light, they can scatter light, and can detect waves. Each of these attributes are what make copepods as predominant as they are and can give us insight into physical concepts that they manipulate to their advantage.
There are three main ways the different species of copepods emit their colour: structural coloration, bioluminescence, and pigmentation. The individual or multiple methods used is dependent on the specific species of copepod and their environment.
Some species of copepod can adjust their colouring due to layers of guanine crystal plates within the dorsal aspect of the copepods. The specific family examined by Kimura et al. in an article that discussed the guanine crystals is the Sapphirinid copepods and therefore we will focus on that species in this section (Kimura et al., 2020). These guanine crystal plates are arranged in a honeycomb pattern with very specific dimensions and microscopic details that are the key to their colour changing abilities (Figure 7). The framework is made of chitin which is a very strong substance and the main component of exoskeleton. The plates are roughly 1.5 µm wide and 80 nm thick. Depending on what part of the copepod body we are looking at, it will have a different colour. This difference is due to minor variations in the dimensions of the guanine plates. Copepods have schematic sections of colour portions; each portion has a similar structure with micro (70 to 100 µm) differences that attribute to the different colour of each portion as shown in Figure 7. Within each portion the guanine crystal arrays are stored in the highly reflective iridophore cells. Iridophore cells are stacks of very thin cells that reflect light and simultaneously change their wavelength. The wavelength change that occurs is completely dependent on the angle at which the incident ray hits them (Gilmore, 2016). It is a combination of the iridophore cells and the changing distance between the guanine plates that contribute to the reflection of the desired colour. When changing the inter-plate distance from a range of 100 to 30 nm, the copepod will change from blue to purple to yellow. By having minor changes in the shape of the guanine crystal plates, light will refract differently off each of them. Within the array, there are 8 layers of guanine plates. This was found experimentally to be the ideal number of layers as any more does not have an impact on how the incident light is reflected. This explains why the number of layers is relatively universal among species of copepods that have guanine crystal plates, as any fewer layers distorts the purity of the colour by decreasing the intensity. Less photons contribute to the emitted light source because they are absorbed into the extra layers. Less photons means the light is less intense and therefore less bright. When light interacts with the plates, the combined effect of diffraction, refraction, reflection, and interference of light are what allows them to change colour.
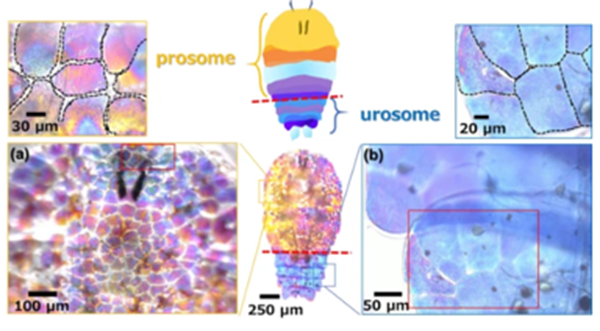
Figure 7: Behaviour of an incident light ray on Sapphirinid copepod (Kimura et al., 2020)
As an incident ray travels toward the Sapphirinid copepod it is first refracted as it enters the guanine plates. Its speed and wavelength change due to the higher refractive index in the copepods. These copepods have a refractive index of 1.83, compared to that of water which is 1.33 (Hirsch et al., 2017). The two different refractive indices means that light travels through two different mediums. By Fermat's Principle, when light travels through two different mediums it will change direction to travel through the new medium at the quickest speed. This change in angle traveled by the light can be represented through Snell's Law: n1sin(α) = n2sin(β). When light hits the different medium that is the guanine crystals, its angle of refraction changes as shown in Snell's Law. This results in a shorter wavelength to form as it begins to diffract.
As the incident light travels through the guanine plates, it is diffracted by their irregular shape. The amount of diffraction experienced by the light rays is dependent on the specific section of the guanine plate it is traveling through. As the light bends when it moves through the layers and hits the iridophore cells and is reflected. It then experiences even more diffraction as the new ray moves outward from the copepod's dorsal aspect.
When looking at multiple rays traveling through the new medium, they are refracted, diffracted, and reflected individually but also interact with each other. Their interaction leads to either constructive or destructive interference depending on the phase of the rays. When the rays are in phase the light's amplitudes combine with each other creating an even stronger light ray. This is known as constructive interference. Destructive interference is when the opposite occurs and light rays' amplitudes either cancel each other out completely or make the existing ray weaker. By the combination of light bending from the structure, angle of refraction, reflection, and a medium with a higher refractive index, light can be manipulated by the copepods to change their color.
While the refractive index of the guanine crystals is 1.83 for species like the Sapphirinid copepods, the tissues of other species of copepods have a refractive index very similar to that of water (1.33) and in combination with their size it allows them to be almost completely translucent. This means light can travel through the copepod tissue similarly to that of water which is what gives it its translucent appearance. It is a combination of these indices that allow copepods to almost have a glow type colour that is still relatively translucent. Temperature and salinity of their surroundings can also impact their tissue and therefore their refractive indices (Los Huertos, 2020).
Due to the small size of copepods, the wavelengths of light that interact with them are of relatively the same magnitude. This allows them to demonstrate Mie Scattering, which is when light encounters small particles and scatters elastically. Copepods are not only small but, as discussed, have an irregular body shape which allows the scattering at different angles. Different wavelengths scatter at different angles and contributes to their colour changing abilities (Sakınan & Gücü, 2016). The purpose of their colour changing property is primarily to attract the opposite sex of copepods, however it is also used for camouflaging with their environment to hide from predators.
What are the various mechanisms of copepods feeding?
In various bodies of water, copepods who are known to be aquatic omnivores play a significant role within the marine food chain due to the variety of plants and animals they eat. These organisms mostly feed on phytoplankton and zooplankton (“What do Copepods Eat? An Overview of the Diverse Diet of These Tiny Aq.,” 2022). Since they can eat many types of species, they can adapt to various types of environments which makes them abundant within oceans and lakes.
Feeding strategies
Due to copepods living in different areas of waters, some of the plankton have evolved to different feeding strategies depending on the availability of resources around them. In a paper written by Kiørboe et al. (2009), they found that copepods have developed two categories of feeding types: active cruising and ambushfeeding; or can demonstrate both if they have high behavioral plasticity (Holm et al., 2019). Active cruising refers to looking for food by roaming around the sea while ambush feeding refers to waiting to capture a prey quickly without them noticing. How they sustain themselves can also be dependent on their body structure. In the paper written by Kiorboe at al., the researchers worked on two types of zooplankters being Acartia tonsa and Oithona davisae. It's important to note that Acartia tonsa is a species that can switch between the two foraging methods whilethe latter who is bigger in sizeuses the surprise attack method. Their experiment revealed that ambush feeding is reserved for bigger copepods who can expend a lot of energy to jump high. In contrast, active cruising can be done by any type of copepod.
To continue further, it is important to understand how each of the mechanisms work. Within active cruising, once the prey is detected, they can generate a feeding current (see “Copepods' generation of flow fields”) or just simply attack prey while swimming.This occurs within waters that are at low Reynolds Number (Giuffre et al., 2019). For that reason, the fluid around the small species is quite viscous and doesn't allow the change in its shape. This is why copepods have developed flagella, feeding appendages and cilia (Kiørboe et al., 2009) to counter this thickness as they roam through bodies of water. The beating occurs at high frequencies that range from 20 to 80 Hz (Jiang & Osborn, 2004). The force of the beating on water is known as a stokeslet. Thisbalanced by gravity produces a feeding current (Kiørboe, 2013). An oscillatory velocity field is also created due to their beating in the water and when particles get caught in this field, they also move with this field with a velocity shift (Giuffre et al., 2019). The main characteristic of having appendages allows the organism to be self-propelled and their oscillatory movement generates a scanning current. Through chemoreception, which will be discussed in another paper, these pelagic feeders can detect the location and even what kind of particles are present within the current when they're at far lengths from possible prey (Giuffre et al., 2019). However, at closer distances, mechanoreception, where they sense the hydrodynamic disturbances generated by the preythrough their antennae plays a more crucial role in sensing their food (see “Copepods' generation of flow field”).
In contrast, ambush feeding is when copepods attack at high velocities in a short amount of time as recorded by Kiorboe at al. They can sense their prey via mechanoreception as mentioned in “Copepods' Generation of flow fields”. How it occurs is that their posterior swimming leg are lunged backward when the prey is approximately 0.2 mm of the first antenna (Kiørboe et al., 2009).
Furthermore, just like active cruising, a flow field is created when they jump, but what's most interesting is that the prey doesn't get pushed away significantly by this motion. It is found that ambush feeders tend to producer weak fluid signals over cruising copepods such as Temora longicornis due to being negatively buoyant (Kiørboe, 2013). When they jump upward, they generate a force that is backwards and forward within the water, otherwise known as an impulsive stresslet (Kiørboe, 2013) as demonstrated in Figure 10. With that in mind, through experimental observations found that the viscous layer around the copepod was weak and at the end of the jump, the thickness was equivalent to the maximum Reynolds number:
where L represents the body length, v the velocity, Umax the maximum potential of the attacking copepod, and it decreases as:

Figure 10: Ambush feeder, Acartia Tonsa jumping and creating an impulsive stresslet (Kiørboe, 2013).
Within environments where food is scarce, these types of feeders would thrive over the cruiser types because not as much energy is spent to move around waters (Holm et al., 2019). In the study done by Holm et al., they demonstrated (Figure 10), that these types of feeders along with switch feeders would survive longer than the feeding current copepods such as Temora longicornis.
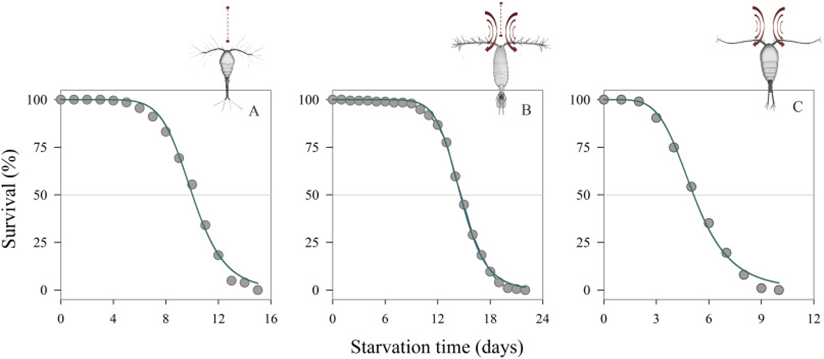
Figure 11: Survival time (%) of copepod females with different feeding behaviors in the absence of food: Oithona nana (strict ambush feeding) (a), Acartia tonsa (switching-behavior feeding) (b) and Temora longicornis (feeding-current feeding) (c) (Holm et al., 2019).
Despite this, cruisers are much more abundant because ambush feeding requires a lot of muscle power which is associated with bigger copepods (Kiørboe et al., 2009).
In contrast, within environments that are non-scarce, cruisers are also more abundant because foraging is an efficient strategy when there's food sources readily available.
Through this, it's observed how hydrodynamical disturbances are pertinent for copepods to survive in both types of feeding.
How do copepods respond to the various hydrodynamic disturbances with their antennae
Copepods are such fascinating species in that they do not sense things in the same nature as humans. These are blind organisms and thus rely on their antennae and other appendages to detect things within waters. These antennules contain mechanosensory setae (Figure 12), which are crucial for their understanding of their surroundings (Kiørboe, 2013). When a prey, possible mate, or predator is nearby, the signal can be picked up by the medium and the setae are able to deform to generate a neurological signal which can be used to generate responses such as escaping, attacking, or mating (Kiørboe, 2013). When the setal array bends, this can lead to exteroceptive transduction where the external signals convert to electrical signals through mechano-gated microchannels opening in copepods' mechanosensory dendrite (Shen et al., 2020). In the model done by Shen et al., the deformation of the seta can be calculated by first using the Resistive Force theory; a model that considers the viscous drag forces acting on microorganisms that move in fluids to describe their movement. This theory can explain why copepods produce a thrust through self-propulsion to reduce the resistance created by water (Shen et al., 2020). Euler Beam Theory is then applied to find the deformation due to the forces with limited constraints such as only looking at the changes in setae and the distributive forces along the setal axis. This theory is used to analyze the behaviour of slender structural beams in structural mechanics. However, copepods rely on fluid dynamics thus it cannot be directly applied, it is only used to model the deformation. They discovered through their mechanical model which only focused on the hydrodynamic effect on their body and the first antennae while ignoring the seta; that lower frequency signals are better detected and lead to a larger bend in the setae. In addition, depending on the length of the setae, short is for capturing high frequencies while long is for low. The authors also found that the signals are detected within short times.
Thus, these species are well-equipped in detecting what's in their surroundings even if they can't see through their setae.
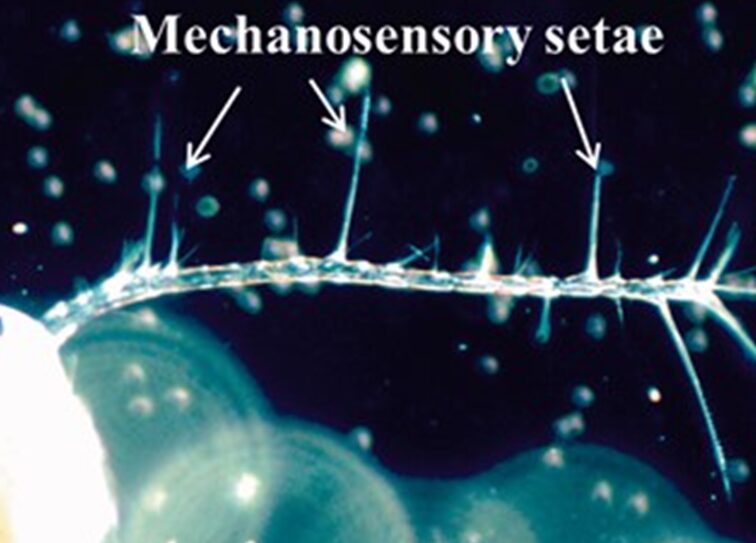
Figure 12 Mechanosensory setae on the antennules of copepods (Kiørboe, 2013).
Conclusion
In conclusion, copepods have evolved to become highly adaptive creatures to survive in low Reynold environments and overcome the physical obstacles of their habitat. Copepods have been designed with a multifaceted approach to overcome several challenges. To begin, the viscous and shear forces acting on copepods drive the design of effective and precise solutions to fulfill their needs for food, movement, protection, and mating. In this paper, it has been proven that the “clap and fling” mechanism emerges as a pivotal adaptation, allowing copepods to optimize fluid movement while nullifying an important obstacle being the Wagner effect which hinders other organisms. This unique and effective mechanism allows them to create flow fields and feeding currents, therefore substantially increasing their encounter rate with prey in low Reynold number environments. From the physical interactions between copepods and their surroundings, we move to the physical interactions taking place within them to explore their internal dynamics. On top of manipulating the water around them, they manipulate the physics of light with their unique optical characteristics. It has been demonstrated that copepods use structural coloration as a design solution to fulfill their survival needs, employing it for camouflage against predators and for mate attraction. Copepods are the perfect illustration of nature's ingenuity revealing how organisms reshape their behaviors and morphology to thrive in challenging environments. These tiny creatures have provided valuable insights into fluid dynamics and bioengineering, shedding light on the fascinating interaction of forces in the depths of our natural world.
References
Alameer, A., Almansour, H., & Saatcioglu, M. CAPACITY OF HYBRID REINFORCED UHPC BEAMS IN FLEXURE AND SHEAR.
Belhaj, H. A. (2023). Chapter 6 – Dynamic modeling of tight unconventional reservoirs. In H. A. Belhaj (Ed.), Tight Oil Reservoirs (Vol. 1, pp. 157-210). Gulf Professional Publishing. https://doi.org/https://doi.org/10.1016/B978-0-12-820269-2.00003-2
Bron, J. E., Frisch, D., Goetze, E., Johnson, S. C., Lee, C., & Wyngaard, G. A. (2011). Observing copepods through a genomic lens. Frontiers in Zoology, 8(1), 22. https://doi.org/10.1186/1742-9994-8-22
Butto, N. (2021). The Nature and Origin of Inertia. Journal of High Energy Physics, Gravitation and Cosmology, 7(02), 761.
Chen, H., Jing, L., Teng, Y., & Wang, J. (2018). Characterization of antibiotics in a large-scale river system of China: Occurrence pattern, spatiotemporal distribution and environmental risks. Sci. Total Environ., 618, 409-418. https://doi.org/10.1016/j.scitotenv.2017.11.054
Chin, D. D., & Lentink, D. (2016). Flapping wing aerodynamics: from insects to vertebrates. Journal of experimental biology, 219(7), 920-932.
Davies, C. H., Beckley, L. E., & Richardson, A. J. (2022). Copepods and mixotrophic Rhizaria dominate zooplankton abundances in the oligotrophic Indian Ocean. Deep Sea Research Part II: Topical Studies in Oceanography, 202, 105136. https://doi.org/https://doi.org/10.1016/j.dsr2.2022.105136
Eyun, S.-I. (2017). Phylogenomic analysis of Copepoda (Arthropoda, Crustacea) reveals unexpected similarities with earlier proposed morphological phylogenies. BMC Evolutionary Biology, 17(1). https://doi.org/10.1186/s12862-017-0883-5
Frost, B. (1972). Effects of size and concentration of food particles on the feeding behavior of the marine planktonic copepod Calanus pacificus 1. Limnology and Oceanography, 17(6), 805-815.
García-Comas, C., Lee, Y.-C., Chang, C.-Y., Gong, G.-C., & Hsieh, C.-h. (2016). Comparison of copepod species-based and individual-size-based community structuring. Journal of Plankton Research, 38(4), 1006-1020.
Giuffre, C., Hinow, P., Jiang, H., & Strickler, J. R. (2019). Oscillations in the near-field feeding current of a calanoid copepod are useful for particle sensing. Scientific reports, 9(1), 17742.
Hack, H. (2018). Viscosity. In (pp. 926-928). https://doi.org/10.1007/978-3-319-73568-9_308
Holm, M. W., Rodríguez-Torres, R., Hansen, B. W., & Almeda, R. (2019). Influence of behavioral plasticity and foraging strategy on starvation tolerance of planktonic copepods. Journal of experimental marine biology and ecology, 511, 19-27.
Jiang, H. (2002). The flow field around a freely swimming copepod in steady motion. Part I: Theoretical analysis. Journal of Plankton Research, 24(3), 167-189. https://doi.org/10.1093/plankt/24.3.167
Jiang, H., & Osborn, T. R. (2004). Hydrodynamics of copepods: a review. Surveys in geophysics, 25, 339-370.
Jibuti, L., Zimmermann, W., Rafaï, S., & Peyla, P. (2017). Effective viscosity of a suspension of flagellar-beating microswimmers: Three-dimensional modeling. Physical Review E, 96(5), 052610.
Kiørboe, T. (2013). Attack or attacked: the sensory and fluid mechanical constraints of copepods' predator–prey interactions. In: Oxford University Press.
Kiørboe, T., Andersen, A., Langlois, V. J., Jakobsen, H. H., & Bohr, T. (2009). Mechanisms and feasibility of prey capture in ambush-feeding zooplankton. Proceedings of the National Academy of Sciences, 106(30), 12394-12399.
Koehl, M., & Strickier, J. R. (1981). Copepod feeding currents: Food capture at low Reynolds number 1. Limnology and Oceanography, 26(6), 1062-1073.
Le, M. (2012). An unconventional lift-enhancing mechanism: Clap and fling: Bio-Aerial Locomotion.
Maxemow, S. (2009). That's a drag: The effects of drag forces. Undergraduate Journal of Mathematical Modeling: One+ Two, 2(1), 4.
Purcell, E. M. (1977). Life at low Reynolds number. American journal of physics, 45(1), 3-11.
Sane, S. P. (2003). The aerodynamics of insect flight. Journal of experimental biology, 206(23), 4191-4208.
Schwentner, M., Combosch, D. J., Nelson, J. P., & Giribet, G. (2017). A phylogenomic solution to the origin of insects by resolving crustacean-hexapod relationships. Current Biology, 27(12), 1818-1824. e1815.
Selden, P. A., Huys, R., Stephenson, M. H., Heward, A. P., & Taylor, P. N. (2010). Crustaceans from bitumen clast in Carboniferous glacial diamictite extend fossil record of copepods. Nature Communications, 1(1), 50. https://doi.org/10.1038/ncomms1049
Shen, X., Marcos, & Fu, H. C. (2020). How the bending mechanics of setae modulate hydrodynamic sensing in copepods. Limnology and Oceanography, 65(4), 749-761.
Soh, H. Y. (2013). Invertebrate Fauna of Korea: Marine Planktonic Copepods II.
Steinberg, D. K., & Landry, M. R. (2017). Zooplankton and the ocean carbon cycle. Annual review of marine science, 9, 413-444.
Strickler, J. R. (1982). Calanoid Copepods, Feeding Currents, and the Role of Gravity. Science, 218(4568), 158-160.
Studying Cells – Cell Size. ((2022, June 8)). https://bio.libretexts.org/@go/page/12711
Vogel, S. (2008). Modes and scaling in aquatic locomotion. Integrative and Comparative Biology, 48(6), 702-712.
Walter, T. C., & Boxshall, G. (2013). World of Copepods Database. . https://www.marinespecies.org/copepoda on 2023-10-02. doi:10.14284/356
Wang, Q., & Wang, Z. (2022). Quantitative Analysis of Drag Force for Task-Specific Micromachine at Low Reynolds Numbers. Micromachines, 13(7), 1134.
What do Copepods Eat? An Overview of the Diverse Diet of These Tiny Aq. (2022). https://www.podyourreef.com/blogs/care/what-do-copepods-eat-an-overview-of-the-diverse-diet-of-these-tiny-aquatic-creatures
Winslow, J., Otsuka, H., Govindarajan, B., & Chopra, I. (2018). Basic understanding of airfoil characteristics at low Reynolds numbers (10 4–10 5). Journal of aircraft, 55(3), 1050-1061.
Yen, J. (2000). Life in transition: balancing inertial and viscous forces by planktonic copepods. The Biological Bulletin, 198(2), 213-224.