Aquatic Fungi: An Exploration of Adaptations in Chemical Processes
Izabela Junqueira Magalhaes, Kristina Kerkelova, Ruizhi Liu, Yasmine Sadr Kaufmann
Abstract
This article explores the chemistry behind the diverse functions and characteristics of aquatic fungi, a fascinating group of microorganisms that inhabit freshwater and marine ecosystems. Aquatic fungi play an important role in the cycling of nutrients and the decomposition of organic matter. This article gives an overview elucidating the key chemical processes and compounds that rule their activities, explores the structural and biochemical aspects of hyphae, and explains the role of exoenzymes in nutrient acquisition and organic matter degradation. The decomposition done by aquatic fungi is discussed, showing their contributions to ecosystem sustainability and the recycling of organic material. Moreover, the environmental implications of mycoremediation are studied, highlighting their potential in plastic cleanup, heavy metal bioremediation, and the biodegradation of organic compounds, ultimately fostering a cleaner environment in an economic and eco-friendly way. This overview also investigates chemotaxis and pheromones in the context of aquatic fungi, offering insights into their navigation mechanisms, and how they can sense certain substances, being attracted to regions with high density of nutrients or potential partners. The potential application of the fungal pheromone sirenin for humans is discussed, along with the concept of quorum sensing, revealing the significance of signaling compounds in fungal communication. This paper covers the intricate chemistry aspects behind the communication and reproduction and physiological structures and processes of aquatic fungi, emphasizing their significant contributions to aquatic ecosystems and environmental bioremediation.
Introduction
Aquatic fungi, a special branch of the fungi kingdom, are eukaryotic microorganisms that have existed for millions of years on Earth. They are closely related to animals and are thought to have diverged from the lineage of animals about 800 million years ago (Organismal Biology). Just like animals, fungi are heterotrophic. They take in organic compounds as a source of carbon and energy, as opposed to plants that conduct photosynthesis directly to produce food. But unlike animals, which first ingest food and then digest it, fungi digest first externally and then ingest the products through osmosis by the cells. This unique and distinct feeding mechanism is called osmotrophy, and its development in many eukaryotic groups is considered convergent evolution (Raghukumar, 2017).
Aquatic fungi play an important role in decomposition. Their ability to decompose the coarse organic matter in streams and water helps other aquatic organisms to gain food and nutrients, and therefore, benefit the whole ecosystem. Aquatic fungi are also used in bioremediation to reduce pollution by breaking down pollutants and plastics, and detoxification by removing metals from the aquatic environment.
Hyphae are one of the most important components of fungi. They are masses of branched filaments that look like thread and have a diameter of 4-6 mm. They support fungi's unique feeding mechanism by growing into the substrates and absorbing nutrients. Each filament is called a hypha, and together, hyphae come to form the mycelium. This network structure is referred to as the vegetative part of the fungi, and sometimes this part can be visible, such as in mushrooms (see Fig. 1) (Biology Dictionary, 2019). Aquatic fungi typically grow with their mycelium on submerged wood or plants and develop symbiotic relationships with aquatic organisms.
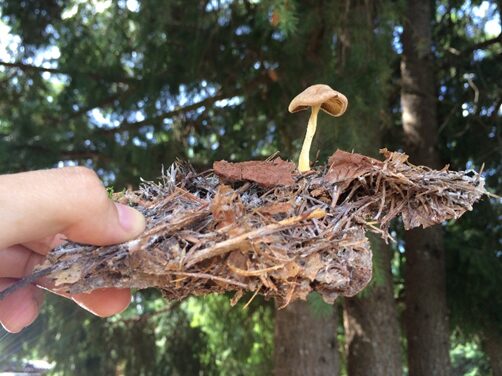
Fig. 1: The mushroom is the top fruit part, and underneath the mycelium grows far and wide to form vast networks (mushroom life cycle, 2015).
Hyphae classification
Hyphae are an important factor in classifying various fungi species. Most fungi have internal walls called septa to divide cells in hyphae, and they are called septate hyphae (see Fig. 2).
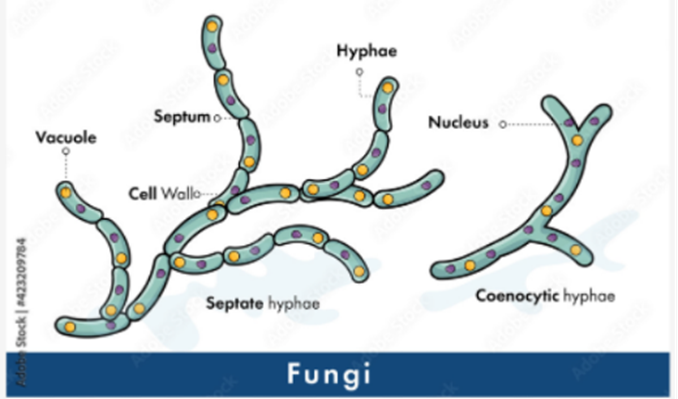
Fig. 2: Classification of fungi based on hyphae. Septate hyphae with septa on the left and coenocytic hyphae without septa on the right (Adobe Stock (n.d).).
Septa allows movement of proteins, ribosomes, and even nuclei between cells by having small pores. Fungi without this partition or septa are called aseptate hyphae or coenocytic hyphae, and they are big cells with multiple nuclei (LibreTexts, 2021). Coenocytic hyphae allow nutrients to be exchanged and move directly to other parts of the cytoplasm. However, this also causes aseptate hyphae to die more easily if the hypha is ruptured, since everything will leak out from the cytoplasm. On the other hand, septate hyphae can close their septa if the hypha is ruptured to prevent any further leakage of substances from the hypha, and thus, increase their chances for survival (Becker, 2018). There is also the term “pseudohyphae” which refers to chains of fungal budding cells that are easily disrupted and grow from the true hyphae. Cells in pseudohyphae are separated by constrictions instead of septa. Some yeast species produce these pseudohyphae (see Fig. 3) (Lakna, 2018).
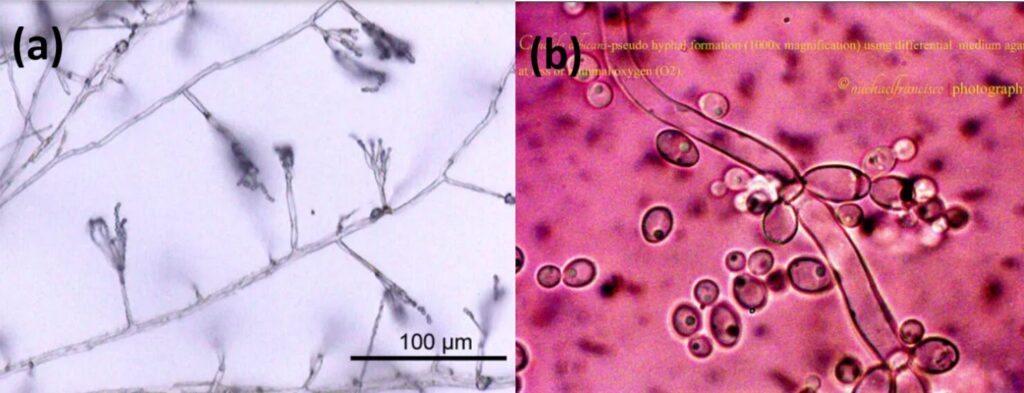
Fig. 3: True hyphae of Penicillium (a) and Pseudohyphae of Candida albicans (b) (Lakna, 2018).
Furthermore, hyphae that have a thick cell wall and are highly branched are called binding hyphae; hyphae with a thin cell wall and less branched, but with many septa, are called generative hyphae; and hyphae with a thick cell wall but few septa are called skeletal hyphae (see Fig. 4).
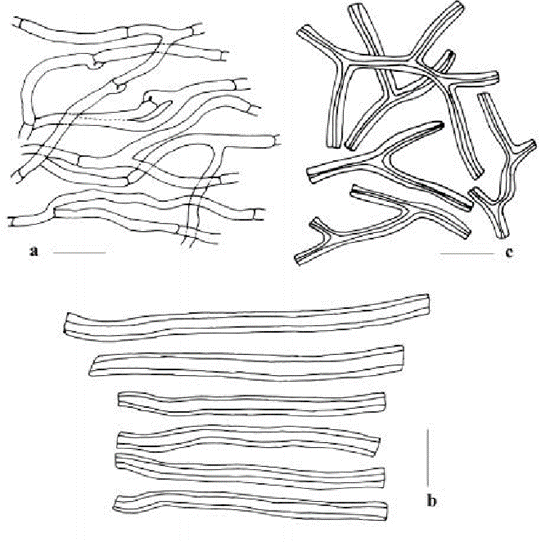
Fig. 4: a is the generative hyphae, b is the skeletal hyphae and c is the binding hyphae. (Tarafder et al., 2017)
Individual cell wall
The most regulated and diverse part of a fungus is its cell wall. Around one-sixth of the fungal genome is needed in order to build the cell wall. That is more than 1,000 genes and proteins, and other networks of signaling systems are also involved. Each fungal cell wall may have a unique chemical composition because there is no precise genetic template for the synthesis of the fungal cell wall's major components, and there are random processes involved in adding key cell wall components. Fungi commonly have an inner cell wall composed of chitin and β-glucans which act as a flexible frame and an outer cell wall where glycosylated proteins and polymers are attached. These adhesive proteins share a tertiary structure with Ser-Thr amino sequences at one end and a ligand-binding T-domain (the domain where other proteins can bind to) at the other end (see Fig. 5). They are able to sort themselves into a more ordered state through β-aggregation (Gow & Lenardon, 2023).
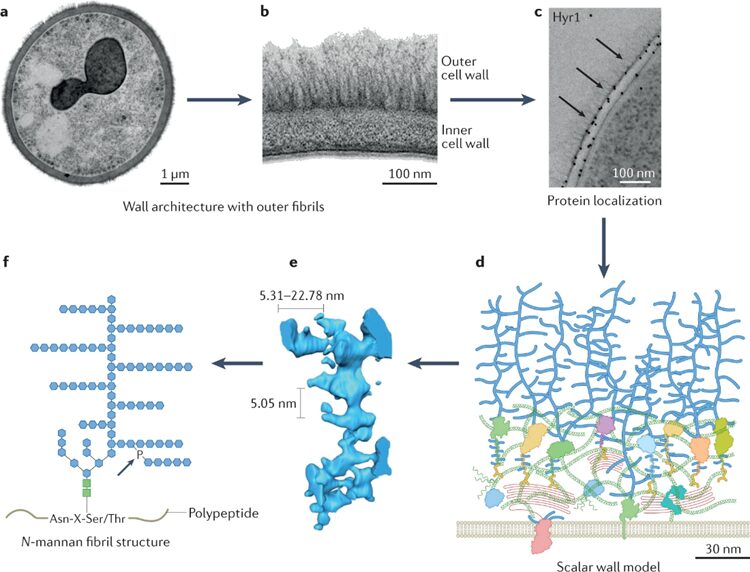
Fig. 5: a, b, and c show that the adhesive proteins are on the outer cell wall. d, e, and f are computer modelling graphs showing the structure of outer proteins. f illustrates that they share common amino acid sequence at one end of tertiary proteins (Gow & Lenardon, 2023).
Furthermore, the composition of the cell wall is also constantly modified based on the current environment. Cell walls are especially important for aquatic fungi since they prevent the outburst of cells from the osmotic pressure, as the water is driven into the cell in a diluted environment. While in a more concentrated environment, fungi are able to prevent water loss by producing a special protein called hydrophobin. Hydrophobins are insoluble and form a hydrophobic layer to seal the cell wall. Fungi also have proteins called aquaporins which regulate the water flow across the cell to keep them in shape for survival. Moreover, some fungi generate an extracellular polysaccharide capsule to protect them from predators and to avoid detection from the immune system of other species (Gow & Lenardon, 2023).
Septate hyphae growth
Hyphae grow at the tip of each hypha by extending cells with the help of a specialized organelle, a spitzenkörper, which is the German name, meaning “pointed body” (Biology Dictionary, 2019). The extension is also regulated by pectin and Ca2+ concentration. In the cell wall, Ca2+ is cross-linked with pectin, a carbohydrate polymer that is in the cell wall of fungi, to strengthen the cell wall. During the cell expansion, the new pectin competes for Ca2+ with the old ones, causing the cell wall to loosen. And by doing so, pectin aids in the continuous formation of cells and extension of the hyphae. Then there is an increase in Ca2+ concentration during the growth of hyphae, which helps in vesicle fusion in cell wall synthesis. Despite the varying mechanisms of creating this Ca2+ gradient, in all the fungi cases studied, it involves a pathway detecting changes in the cell wall tension to regulate Ca2+ concentration at various locations by signal transduction. For example, in the hyphae of oomycete S. ferax, the Ca2+ is controlled by the stretch-activated Ca2+ channels which are located at the tip of the hypha, and through which Ca2+ is transported into the cell. While in the hyphae of N. crassa, the Ca2+ is controlled and maintained internally by InsP3 (inositol-1,4,5-trisphosphate, which is produced by a stretch-activated phospholipase C), which helps release Ca2+ from stores at the tip. In both ways, the increase in Ca2+ concentration is said to aid in the vesicle fusion process in the expansion of cells, even though the mechanism of this is not fully understood (Lew, 2011). However, the mechanism of transportation of vesicles is understood and it is mainly carried out by spitzenkörper in the cell. Spitzenkörper picks vesicles released from the Golgi apparatus and drops them at the vertex of the hypha. (see Fig. 6) These extracellular vesicles contain biosynthetic and remodeling enzymes for the cell wall and are shown to play a role in repairing damaged cell walls. The cell wall at the tip of the hyphae is then built and extended through the content released by the vesicles, and the vesicle membranes fuse to create a new cell membrane (Biology Dictionary, 2018). Chitin and glucan synthases, which catalyze the polysaccharides that make up the cell wall, are delivered and activated to allow assembly of polysaccharides at the cytoplasmic side of the cell membrane. Fibrils also start cross-linking with other wall components (Gow & Lenardon, 2023). As hyphae extend, the septa are also formed to separate each cell. Thus, the hyphae growth is accomplished by the regulation of pectin, which first loosens the cell wall, and then the organelle spitzenkörper helps transport vesicles to the tip of the hypha to form the cell wall and membrane, and the increase assists the vesicle fusion in Ca2+ gradient.
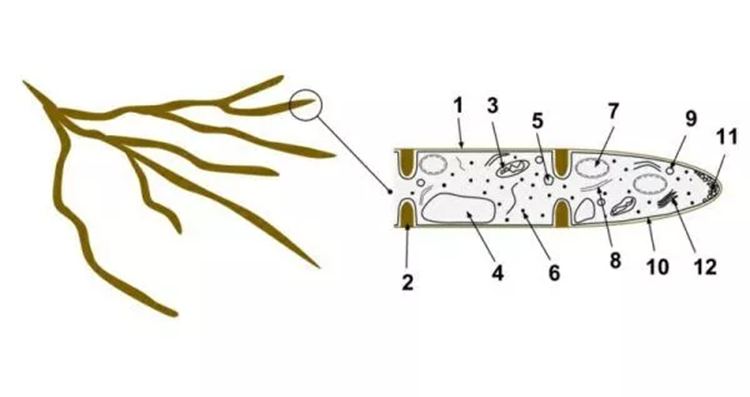
Fig. 6: 1- hyphae wall, 2- septum, 3- Mitochondrion 4- Vacuole 5- Ergosterol crystal 6- Ribosome 7- Nucleus 8- Endoplasmic reticulum 9- Lipid body 10- Plasma membrane. Number 11 is the spitzenkörper and vesicles at the apex of the hypha, and number 2 is the septum separating the cells (Biology Dictionary, 2018).
Use of chemotaxis by aquatic fungi
Fungal spores use chemotaxis to adhere to substrates in areas with nutrients that will be beneficial for their growth. Chemotaxis is the movement and attraction or repulsion of organisms towards certain chemicals. Attraction is called positive chemotaxis, while repulsion is called negative chemotaxis. It is presently unknown the exact process that aquatic fungi use to detect and move toward these chemicals. It is assumed that the chemicals bind to a receptor protein, which then leads to a reaction in the cell, but details are unknown (Moss et al., 2008).
In the marine fungus Rhizophydium littoreum, there is recorded chemotaxis towards carbohydrates, like potato starch and amylase, nitrogen-rich compounds, like bovine serum albumin, and polysaccharides. In a study performed with isolated Rhizophydium littoreum spores,the strongest chemotaxis was to polysaccharides, potentially implying that they are the nutrient that fungi prioritise and need the most. It is also possible that evolutionarily, places with polysaccharides made better substrates. Since fungal spores both swim in the water and are moved by it, it is advantageous for the spores to know where there is a good food source, as it allows fungi to locate high-nutrient environments where they will be able to proliferate more easily (Muehlstein et al., 1988).
Chemotaxis was also observed in many chytrids, like Batrachochytrium dendrobatidis and Blastocladiella emersonii. Batrachochytrium dendrobatidis (Fig. 7 and Fig. 8), like Rhizophydium littoreum, was found to display positive chemotaxis to sugars and proteins when tested in a lab setting. This chytrid is parasitic and infects and feeds off of amphibians, so the chemicals it responds to could be connected to what amphibians emit. If the chemicals are specific to amphibians, they would inform the fungi of where their specific ideal substrates are located. However, this is still merely a theory (Moss et al., 2008).
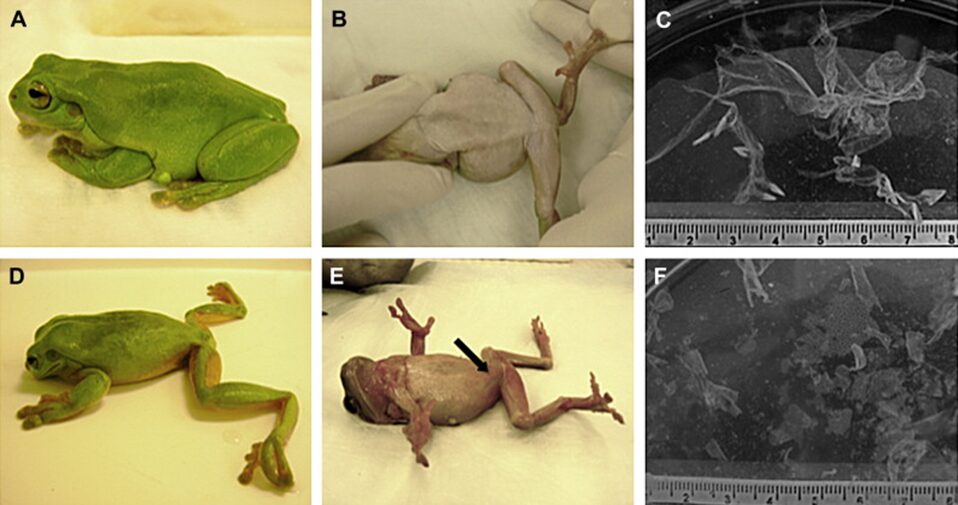
Fig. 7: Appearance and behavior of Litoria caerulea and the pattern of skin shedding were altered in animals experimentally infected with Batrachochytrium dendrobatidis. A, B) Lateral and ventral views of a healthy frog. C) A single large skin slough from a healthy frog. D) A frog with severe chytridiomycosis showing abnormal posture with head lowered and hind legs abducted. E) A frog in dorsal recumbency that is unable to reorient and has cutaneous erythema (arrow) in the ventral epidermis. F) Multiple small skin sloughs collected from the water in the container of a frog with severe chytridiomycosis (Voyles et al., 2011).
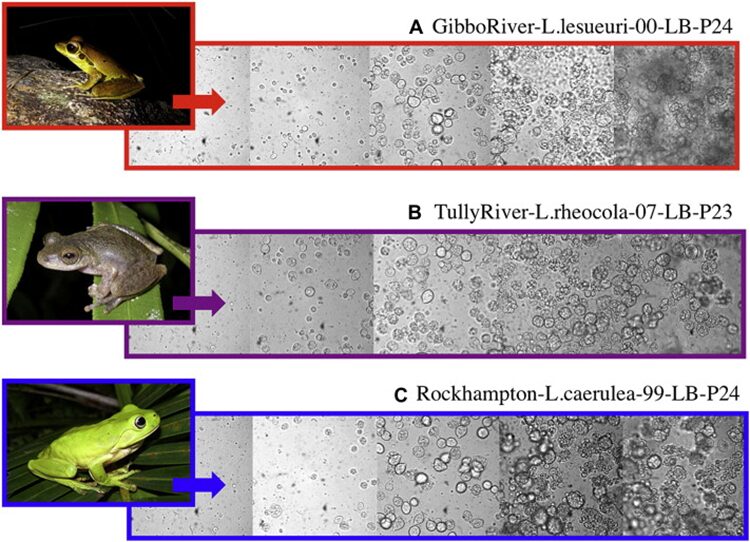
Fig. 8: Light microscopy images of three isolates of Batrachochytrium dendrobatidis: (A) GibboRiver-L.lesueuri-00-LB-P24, (B) TullyRiver-L.rheocola-07-LB-P23, (C) Rockhampton-L.caerulea-99-LB-P24. Images show successive developmental stages of Bd from active zoospores (far left) to mature sporangia (far right). The images of frogs (left side) are representative images of the frog species from which each isolate was originally cultured (Voyles, 2011).
Chemotaxis is a design solution permitting fungi to thrive and find food and substrates in large bodies of water. The ability to detect different molecules, including lipids, proteins, and sugars, and swim towards or away from them, leads to fungal spores swimming directionally, as opposed to in random patterns. Furthermore, this improved detection of what is in their environment allows spores to find nutrients and substrates significantly more easily, hence, increasing proliferation and reproduction.
Use of pheromones in sexual reproduction
Chytridiomycetes are a type of fungi that produce chemoattractants that are involved in fungal development preceding sexual reproduction and in spore attraction. Allomyces macrogynus, a species of aquatic fungi and a member of the class Chytridiomycetes, produces male and female spores on a single thallus. It utilises chemoattractants or pheromones to ensure that male and female gametes meet and reproduce. Since it is an aquatic fungus, the spores have one flagellum each, and both swim and are carried by currents. The female spores produce a compound called sirenin, which they excrete (Moore, 1998). Female gametes produce sirenin in the last few stages of gametogenesis and once they are ejected from the thallus. This production of sirenin has been documented as continuing for at least 6 hours (Muehlstein et al., 1988). Sirenin is a bicyclic sesquiterpene diol with molecular formula C15H24O2 (Fig. 9) and it is active at concentrations of 10-10 M. It has been determined through lab tests that sirenin requires a terminal hydroxymethyl group on the side chain and a hydrophobic group on the other end of the molecule in order for it to function and induce a reaction in male gametes. Sirenin likely binds to a receptor on male spores. However, the structure and characteristics of that receptor are not known (Pommerville et al., 1990).
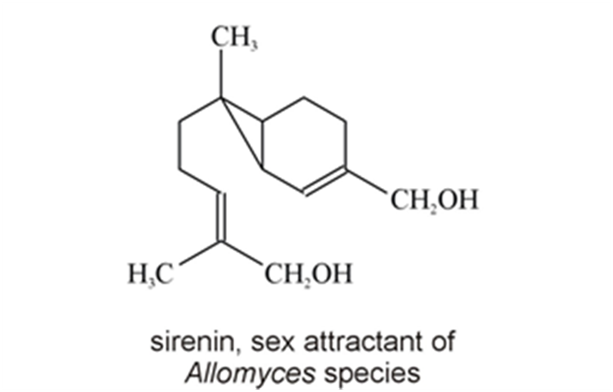
Fig. 9: Chemical structure of sirenin, a pheromone produced by Allomyces (Moore, 1998).
Sirenin affects the movements of male spores. In Allomyces, female spores move less than male spores and male spores move in one direction for a while before stopping and then continuing. Sirenin encourages male spores swimming toward female spores to swim for longer periods, making the male spores stop less, and discourages male spores from swimming away from female spores by causing them to stop more often, thus discouraging the spores from swimming in the opposite direction. They also encourage the male spores to change direction and swim in the direction of the female spores (Moore, 1998). Sirenin makes male gametes have a sudden uptake of calcium ions, with a charge of +2, which enter through calcium cation channels, called CatSper, into the spore's membrane (Syeda et al., 2016). The male gametes, on the other hand, produce a far less studied pheromone called parisin. Parisin leads to the attraction of female spores to male ones and displays characteristics similar to sirenin (Pommerville & Olson, 1987). The exact chemical structure of parisin is not known, nor is the exact interaction that sirenin has with male spores, other than the fact that it involves calcium signalling (Medina & Buchler, 2020). Sirenin is inactivated by male gametes. However, the exact mechanism by which inactivation occurs is unknown (Moore, 1998). The production of sirenin and the fact that it causes an uptake of calcium in male spores is a design solution meant to increase the success of sexual reproduction in Allomyces, as spores swimming in random directions are far less likely to meet.
A potential application of sirenin:
A use for sirenin in medicine, fertility, or contraception could be possible. Sirenin is not only able to activate the calcium ion channels in male fungal spores but also in human sperm. The specific channel complex in human sperm is also called CatSper, as it is in Allomyces macrogynus. It is hypothesized that because of this connection, analogues of sirenin could be synthesized that would instead block the CatSper ion channels in sperm, thus providing a type of contraception (Syeda et al., 2016). CatSper channels in sperm allow the entry of calcium ions into the cells. These ions are required for the hyperactivity of sperm flagellum and chemotaxis to the egg. Their inactivation would impede sperms' ability to fertilize an egg, creating a contraceptive (Sun et al., 2017).
Sexual reproduction hormones in Achlya Ambisexualis
In fungi of the genus Achlya, reproduction is guided by two steroid hormones. The hormone produced by the female gametes as they are being formed is Antheridiol. Antheridiol naturally has a 22S 23R configuration and a molecular formula of C29H42O5 (Fig. 10). It leads to the differentiation and production of male gametes, meaning that it is the female cells that initiate sexual reproduction (Riehl et al., 1984). The male cells secrete oogoniol, in response to the initial secretion of antheridiol by the female cells, leading to the female cells secreting more antheridiol. The combination of oogoniol and antheridiol causes both the female and male cells to grow hyphae towards each other, culminating in conjugation and sexual reproduction. Oogoniol has a similar molecular formula and structure to antheridiol, with C29H48O5 (Fig. 11) (Cole et al., 2003).
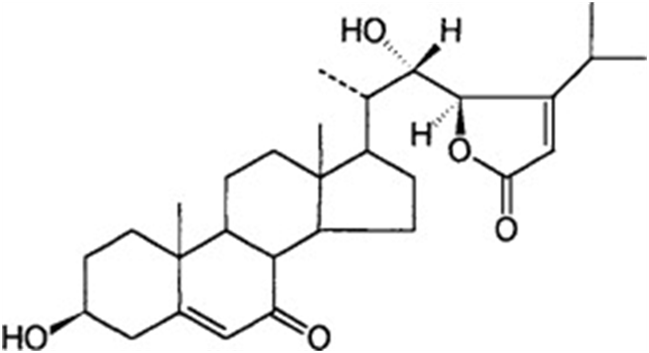
Fig. 10: Antheridiol, a hormone produced by female Achlya cells: 22S, 23R configuration (Moore, 1998).
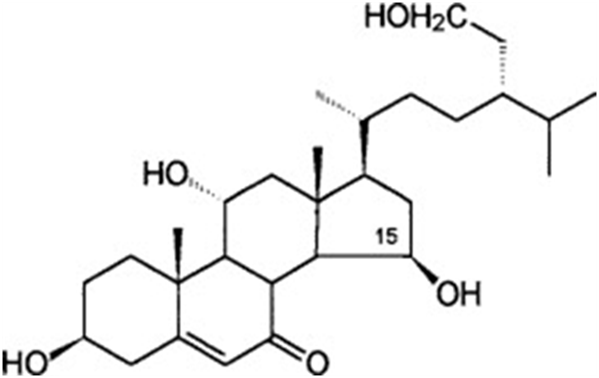
Fig. 11: Oogoniol, a hormone produced by male Achlya cells (Moore, 1998).
Quorum sensing in aquatic fungi
Quorum sensing is the ability of an organism, through signalling compounds, to determine the density or concentration of similar organisms around it. It is an ability that many bacteria are widely known to possess. More recently, it was discovered that certain fungi are also capable of quorum sensing and that they may be using it to communicate amongst themselves. Quorum sensing involves the use of autoinducers, low molecular weight signalling compounds produced by the organism. The concentration of autoinducers in an area is indicative of the density of the organisms and is information that fungi and bacteria can interpret. When a threshold concentration of autoinducers is reached, certain genes are expressed or repressed, indicating that autoinducers affect gene expression and control. Quorum sensing allows multiple cells to behave as one, for example, mass bioluminescence in bacteria once it has passed a certain threshold concentration (Padder et al., 2018).
Of the fungi known to perform quorum sensing, Candida albicans utilises it for control of filamentation. Candida albicans is a pathogenic aquatic fungus often present in the human gut and in the wild (Blaschke-Hellmessen, 1999). It utilises the chemical farnesol, 3,7,11-trimethyl-2,6,10-dodecatriene-1-ol, with molecular formula C15H26O, (Fig. 12), to inhibit the formation of hyphae. Farnesol is also found to affect genes involved in drug resistance within Candida albicans. The oxidized form of farnesol called farnesoic acid produces a milder reaction and is a less effective inhibitor of hyphae formation. Other examples of quorum signalling molecules are tryptophol (Fig. 13) and 1-Phenyl-ethanol (Fig. 14), auto-antibiotics that also inhibit the formation of filaments and hyphae in C. albicans (Padder et al., 2018).
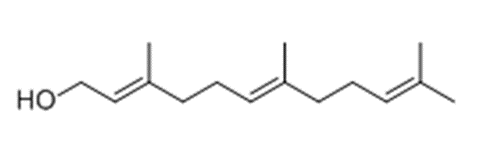
Fig. 12: Farnesol, quorum signalling molecule in Candida albicans (Padder et al., 2018).
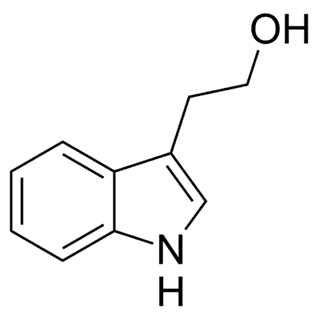
Fig. 13: Chemical structure of Tryptophol (Padder et al., 2018).
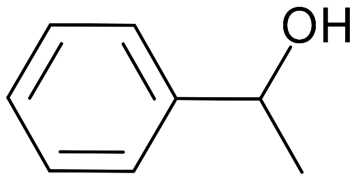
Fig. 14: Chemical structure of 1-Phenyl-ethanol(Padder et al., 2018).
Inhibition of quorum sensing by fungi
A study ascertained that certain fungal molecules' secondary metabolites were able to prevent quorum sensing in bacteria. Of the 75 molecules tested, four showed great inhibition of quorum sensing. Of those four, two molecules came from fungi that grow on corals. These molecules were from Sarocladium (LAEE06), Fusarium (LAEE13), Epicoccum (LAEE14), and Khuskia (LAEE21) and each was assigned an identifier, as shown in brackets. In concentrations varying from 500 to 50 µg/ml, molecules from these fungi caused significant disruption to quorum sensing in bacteria. This disruption was attributed to secondary metabolites, some of which were isolated and are depicted in Fig. 15 (Martín-Rodríguez et al., 2014). Therefore, inhibiting quorum sensing is something that fungi have evolved to do, while simultaneously using it as well.
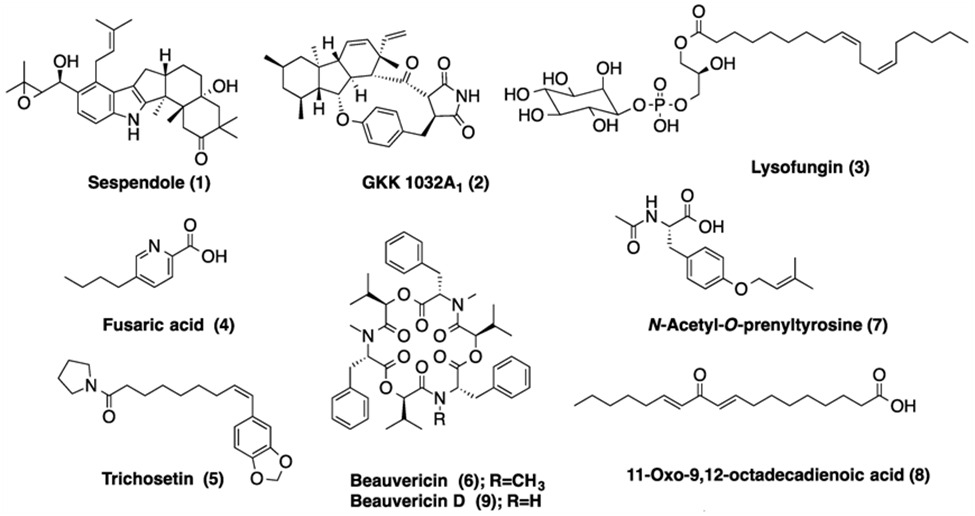
Fig. 15: Chemical structures of some secondary metabolites detected in bioactive extracts (Martín-Rodríguez et al., 2014).
Decomposition by aquatic fungi
Aquatic fungi play a crucial role in the decomposition of plant litter in smaller streams that flow into or out of rivers. When coarse particulate organic matter (CPOM), which refers to particles >1mm in size, originating from leaves or twigs, enters streams, fungi work to colonize and degrade it so they can gain nutrients and energy from it. This allows fungi to increase their biomass and sporulate, and it also breaks down plant matter to make it palatable for other aquatic invertebrates called shredders, that do not have the required enzymes to break down CPOM. By doing this, aquatic fungi transfer energy to other trophic levels in the food web (Seena et al., 2022).
Aquatic fungi are considered significant decomposers if they (1) colonize CPOM faster than other competing microorganisms such as bacteria, (2) produce enough enzymes to be able to break down substantial amounts of CPOM, and (3) create a net loss of CPOM biomass. CPOM decomposition results in the formation of carbon dioxide, other mineral substances, dissolved organic matter, and fine particulate organic matter. Importantly, it results in an increase in fungal biomass. Aquatic hyphomycetes' (AQHs) ability to degrade CPOM is amongst the most studied, as they are the main fungal decomposers of CPOM in aquatic ecosystems (Gessner et al., 2007).
Decomposition process
The decomposition process has three main steps after CPOM enters water: attachment and colonization, mycelial growth inside and on the leaf, and breakdown of leaf structural polymers (Gessner et al., 2007).
Fungi attach to CPOM in three ways: by outgrowth of hyphae from one leaf/twig touching another, by detached hyphal fragments making contact with a new leaf/twig, or by asexual AQH spores (conidia) landing on the surface of the leaf/twig. Colonization by conidia is the most common. Just hours after the fungi have reached the CPOM, they develop specialized adhesion structures called appressoria which pierce the top of the leaf, guaranteeing adhesion. Next, rapid hyphal extension occurs, and conidia are produced and released. Around 7000 conidia are produced per day per mg of dry leaf mass (Dang et al., 2007).
Aquatic hyphomycetes conidia attachment
The shape of AQH conidia affects their ability to attach to CPOM and thus, decompose it. AQH conidia vary in structure, with three categories: tetraradiate (branched), filiform (thread-like), and compact (seed-like) (Fig. 16). Of the three, tetraradiate conidia are most easily trapped on the surface of CPOM, as they make contact with three of their four branches. Filiform conidia are often twisted, allowing them to make contact with the CPOM at two points. Compact conidia can only have one point of contact with the CPOM; they are the least efficient at attaching. The more points of contact, the more surface area at which chemical reactions can occur between the CPOM and fungi. While compact conidia do not have the opportunity to attach at multiple points, their small size allows many conidia to attach to one leaf or twig, creating more points of contact and thus increasing the surface area over which reactions can happen. Aquatic fungi have optimized their attachment techniques for all spore types to succeed to some degree and gain energy and nutrients from leaves. Additionally, leaf topology impacts attachment. The top, smoother parts of leaves typically have less fungal attachment than the rough, underside. The veins and tufts of leaf undersides provide good anchorage for spores (Fig. 17), (Dang et al., 2007).
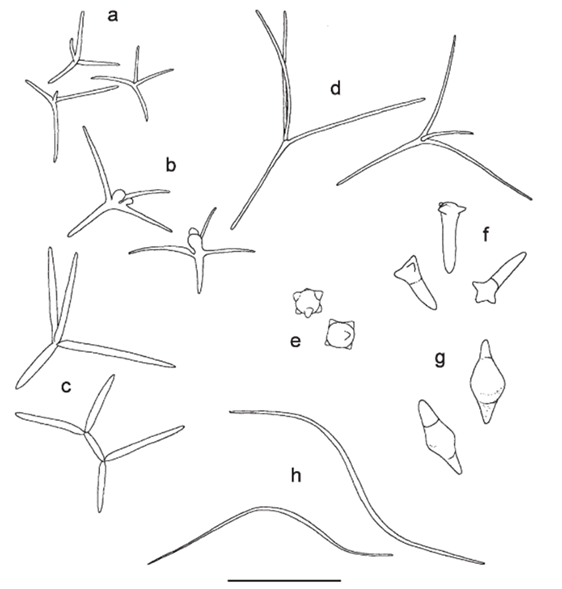
Fig. 16: Various AQH spores. (a-d) Tetraradiate, (e,f,g) compact, (h) filiform. Scale bar: 50 microns (Dang et al., 2007).
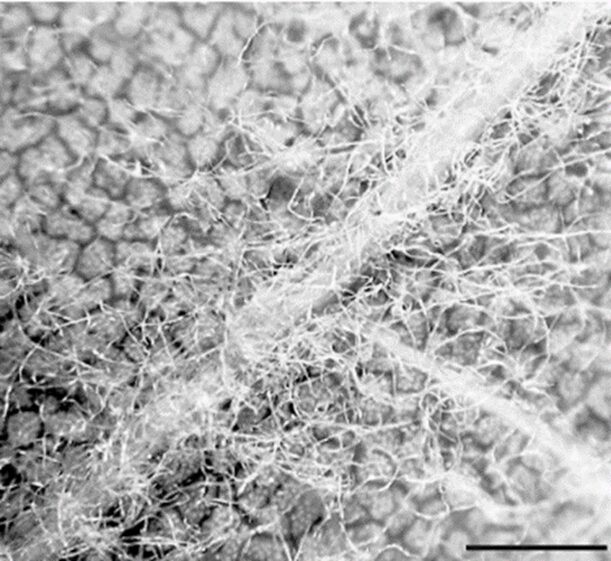
Fig. 17: Lower side of oak leaf colonized by AQH conidia. Higher concentration of conidia at tuft. Scale bar = 1mm (Dang et al., 2007).
Infiltration into the leaf matrix
Following attachment, fungi infiltrate the leaf matrix using their appressoria (Fig.18). This allows them to get closer to the structural elements of the leaf and begin the breakdown process. Mycelial growth occurs rapidly, and spore-holding structures are created, which release spores (conidia) in as little as six days post-colonization (Dang et al., 2007). As hyphae grow, fungi surface area increases, creating a larger interface at which enzymes can exit the hyphae and break down the CPOM's structural polymers (Gessner et al., 2007).
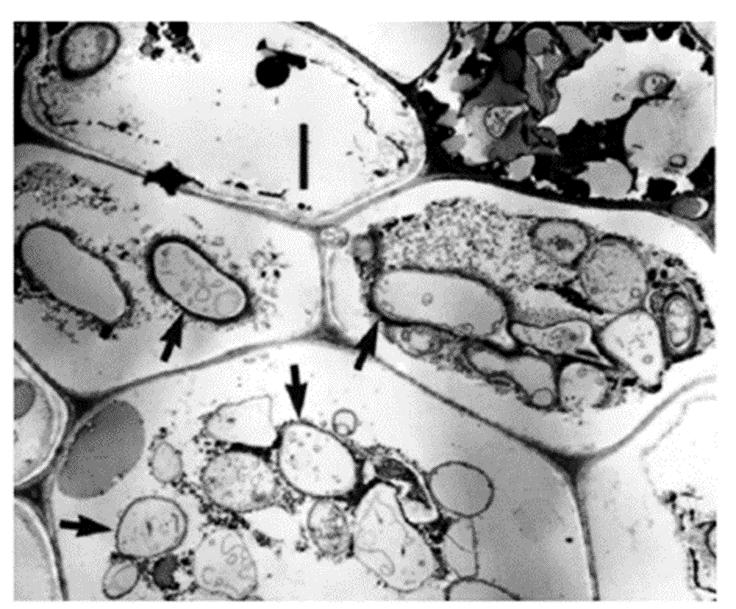
Fig. 18: Transmission electron micrograph of yellow poplar leaf cross-section. Four hyphae inside the cellular matric are indicated by black arrows. Scale bar = 2 microns (Dang et al., 2007).
Exoenzymes
As previously mentioned, fungi have a unique feeding mechanism in which they first digest outside the body and then ingest and extract nutrients from their hyphae. Enzymes that are secreted outside of the fungal body to perform their function are called exoenzymes. The secretions of enzymes to digest the food are very important for fungi to survive. Studies have shown that fungi can control their gene expressions to produce particular enzymes based on their habitats and environmental stimuli (Archer & Wood, 1995).
Enzymes specific to CPOM decomposition
AQHs have enzymes that can break down the three main plant fibers that keep leaves and twigs intact: pectin, cellulose, and hemicellulose. Enzymes that hydrolyze cellulose (endoglucanase, exoglucanase, and exoglucosidase) and hemicellulose (xylanases, xylosidase, and arabinosidase) are released by hyphae. Most importantly, the degradation of pectin by pectinlyase and polygalacturonase finally macerates the plant tissues and releases mesophyll cells (internal cells coming from between the epidermal cells of the leaf) from the plant. When this process is complete, the plant matter can be digested by shredders.
AQHs' ability to degrade lignin, another plant fiber, is not well understood. Some researchers believe that AQHs do not possess the required enzymes for lignin breakdown (CHAMIER, 1985). Much more recently, Heeger et al. (2021) sequenced AQHs' genome and identified that various laccases, peroxidases, and putative cytochrome P450 monooxygenases can be created by this organism. This suggests that AQHs can modify lignin to some extent, maybe converting the polysaccharide component of lignocellulose into carbon and energy sources (Heeger et al., 2021).
Factors impacting decomposition rate
After colonization of the CPOM, fungal biomass increases slowly for a few weeks, then levels off or decreases slightly (Fig. 19 (a)). The rate of fungal growth depends on external factors, such as temperature and water composition, as well as internal factors, such as the quality of the CPOM. Average fungal biomass levels remain constant, but production peaks in warmer temperatures, summertime in particular. Fungal biomass per area of CPOM peaks in autumn, after trees shed their leaves and there is significantly more CPOM in streams. Additionally, there is a strong positive correlation between dissolved nitrogen and phosphorus in streams and fungal sporulation and biomass. The rate of decomposition is higher when more nitrogen and phosphorus are present. Furthermore, fungal growth is favored when the fungi have a higher C:N or C:P ratio compared to the CPOM (Stelzer et al., 2003). Less fungal growth on alder leaves low in nitrogen and phosphorus confirms this (Nikolcheva et al., 2003) (see Fig. 19(b)).
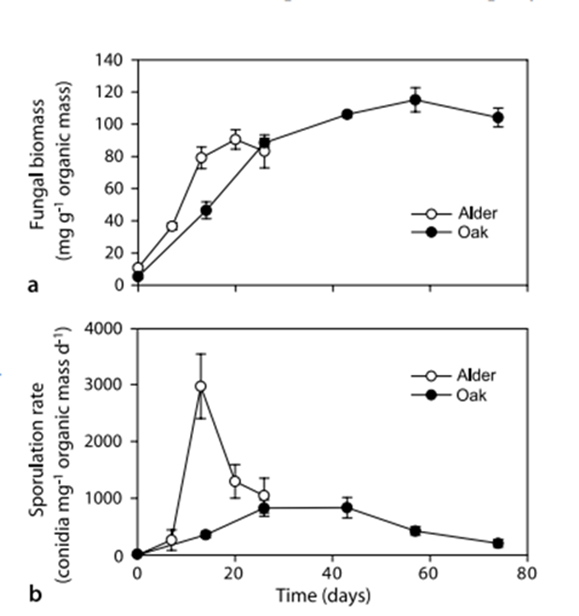
Fig. 19: Fungal biomass (a) and sporulation rates (b) of aquatic hyphomycetes (AQHs) associated with alder and oak leaves decomposing in Portuguese stream (Ferreira & Chauvet, 2011).
Bioremediation: aquatic fungi as a solution to modern-society problems
Bioremediation is the process of using living organisms to clean up and remove environmental contaminants. This technique has been shown to be a great replacement for conventional clean-up techniques using machines and chemicals due to its high effectiveness, economic feasibility, and eco-friendliness (Singh et al., 2020). This technology addresses the issue of pollution or contamination in the ecosystem by harnessing natural microbial processes that transform toxic substances into harmless ones (Sharma & Adholeya, 2011).
In the case of aquatic systems, pollution is an ongoing and severe problem that has many negative impacts and demands immediate addressing. The contamination and unbalance of water bodies and marine environments represent a matter of public health, a threat to various aquatic species, and an economic issue (Given et al., 2006). One way to confront this situation is called mycoremediation: the employment of different species of aquatic fungi for the clean-up and detoxification of aquatic ecosystems affected by different toxic substances or compounds that resist removal by natural processes or conventional treatments (AbuQamar et al., 2023).
Because of the different metabolic reactions they are capable of, as well as their ability to adapt, aquatic fungi are a powerful tool for bioremediation, representing a sustainable and clever solution to the pollution of aquatic environments. Mycoremediation relies on the action of species of aquatic fungi on their own or in microbial consortia (AbuQamar et al., 2023) to sequester or degrade contaminants through the digestive enzymes they produce (Hawksworth & Lücking, 2017). In the case of microbial consortia, aquatic fungi interact and coexist with other species, also capable of chemical processes, resulting in the control of harmful substances to the environment, promoting bioremediation (Kumar et al., 2023; Reis et al., 2018). This combination of organisms includes aquatic fungi, bacteria, and other microorganisms and can be engineered and designed for many applications, such as degrading pollutants and breaking down complex organic matter (Padmaperuma et al., 2020).
Among the pollution issues that can be addressed by mycoremediation is the contamination of marine and freshwater environments through the introduction of chemicals, plastics, oil, waste from industries and agriculture, especially fertilizers (nutrient pollution), and residential trash (AbuQamar et al., 2023; Frid & Caswell, 2017). This approach offers several advantages due to fungi's ability to effectively break down a diverse range of contaminants while not being affected by the concentration of these pollutants, making it a highly desirable remediation method (Vaksmaa et al., 2023). See an overview of this technology in Figure 20.
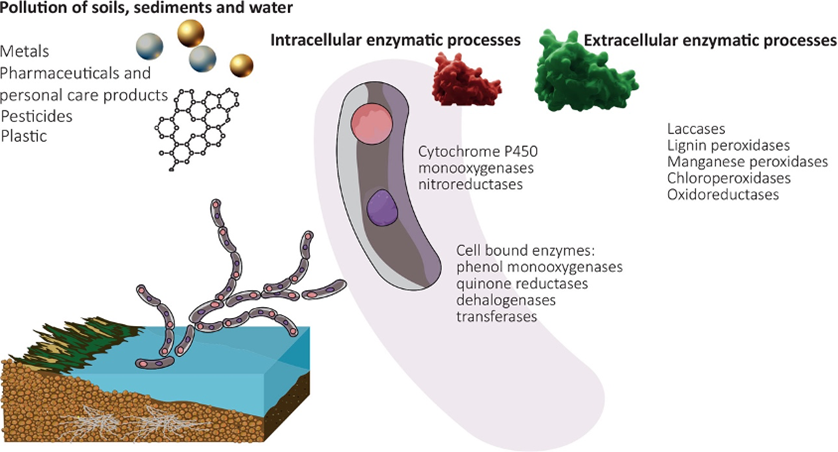
Fig. 20: Schematic overview of mycoremediation in aquatic environments highlighting pollutants and common fungal enzyme classes (Vaksmaa et al., 2023).
Additionally, the use of mycoremediation in cleaning up aquatic environments presents multiple economic benefits. It is an energy-efficient process with long-lasting practicality, resulting in minimal secondary pollution and eliminating the need for additional construction (Gao et al., 2018). These combined advantages make mycoremediation and microbial technology attractive solutions for addressing pollution in aquatic ecosystems.
The most important chemical mechanisms currently being used for mycoremediation are the processes done by fungi to break down organic pollutants, clean up plastics, and remove metals from aquatic ecosystems such as those summarized in Fig. 21, to be studied in the following sessions.
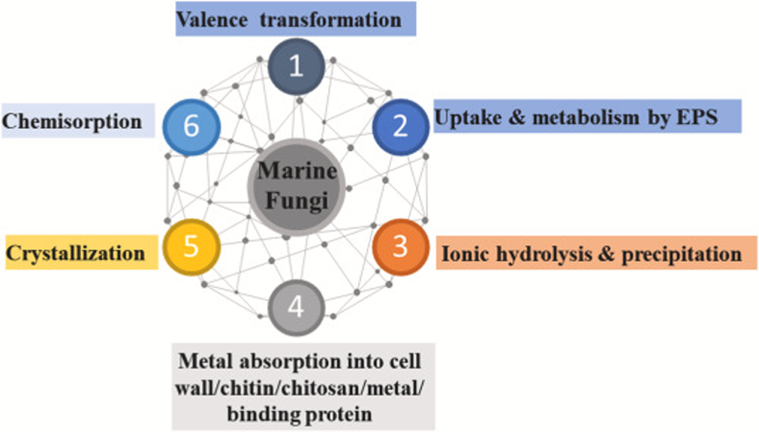
Fig. 21: Chemical processes associated with mycoremediation exemplifying marine fungi (AbuQamar et al., 2023).
Metal mycoremediation
The presence of heavy metals in aquatic systems poses a significant threat to both flora and fauna, as well as to human health. Heavy metals, such as silver, copper, lead, mercury, cadmium, and arsenic, can enter water bodies through various natural and anthropological sources, including industrial discharges, mining, electronic waste, and runoff from urban areas (Gautam et al., 2014). These toxic substances can accumulate in aquatic organisms in a process called bioaccumulation or biomagnification (see Fig. 22), leading to serious consequences. For aquatic flora and fauna, heavy metal exposure can result in reduced growth, impaired reproduction, and, in severe cases, fatalities (Chakraborty, 2023). Additionally, when humans consume contaminated fish or water, heavy metal exposure can lead to various health problems, including neurological disorders, organ damage, cancer, or even death (Mitra et al., 2022).
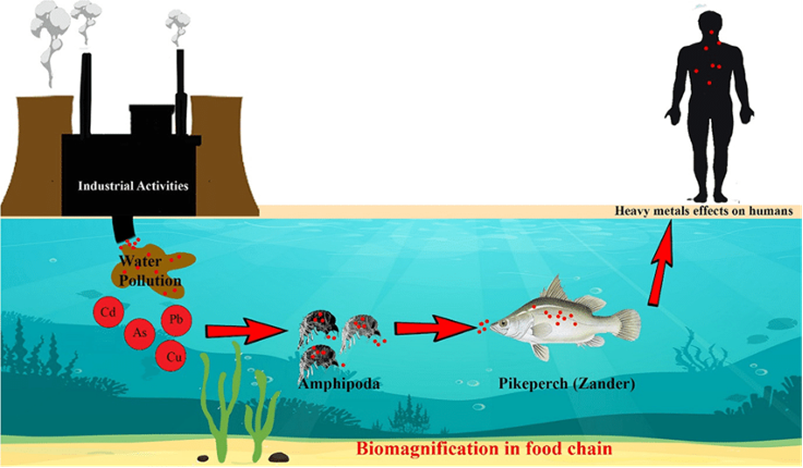
Fig. 22: A schematic model of bioaccumulation of heavy metals in aquatic food chain (Dehghani et al., 2022).
There are several methods to remove these harmful metals from the environment, but bioremediation with fungi is shown to be one of the most effective and eco-friendly ways. The transformation of heavy metals in mycoremediation is often done by biosorption, bioaccumulation, and biovolatilization (Boriová et al., 2014; Matozzo et al., 2018). Biosorption is a passive process that can be carried out by either living cells or dead biomass, and it operates independently of metabolic activity (Matozzo et al., 2018). In contrast, bioaccumulation is a process that occurs within living cells, relying on their metabolic functions. Biovolatilization entails the transformation of both inorganic and organic compounds into their volatile derivatives through intracellular enzymatic reactions (Akhtar & Mannan, 2020).
One incredible feature of aquatic fungi that makes mycoremediation so suitable for the management and removal of heavy metals is that they are not only resistant to the presence of these toxic substances, but also adapt well to the conditions of polluted water. For example, some species show an increase in the enzymatic activity of antioxidant enzymes as a response to the accumulation of heavy metals, helping them tolerate high concentrations of many different metals (Vaseem et al., 2017). This is the case of fungi that are present in areas close to abandoned mining sites, one of the main sources of metals discharge into nature, that adapt to tolerate and accumulate high levels of silver nitrate and gold, accumulating it (Cecchi et al., 2017). In cases like this, fungi represent the hope of improving health and restoring living conditions to other organisms in these areas. Another possibility studied in the mycoremediation of mining areas that makes it even more economically favorable is the possibility of recovery of these valuable metals for the fungi's mycelium (Cecchi et al., 2017).
Furthermore, the presence of surfactants can also help fungi in the removal of metals. Because surfactants are amphiphilic chemical compounds that have the ability to reduce the surface tension of a liquid, they aid aquatic fungi in the removal of, for example, manganese from water by increasing the surface area and metal-binding sites on fungal hypha (Wu et al., 2016). As a result, the bioaccumulation of this metal by fungi is enhanced, leading to its complete removal (Akhtar & Mannan, 2020). Additionally, surfactants are easily and naturally degraded and present low toxicity to the environment, besides being diverse and accessible, fitting into the advantages of mycoremediation (Matozzo et al., 2018).
Many studies have researched the metal harvesting abilities within the enormous diversity of aquatic fungi species. Although more study is always needed in the field due to the emergence and gravity of heavy metal pollution in marine and freshwater environments, is possible to compile some of the most known species for the bioremediation of specific metals, as seen in Table 1:
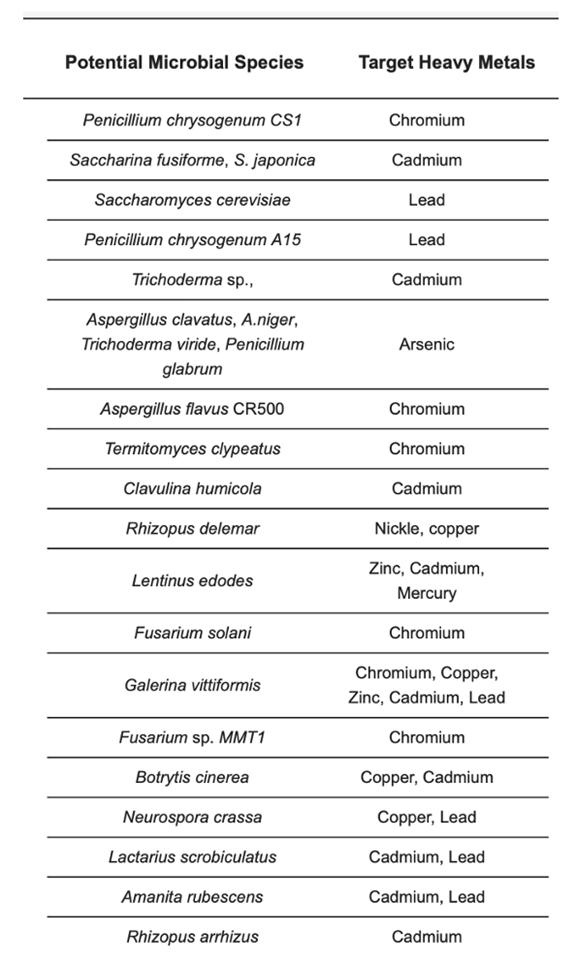
Table 1: Mycoremediation of heavy metal-contaminated matrixes (Najeebul et al., 2022).
Plastic clean-up
Another issue that arises from anthropological activities is plastic pollution. The accumulation of plastic waste in the marine environment has been ongoing since the commencement of large-scale plastic production in the 1950s (Zeghal et al., 2021). Plastic pollution includes single-use plastics such as bottles, personal care products and other packaging materials, microplastics, and nanoplastics, and it is one of the main environmental issues in modern society (Bergmann et al., 2015). Their decomposition time can take up to 500 years, representing a serious threat to aquatic life once inserted into these ecosystems, which is done on large scales due to many reasons, notably inadequate policies on plastic disposal and recycling (Chamas et al., 2020).
Plastics are usually synthetic polymers, mostly originating from petrochemicals (Wayman & Niemann, 2021). There are various types of plastics, with the most common ones having a carbon-carbon backbone, such as polyethylene (PE), polypropylene (PP), and polystyrene (PS). However, some plastics, like polyethylene terephthalate (PET) and nylon, also contain additional heteroatoms (Zeghal et al., 2021). Depending on the polymer's structure and density, the plastic debris can either sink or float, accumulating on the surface of the water, or they can be ingested by aquatic animals. Either way, they negatively affect the ecosystem.
Because of their composition of mostly hydrocarbons, plastics are chemically energetically rich, and some organisms are capable of breaking them down as a source of energy (Yoshida et al., 2016). This process requires specific enzymes depending on the type of plastic and the process requires a large expense of energy to break down the carbon-carbon bonds in polymers. Thus, identifying the organisms capable of plastic degradation is an essential task to deal with the ever-growing problem of plastic pollution.
Due to the metabolic diversity and versatility of fungi and their role as decomposers, they are one of the main organisms that could potentially have a key role in plastic degradation. Although many studies focus on the role of terrestrial fungi as plastic decomposers, not as much is known about the plastic degrading potential of aquatic fungi, although they could be the key to dealing with marine and freshwater plastic pollution. In the research done so far, many species of fungi, especially in microbial biofilms coating plastic fragments, have shown to be capable of breaking down various types of plastic (Vaksmaa et al., 2023).
Recently, efforts have been put into identifying the enzymes produced by fungi that are capable of degrading plastic and the conditions under which these reactions take place. A study done by Zeghal et al. (2021) investigated the literature and genetic investigation on plastic-degrading fungi and showed that these enzymes are not limited to specific constraints, allowing fungi to target a wide range of plastic compounds. Notably, enzymes like manganese peroxidase (MnP) and lignin peroxidase (LiP), commonly associated with lignin degradation, play a significant role in catalyzing oxidation-reduction reactions involving free radicals. This process transforms various plastic compounds into oxidized or polymerized products. Additionally, laccase, a multicopper oxidase with well-established roles in lignin modification, may be involved in plastic degradation by mediating the oxidation of the polymer's carbon backbone. These enzymes showcase the adaptability and versatility of fungi in breaking down plastic materials, as shown in Figure 23.
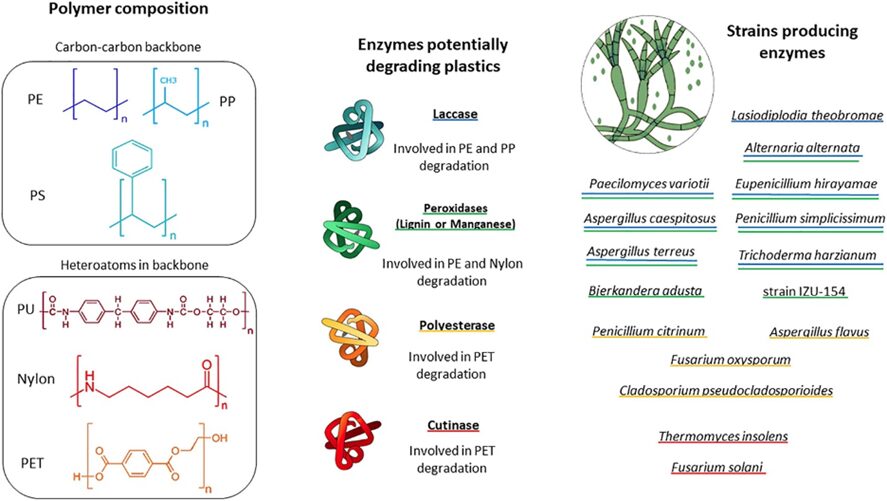
Fig. 23: Diagram illustrates key plastic types commonly found in marine environments. It also highlights fungal enzymes and fungal groups that may play a role in plastic degradation. Fungi capable of producing each enzyme are marked with color-specific underlines (Zeghal et al., 2021).
It is noticeable that aquatic fungi, therefore, have great potential in the bioremediation of plastic debris. Thus, more research has to be done to understand the involvement of fungi in plastic degradation in aquatic environments, since it could be a crucial step in mitigating the plastic pollution crisis.
Biodegradation of organic pollutants
Finally, the issue of organic pollutants is a significant environmental concern due to their widespread presence and detrimental effects on ecosystems and human health. Organic pollutants encompass a broad range of compounds, including oils, gasoline, dyes, pesticides, fertilizers, and polycyclic aromatic hydrocarbons (PAHs)
One of the main applications of mycoremediation is in the clean-up of polycyclic aromatic hydrocarbons (PAH). PAH are chemicals of organic nature, presenting fused benzene rings, specifically pyrene, anthracene, chrysene, phenanthrene, 2-methyl naphthalene, and acenaphthene (Akhtar & Mannan, 2020), as seen in Figure 24. They are released as a product of the incomplete combustion of organic materials, such as petroleum, wood, and coal, and can contaminate the air, soil, and water, being one of the major pollutants of the environment. On the other hand, PAHs are also the most degradable compounds by fungi, especially under aerobic conditions, given that they are hydrocarbons (Thacharodi et al., 2023).
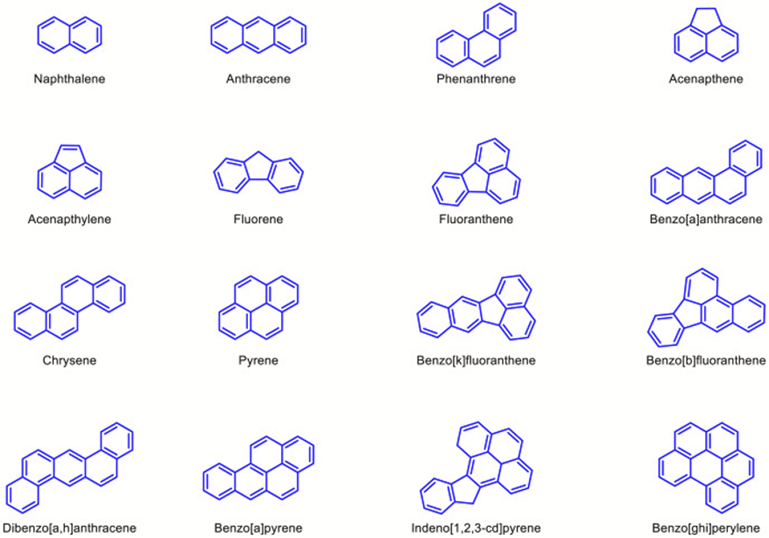
Fig. 24: Molecular structure of PAHs that are enlisted as top priority pollutants by US EPA (Thacharodi et al., 2023).
In aquatic environments, the introduction of PAHs can occur in many forms, mainly by oil spills, marine industries, effluent run-off, and rain, due to the pollution of the atmosphere by many other sources. To remove them from the water and prevent fauna, flora, and human contamination, mycoremediation can be used once aquatic fungi possess intricate degradation mechanisms that enable them to break down various hydrocarbons. This capability arises from their diverse enzyme cassettes, allowing them to utilize different hydrocarbons as their primary sources of carbon and energy. Fungi can oxidize unsaturated, branched-chain aliphatic, and cyclic alkanes in several pathways, generating epoxides, alcohols, diols, and carboxylic acids as metabolic byproducts (AbuQamar et al., 2023).
Moreover, other types of organic pollutants are also a source of concern. Oils, such as crude oil and petroleum products, are notorious for their devastating impact on aquatic environments, often resulting from oil spills and industrial discharges. Gasoline, a common fuel source, can contaminate soil and water, posing risks to both terrestrial and aquatic ecosystems. Dyes, used extensively in textile and industrial processes, can be released into water bodies, leading to water quality issues and potential toxicity.
Through fungi bioremediation mechanisms, these issues can be addressed to minimize their impacts on nature and society. For dyes, fungi-mediated decolorization, degradation, and removal of dyes have been majorly used to avoid and remediate contamination from industrial effluent (Senthilkumar et al., 2014). Fungi promote the enzymatic degradation of dyes after absorbing them through many intra- and extracellular enzymes, depending on the chemical composition of the coloring substances (Husain, 2006), being a powerful and efficient tool for their bioremediation.
Another source of organic pollutants that deserves attention in the context of marine and especially freshwater ecosystems is contamination from pesticides and herbicides. These chemicals represent a great threat to aquatic life since their insertion into these environments causes imbalances in the biomass and introduces exotic substances, greatly affecting the animal and plant populations. Additionally, the natural removal of these substances can be extremely slow (Aravinna et al., 2017), possibly changing the ecosystem permanently and irreversibly.
Recently, many studies have shown that mycoremediation can also be a solution to this problem. By studying contaminated bodies of water, species of aquatic fungi that are able to resist these chemicals can be found and used to bioaccumulate and promote the enzymatic degradation of these chemicals, removing them from the water (Carles et al., 2017). This could effectively mean saving and restoring many ecosystems affected by the introduction of pesticides and herbicides.
These are only some examples of the potential pollutants that could be managed and removed by aquatic fungi. With their enzymatic activity, they can break down various chemicals, making them less toxic. The effectiveness and safety of this process depend on the pollutant, the fungal species involved, and many other environmental conditions, potentially affecting the species that feed on aquatic fungi. However, mycoremediation is an important tool that represents hope in the process of cleaning the environment from the substances that society inputs into the ecosystem daily. As a largely understudied group, this potential only adds to the need for further research into the wonders and fascinating diversity of aquatic fungi.
Conclusion
The chemical properties of aquatic fungi are worthwhile investigating as they solve problems ranging from simple nutrient acquisition to complex breakdown of water pollutants. First, this paper explored the intricate hyphal structures of aquatic fungi, and how both septate and coenocytic hyphae solve significant, yet different conundrums that aquatic fungi face. Coenocytic hyphae allow direct uptake of nutrients into the cytoplasm from the fungi's surroundings, making feeding as efficient as possible, which is crucial for growing hyphae. While septate fungi make the uptake of nutrients slower, they ensure that if a hypha were to rupture, only one unit would lose its cytoplasm and contents, and the other units would stay intact and survive.
Second, to ensure controlled water flow, hydrophobin proteins seal fungi cells while aquaporin proteins regulate flow in a way optimal for the cells. Next, chemotaxis was investigated, showing that fungi are attracted to nutrient-dense substances. Having such an “internal compass” is useful in large bodies of water where nutrients are scarce or far apart. Chemotaxis also plays a role in aquatic fungi reproduction, as the release of sirenin encourages prolonged movement of male gametes toward female ones, and the release of parisin attracts female gametes to male spores. This is especially useful in aquatic environments, where spores have little control over where they end up due to currents. Interestingly, this design solution can help with human contraception, as sirenin can be modified to block calcium ion channels in human sperm.
Third, quorum sensing used by aquatic fungi as a form of communication was examined. Quorum sensing allows fungi to sense where their fellow fungi are in the water through the release of various signaling compounds which are an indicator of aquatic fungi density.
Fourth, aquatic fungi's ability to move nutrients into other trophic levels was explored. Through their unique enzymes, aquatic fungi break down coarse particle organic matter, gaining nutrients and energy, but also making it palatable for other aquatic organisms who do not have the enzymes needed for this breakdown. The various structures of aquatic hyphomycete spores are specially designed to attach to leaves such that contact with the greatest amount of surface area is achieved to optimize chemical reactions. Additionally, aquatic fungi are engineered such that they digest food outside of their body and absorb only the nutrients. This is useful since they live in their nutrient source (substrate).
Finally, the fascinating filtration properties of aquatic fungi are investigated. To humans, plastics, oils, pesticides, heavy metals, and organic pollutants are seen as waste, but to aquatic fungi, they are a source of nutrients. With the help of fungi, some amounts of these (as well as other pollutants) can slowly be removed from the Earth's bodies of water. The intricate adaptations and multifaceted capabilities of aquatic fungi not only shed light on their survival strategies but also hold promise for addressing pressing environmental challenges.
References
AbuQamar, S. F., Abd El-Fattah, H. I., Nader, M. M., Zaghloul, R. A., Abd El-Mageed, T. A., Selim, S., Omar, B. A., Mosa, W. F., Saad, A. M., El-Tarabily, K. A., & El-Saadony, M. T. (2023). Exploiting fungi in bioremediation for cleaning-up emerging pollutants in aquatic ecosystems. Marine Environmental Research, 190, 106068. https://doi.org/https://doi.org/10.1016/j.marenvres.2023.106068
Akhtar, N., & Mannan, M. A.-u. (2020). Mycoremediation: Expunging environmental pollutants. Biotechnology Reports, 26, e00452. https://doi.org/https://doi.org/10.1016/j.btre.2020.e00452
Aravinna, P., Priyantha, N., Pitawala, A., & Yatigammana, S. K. (2017). Use pattern of pesticides and their predicted mobility into shallow groundwater and surface water bodies of paddy lands in Mahaweli river basin in Sri Lanka. Journal of Environmental Science and Health, Part B, 52(1), 37-47.
Archer, D. B., & Wood, D. A. (1995). Fungal Exoenzymes. In N. A. R. Gow & G. M. Gadd (Eds.), The Growing Fungus (pp. 137-162). Springer Netherlands. https://doi.org/10.1007/978-0-585-27576-5_7
BD Editors. (2018, January 21). Hyphae vs. mycelium. Biology Dictionary. https://biologydictionary.net/hyphae-vs-mycelium/
BD Editors. (2019, April 25). Hyphae vs. mycelium. Biology Dictionary. https://biologydictionary.net/hyphae-vs-mycelium/
Becker, Andrea. (2018, March 13). Septate vs. Non-Septate Hyphae. sciencing.com.
Retrieved from https://sciencing.com/septate-vs-nonseptate-hyphae-21818.html
Bergmann, M., Gutow, L., & Klages, M. (2015). Marine anthropogenic litter. SpringerOpen. https://doi.org/10.1007/978-3-319-16510-3
Blaschke-Hellmessen, R. (1999). [Habitats for Candida in medical and hygienic respects]. Mycoses, 42 Suppl 1, 22-29. https://doi.org/10.1111/j.1439-0507.1999.tb04522.x (Standorte für Candida aus medizinisch-hygienischer Sicht.)
Boriová, K., Čerňanský, S., Matúš, P., Bujdoš, M., & Šimonovičová, A. (2014). Bioaccumulation and biovolatilization of various elements using filamentous fungus Scopulariopsis brevicaulis. Letters in Applied Microbiology, 59(2), 217-223. https://doi.org/10.1111/lam.12266
Carles, L., Rossi, F., Joly, M., Besse-Hoggan, P., Batisson, I., & Artigas, J. (2017). Biotransformation of herbicides by aquatic microbial communities associated to submerged leaves. Environmental Science and Pollution Research, 24, 3664-3674.
Cecchi, G., Marescotti, P., Di Piazza, S., & Zotti, M. (2017). Native fungi as metal remediators: Silver myco-accumulation from metal contaminated waste-rock dumps (Libiola Mine, Italy). Journal of environmental science and health. Part. B, Pesticides, food contaminants, and agricultural wastes, 52(3), 191-195. https://doi.org/10.1080/03601234.2017.1261549
Chakraborty, S. (2023). Bioaccumulation and Impact on Aquatic Fauna and Human.
Chamas, A., Moon, H., Zheng, J., Qiu, Y., Tabassum, T., Jang, J. H., Abu-Omar, M., Scott, S. L., & Suh, S. (2020). Degradation Rates of Plastics in the Environment. ACS Sustainable Chemistry & Engineering, 8(9), 3494-3511. https://doi.org/10.1021/acssuschemeng.9b06635
Chamier, A.-C. (1985). Cell-wall-degrading enzymes of aquatic hyphomycetes: a review. Botanical Journal of the Linnean Society, 91(1-2), 67-81.
Cole, R. J., Jarvis, B. B., & Schweikert, M. A. (2003). 8 – Antheridiols and Oogoniols. In R. J. Cole, B. B. Jarvis, & M. A. Schweikert (Eds.), Handbook of Secondary Fungal Metabolites (pp. 249-270). Academic Press. https://doi.org/https://doi.org/10.1016/B978-012179460-6/50318-7
Frid, C. L. J., & Caswell, B. A. (2017). Marine Pollution. Oxford University Press.
Dang, C., Gessner, M., & Chauvet, E. (2007). Influence of conidial traits and leaf structure on attachment success of aquatic hyphomycetes on leaf litter. Mycologia, 99, 24-32. https://doi.org/10.3852/mycologia.99.1.24
Dehghani, A., Roohi Aminjan, A., & Dehghani, A. (2022). Trophic transfer, bioaccumulation, and health risk assessment of heavy metals in Aras River: case study-Amphipoda-zander -human. Environmental Science and Pollution Research, 3. https://doi.org/10.1007/s11356-021-18036-7
Ferreira, V., & Chauvet, E. (2011). Synergistic effects of water temperature and dissolved nutrients on litter decomposition and associated fungi. Global Change Biology, 17(1), 551-564.
Frid, C. L. J., & Caswell, B. A. (2017). Marine Pollution. Oxford University Press. https://doi.org/10.1093/oso/9780198726289.001.0001
Fungi. Organismal Biology. (n.d.). https://organismalbio.biosci.gatech.edu/biodiversity/fungi-2/
Gao, H., Xie, Y., Hashim, S., Akhtar Khan, A., Wang, X., & Xu, H. (2018). Application of microbial technology used in bioremediation of urban polluted river: a case study of Chengnan River, China. Water, 10(5), 643.
Gautam, R., Sharma, S., Mahiya, S., & Chattopadhyaya, M. (2014). Heavy Metals In Water : Presence, Removal and Safety.
Gessner, M., Gulis, V., Kuehn, K., Chauvet, E., & Suberkropp, K. (2007). 17 fungal decomposers of plant litter in aquatic ecosystems. Environmental and microbial relationships, 4, 301.
Given, S., Pendleton, L. H., & Boehm, A. B. (2006). Regional Public Health Cost Estimates of Contaminated Coastal Waters: A Case Study of Gastroenteritis at Southern California Beaches. Environmental Science & Technology, 40(16), 4851-4858. https://doi.org/10.1021/es060679s
Gow, N. A. R., & Lenardon, M. D. (2023). Architecture of the dynamic fungal cell wall. Nature Reviews Microbiology, 21(4), 248-259. https://doi.org/10.1038/s41579-022-00796-9
Hawksworth, D. L., & Lücking, R. (2017). Fungal diversity revisited: 2.2 to 3.8 million species. Microbiology spectrum, 5(4), 10.1128/microbiolspec. funk-0052-2016.
Heeger, F., Bourne, E. C., Wurzbacher, C., Funke, E., Lipzen, A., He, G., Ng, V., Grigoriev, I. V., Schlosser, D., & Monaghan, M. T. (2021). Evidence for Lignocellulose-Decomposing Enzymes in the Genome and Transcriptome of the Aquatic Hyphomycete Clavariopsis aquatica. J Fungi (Basel), 7(10). https://doi.org/10.3390/jof7100854
Husain, Q. (2006). Potential applications of the oxidoreductive enzymes in the decolorization and detoxification of textile and other synthetic dyes from polluted water: a review. Critical reviews in biotechnology, 26(4), 201-221.
Kadri, T., Rouissi, T., Brar, S. K., Cledon, M., Sarma, S., & Verma, M. (2017). Biodegradation of polycyclic aromatic hydrocarbons (PAHs) by fungal enzymes: A review. Journal of environmental sciences, 51, 52-74.
Kumar, D., Singh, S. K., Arya, S. K., Srivastava, D., Rajput, V. D., & Husain, R. (2023). Chapter 9 – Multifunctional growth-promoting microbial consortium-based biofertilizers and their techno-commercial feasibility for sustainable agriculture. In J. A. Parray, N. Shameem, D. Egamberdieva, & R. Z. Sayyed (Eds.), Rhizobiome (pp. 167-208). Academic Press. https://doi.org/https://doi.org/10.1016/B978-0-443-16030-1.00010-9
Lakna. (2018, August 3). Difference between hyphae and pseudohyphae. Pediaa.Com. https://pediaa.com/difference-between-hyphae-and-pseudohyphae/
Lew, R. R. (2011). How does a hypha grow? The biophysics of pressurized growth in fungi. Nature Reviews Microbiology, 9(7), 509-518. https://doi.org/10.1038/nrmicro2591
Libretexts. (2021, March 6). 8.9: Fungi Structure. Biology LibreTexts. https://bio.libretexts.org/Bookshelves/Introductory_and_General_Biology/Introductory_Biology_(CK-12)/08%3A_Protists_and_Fungi/8.09%3A_Fungi_Structure
Martín-Rodríguez, A. J., Reyes, F., Martín, J., Pérez-Yépez, J., León-Barrios, M., Couttolenc, A., Espinoza, C., Trigos, A., Martín, V. S., Norte, M., & Fernández, J. J. (2014). Inhibition of bacterial quorum sensing by extracts from aquatic fungi: first report from marine endophytes. Mar Drugs, 12(11), 5503-5526. https://doi.org/10.3390/md12115503
Matozzo, V., Adeyi, A. A., Akabuogu, E. P., Idoko, G. O., Okoduwa, S. I. R., Igiri, B. E., & Ejiogu, I. K. (2018). Toxicity and Bioremediation of Heavy Metals Contaminated Ecosystem from Tannery Wastewater: A Review. Journal of Toxicology.(2018).
Medina, E. M., & Buchler, N. E. (2020). Chytrid fungi. Current Biology, 30(10), R516-R520. https://doi.org/https://doi.org/10.1016/j.cub.2020.02.076
Mitra, S., Chakraborty, A. J., Tareq, A. M., Emran, T. B., Nainu, F., Khusro, A., Idris, A. M., Khandaker, M. U., Osman, H., Alhumaydhi, F. A., & Simal-Gandara, J. (2022). Impact of heavy metals on the environment and human health: Novel therapeutic insights to counter the toxicity. Journal of King Saud University – Science, 34(3), 101865. https://doi.org/https://doi.org/10.1016/j.jksus.2022.101865
Moore, D. (1998). Fungal Morphogenesis. Cambridge University Press. https://books.google.ca/books?id=WUcYZNggBecC
Moss, A. S., Reddy, N. S., Dortaj, I. M., & San Francisco, M. J. (2008). Chemotaxis of the amphibian pathogen Batrachochytrium dendrobatidis and its response to a variety of attractants. Mycologia, 100(1), 1-5. https://doi.org/10.1080/15572536.2008.11832493
Muehlstein, L. K., Amon, J. P., & Leffler, D. L. (1988). Chemotaxis in the Marine Fungus Rhizophydium littoreum. Appl Environ Microbiol, 54(7), 1668-1672. https://doi.org/10.1128/aem.54.7.1668-1672.1988
Najeebul, T., Khair Ul, N., Burhan, H., Zaffar, B., Ali Mohd, Y., Mohd Ashraf, D., Fayaz Ahmad, M., Zakir, A., Rabi'atul Adawiyah, A., & Sayyed, R. Z. (2022). Microbial Remediation: A Promising Tool for Reclamation of Contaminated Sites with Special Emphasis on Heavy Metal and Pesticide Pollution: A Review. 10(1358), 1358.
Nikolcheva, L. G., Cockshutt, A. M., & Bärlocher, F. (2003). Determining diversity of freshwater fungi on decaying leaves: comparison of traditional and molecular approaches. Applied and environmental microbiology, 69(5), 2548-2554.
Padder, S. A., Prasad, R., & Shah, A. H. (2018). Quorum sensing: A less known mode of communication among fungi. Microbiological Research, 210, 51-58. https://doi.org/https://doi.org/10.1016/j.micres.2018.03.007
Padmaperuma, G., Butler, T. O., Shuhaili, F. A. B. A., Almalki, W. J., & Vaidyanathan, S. (2020). Chapter 25 – Microbial consortia: Concept and application in fruit crop management. In A. K. Srivastava & C. Hu (Eds.), Fruit Crops (pp. 353-366). Elsevier. https://doi.org/https://doi.org/10.1016/B978-0-12-818732-6.00025-3
Pommerville, J. C., Strickland, J. B., & Harding, K. E. (1990). Pheromone interactions and ionic communication in gametes of aquatic fungusAllomyces macrogynus. J Chem Ecol, 16(1), 121-131. https://doi.org/10.1007/bf01021274
Pommerville, J., & Olson, L. W. (1987). Evidence for a male-produced pheromone inAllomyces macrogynus. Experimental Mycology, 11(3), 245-248. https://doi.org/https://doi.org/10.1016/0147-5975(87)90012-0
Prenafeta-Boldú, F. X., De Hoog, G. S., & Summerbell, R. C. (2018). Fungal communities in hydrocarbon degradation. Microbial Communities Utilizing Hydrocarbons and Lipids: Members, Metagenomics and Ecophysiology; McGenity, TJ, Ed, 1-36.
Raghukumar, S. (2017). Fungi: Characteristics and Classification. In S. Raghukumar (Ed.), Fungi in Coastal and Oceanic Marine Ecosystems: Marine Fungi (pp. 1-15). Springer International Publishing. https://doi.org/10.1007/978-3-319-54304-8_1
Reis, A. C., Čvančarová, M., Liu, Y., Lenz, M., Hettich, T., Kolvenbach, B. A., Corvini, P. F., & Nunes, O. C. (2018). Biodegradation of sulfamethoxazole by a bacterial consortium of Achromobacter denitrificans PR1 and Leucobacter sp. GP. Appl Microbiol Biotechnol, 102(23), 10299-10314. https://doi.org/10.1007/s00253-018-9411-9
Riehl, R. M., Toft, D. O., Meyer, M. D., Carlson, G. L., & McMorris, T. C. (1984). Detection of a pheromone-binding protein in the aquatic fungus Achlya ambisexualis. Exp Cell Res, 153(2), 544-549. https://doi.org/10.1016/0014-4827(84)90623-2
Seena, S., Barros, J., Graça, M. A. S., Arce-Funck, J., & Bärlocher, F. (2022). Aquatic hyphomycete spores: What do we know, where do we go from here? In Freshwater Mycology: Perspectives of Fungal Dynamics in Freshwater Ecosystems (pp. 1-20). https://doi.org/10.1016/B978-0-323-91232-7.00016-7
Senthilkumar, S., Perumalsamy, M., & Prabhu, H. J. (2014). Decolourization potential of white-rot fungus Phanerochaete chrysosporium on synthetic dye bath effluent containing Amido black 10B. Journal of Saudi Chemical Society, 18(6), 845-853.
Sharma, S., & Adholeya, A. (2011). Detoxification and accumulation of chromium from tannery effluent and spent chrome effluent by Paecilomyces lilacinus fungi. International Biodeterioration & Biodegradation, 65(2), 309-317. https://doi.org/https://doi.org/10.1016/j.ibiod.2010.12.003
Singh, P., Singh, V. K., Singh, R., Borthakur, A., Madhav, S., Ahamad, A., Kumar, A., Pal, D. B., Tiwary, D., & Mishra, P. K. (2020). Chapter 1 – Bioremediation: a sustainable approach for management of environmental contaminants. In P. Singh, A. Kumar, & A. Borthakur (Eds.), Abatement of Environmental Pollutants (pp. 1-23). Elsevier. https://doi.org/https://doi.org/10.1016/B978-0-12-818095-2.00001-1
Stelzer, R. S., Heffernan, J., & Likens, G. E. (2003). The influence of dissolved nutrients and particulate organic matter quality on microbial respiration and biomass in a forest stream. Freshwater Biology, 48(11), 1925-1937.
Structure of fungi, septate and coenocytic hyphae. stock vector. Adobe Stock. (n.d.).
https://stock.adobe.com/ca/images/structure-of-fungi-septate-and-coenocytic hyphae/423209784
Sun, X. H., Zhu, Y. Y., Wang, L., Liu, H. L., Ling, Y., Li, Z. L., & Sun, L. B. (2017). The Catsper channel and its roles in male fertility: a systematic review. Reprod Biol Endocrinol, 15(1), 65. https://doi.org/10.1186/s12958-017-0281-2
Syeda, S. S., Carlson, E. J., Miller, M. R., Francis, R., Clapham, D. E., Lishko, P. V., Hawkinson, J. E., Hook, D., & Georg, G. I. (2016). The Fungal Sexual Pheromone Sirenin Activates the Human CatSper Channel Complex. ACS Chemical Biology, 11(2), 452-459. https://doi.org/10.1021/acschembio.5b00748
Tarafder, Entaj & Dutta, Arun & Pradhan, Prakash & Mondal, Bidisha & Chakraborty, Nilanjan & Paloi, Soumitra & Roy, Anirban & Acharya, Krishnendu. (2017). Contribution to the Macromycetes of West Bengal, India: 13–17. Research Journal of Pharmacy and Technology. 10. 1123-1130. 10.5958/0974-360X.2017.00203.7.
Thacharodi, A., Hassan, S., Singh, T., Mandal, R., Chinnadurai, J., Khan, H. A., Hussain, M. A., Brindhadevi, K., & Pugazhendhi, A. (2023). Bioremediation of polycyclic aromatic hydrocarbons: An updated microbiological review. Chemosphere, 328, 138498. https://doi.org/https://doi.org/10.1016/j.chemosphere.2023.138498
Vaksmaa, A., Guerrero-Cruz, S., Ghosh, P., Zeghal, E., Hernando-Morales, V., & Niemann, H. (2023). Role of fungi in bioremediation of emerging pollutants [Review]. Frontiers in Marine Science, 10. https://doi.org/10.3389/fmars.2023.1070905
Vaseem, H., Singh, V., & Singh, M. (2017). Heavy metal pollution due to coal washery effluent and its decontamination using a macrofungus, Pleurotus ostreatus. Ecotoxicology and environmental safety, 145, 42-49.
Voyles, J. (2011). Phenotypic profiling of Batrachochytrium dendrobatidis, a lethal fungal pathogen of amphibians. Fungal Ecology, 4(3), 196-200. https://doi.org/https://doi.org/10.1016/j.funeco.2010.12.003
Voyles, J., Rosenblum, E. B., & Berger, L. (2011). Interactions between Batrachochytrium dendrobatidis and its amphibian hosts: a review of pathogenesis and immunity. Microbes and Infection, 13(1), 25-32. https://doi.org/https://doi.org/10.1016/j.micinf.2010.09.015
Wayman, C., & Niemann, H. (2021). The fate of plastic in the ocean environment–a minireview. Environmental Science: Processes & Impacts, 23(2), 198-212.
Wu, M., Xu, Y., Ding, W., Li, Y., & Xu, H. (2016). Mycoremediation of manganese and phenanthrene by Pleurotus eryngii mycelium enhanced by Tween 80 and saponin. Applied Microbiology and Biotechnology, 100(16), 7249-7261. https://doi.org/10.1007/s00253-016-7551-3
Yellowelanortv@gmail.com. (2015, September 19). Mushroom life cycle. Yellow Elanor. https://www.yellowelanor.com/mushroom-life-cycle/
Yoshida, S., Hiraga, K., Takehana, T., Taniguchi, I., Yamaji, H., Maeda, Y., Toyohara, K., Miyamoto, K., Kimura, Y., & Oda, K. (2016). A bacterium that degrades and assimilates poly (ethylene terephthalate). Science, 351(6278), 1196-1199.
Zeghal, E., Vaksmaa, A., Vielfaure, H., Boekhout, T., & Niemann, H. (2021). The Potential Role of Marine Fungi in Plastic Degradation – A Review [Review]. Frontiers in Marine Science, 8. https://doi.org/10.3389/fmars.2021.738877