An Advantageous Appendage: the Biomechanical Design of Tentacles and their Adaptation for the Aquatic Environment
Yousif Al Shajlawi, Henry Bain, Julianna Bede
Abstract
Amongst the various muscular organs that lack a skeletal support system, whether it be the tongues of terrestrial vertebrates or the trunks of elephants, the tentacle is certainly one of the most fascinating in the animal kingdom (Kier & Smith, 1985). The mechanical properties of this appendage have long been investigated by scientists, so much so that certain innovations in modern-day robotics have taken inspiration from its complex and varied movements (Stella et al., 2022). This paper will explore the biomechanical structure and function of the tentacle, both in the aquatic and terrestrial environment. There will be a particular focus on the biomechanics of the cephalopod tentacle, such as that of the squid and cuttlefish, and its optimized functioning for predation. The advantages and disadvantages of the jellyfish tentacle will be covered, as well as a comparison with plant tendrils to address the benefits of tentacle-like structural characteristics in the terrestrial environment. Finally, this paper will investigate the properties of water and how the tentacle has evolved to be best suited for the aquatic environment.
Introduction
Tentacles can be found in many organisms, including cephalopods, cnidarians, and gastropods. There are also similar structures in plants like redvine and the common green bean called tendrils. Tentacles serve many functions that vary among these different organisms. Some of these functions are predation, support, and sensation. Predation is the most common function found in aquatic species. Some aquatic creatures, like the octopus and squid, use their tentacles to capture prey with sudden and rapid movement, which requires mechanisms such as elongation, shortening, bending, and torsion. Other aquatic creatures, like jellyfish, use tentacles to paralyze prey. Surprisingly, however, jellyfish tentacles decrease swimming performance because of the mechanism jellyfish use to swim.
Many tentacles, especially those used for predation, are found in aquatic environments. This is no coincidence because water creates an ideal environment where limbs do not need skeletal bones and provides the ability for these flexible structures to move in various ways without a significant expenditure of energy. Water’s density and viscosity, which provide a force to counteract Earth’s gravity, allow tentacles to thrive in aquatic environments.
Cephalopod tentacle structure
The cephalopod family includes several noteworthy animals which have tentacles, the most famous being the octopus and several species of squid (see Figure 1 below). Although octopuses technically do not have tentacles but rather have arms, this paper plays with the definition of a tentacle and considers many similar structures. Cephalopods use their tentacles primarily for catching prey, whereas their arms are used for swimming and manipulating food (Kier, 1985). Another difference is that the arms have suction cups lining all of one side whereas the tentacles only have suction cups at the tip. These two distinct structures are also similar in many ways, one of the most important being that they lack a skeleton and are made mainly of muscle.

Fig. 1. Anatomy of the giant squid, including its two extensible tentacles (“Giant squid anatomy,” n.d.).
Typically, muscles require some sort of skeletal structure to function; they need something solid to attach to. However, cephalopod appendages do not contain a skeleton, so the muscle must act as its own support system (Kier, 1982). A hydrostatic skeleton does not contain a rigid structure. Animals with hydrostatic skeletons include worms and anemones, and structures like trunks and tentacles are included as well. They rely on internal pressure to apply force (Kier, 2012). Because these structures are of a relatively constant volume, elongation of one dimension involves the shrinking of another. For instance, if a squid’s tentacle is imagined to be a cylinder of constant volume, the length of the cylinder cannot increase without the radius decreasing.
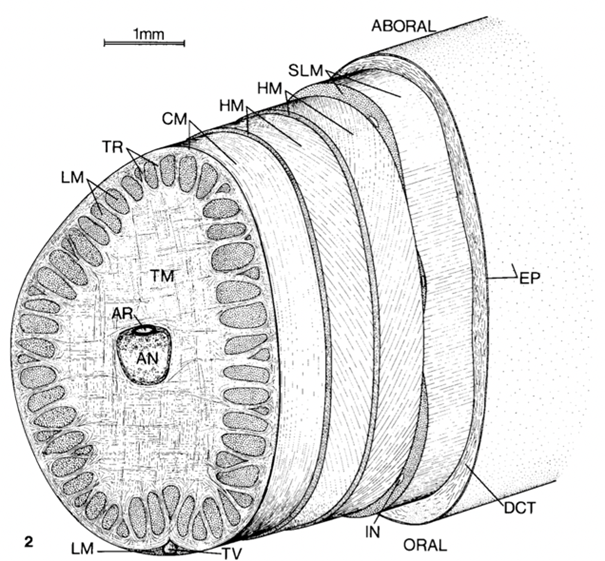
Fig. 2. Tentacle cross section showing the muscle layout. TM-transverse muscle, LM-longitudinal muscle, HM-helical muscle, CM-circular muscle, AR-artery, DCT-dermal connective tissue, EP-epithelium, IN-intramuscular nerve cord, SLM-superficial longitudinal muscle, TR-trabeculae of transverse muscle, TV-superficial tentacular vein (Kier, 1982).
A squid’s tentacle contains one central nerve cord that is accompanied by a central artery. This central axis is surrounded by the most predominant organ, transverse muscle (Kier, 1982). Figure 2 shows a tentacle cross-section outlining the muscle arrangements. The name “transverse muscle” describes the direction in which the muscle fibers are oriented relative to the tentacle’s central axis. These transverse muscle fibers are perpendicular to the central nerve of the tentacle. The retraction of the transverse muscle decreases the radius of the axis, which has led to the theory that tentacle elongation is caused by retraction of the transverse muscle (Kier, 1982). Longitudinal muscle fibers occur in clumps around the outer edge of the tentacle when a crosswise section is cut. These longitudinal fibers are parallel to the central axis, as seen in Figure 3 below. Sections of transverse and longitudinal fibers alternate around the outer layer of the tentacle.
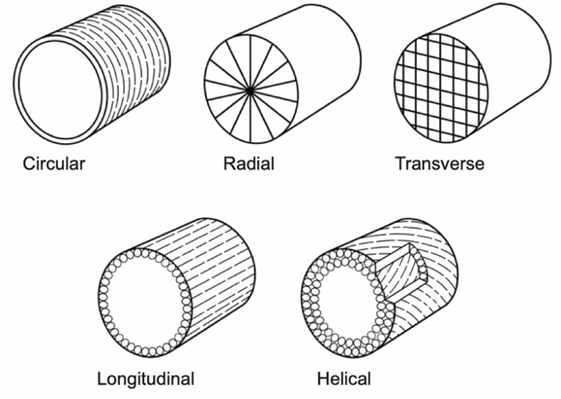
Fig. 3. Diagram showing how different muscle fiber types are oriented relative to the central axis of the tentacle (Kier, 2012).
Some fascinating muscle structures of the tentacle are the helically oriented fibers surrounding a thin circular layer of muscle. The word helix brings to mind the structure of DNA and indeed these muscles, called oblique muscles, are quite similar: two separate helices wrap in opposite directions to form a double helix. The inner helical muscle fibers wrap to the right and the outer fibers wrap to the left (Kier, 1982). Much like a DNA double helix stretching, as the tentacle extends, the angle that each helix makes with the central axis decreases. When the tentacle is retracted, this angle is about 67o; when extended, it is about 37o (Kier, 1982). These oblique muscles are tense even when the tentacle is not extended. Rather than the muscle stretching as the tentacle extends, the helix unwinds to form a smaller angle with the central axis (Kier, 2012). This resting tension provides a firm layer that resists deformation. If considered separately, each helical muscle can be imagined as a means for the tentacle to rotate, as outlined in Figure 4. If one of the helices contracts, it will spin the tentacle about its own axis, and if the other contracts the tentacle will spin in the other direction.
Function of the cephalopod tentacle
Tentacles are particularly interesting organs in that they do not possess a skeletal support system, although they are capable of varied, complex, and controlled movements (Kier & Smith, 1985). As for other muscular-hydrostats –muscular organs whose movement and support both result from their musculature as opposed to a distinct skeletal system– one of the most important features of tentacles is that their volume remains constant (Kier & Smith, 1985). As a result, a change in one dimension will always cause a compensatory change in another dimension (Kier & Smith, 1985). This feature is of particular interest when discussing the principal mechanisms of the appendage: bending, shortening, elongation, and torsion.

Fig. 4. This diagram shows how a tentacle could theoretically rotate about its central axis via helical muscle contraction. The spiral represents the muscle, the cylinder represents the tentacle, and the black line can be used as a reference (Kier, 1982).
Force generation
The forces required to conduct the rapid and varied movements of a tentacle are generated by the contraction of muscle fibers and associated connective tissue, as well as the intramuscular pressure differences between neighboring muscle segments (Van Leeuwen et al., 2000). The force generated by a particular muscle bundle can be calculated using Newton’s Second Law with a determined lumped mass (m) and the resultant of the aforementioned forces (F), as seen in (1). Subsequent integrations can then be performed to determine the velocity (v) and length (l) of the tentacle segment from its acceleration (a) as it undergoes a given movement, such as elongation (see (2), (3)). From these, the corresponding muscle fiber strain and strain rates can be found, which contribute to the overall muscle force mechanism (Van Leeuwen et al., 2000). A simplified procedure for deriving the longitudinal strain of a tentacle segment (εl) and its strain rate (εl) (see (4), (5), respectively), is shown below (Van Leeuwen et al., 2000):
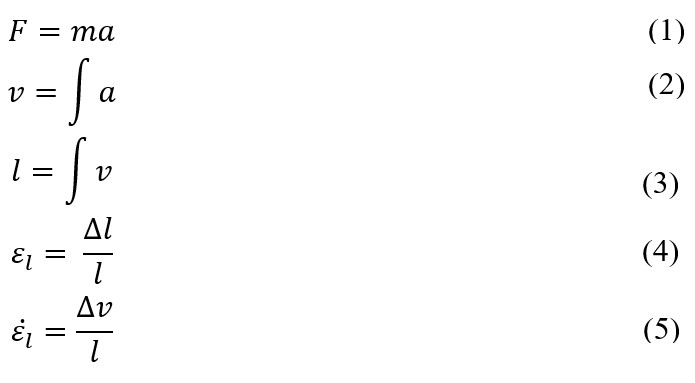
The optimization of the acceleration and peak velocity of the tentacle is indeed one of its most important characteristics. In fact, the short sarcomeres of the transverse and circular muscle fibers are arranged in series, and because the shortening velocity of elements in series is additive, the contraction of the relatively short sarcomeres results in higher overall strain rates (Kier, 2016; Van Leeuwen et al., 2000). While the length of the sarcomere filaments means that they generate less force than longer filaments, the external mass moved by the muscular stalk, which includes the terminal club and a small mass of water, is relatively small (Van Leeuwen et al., 2000). The rapid rate of extension strain is also a result of the thickness of the muscle filaments, which contributes to the tentacle’s rapid acceleration during prey strike (Van Leeuwen et al., 2000).
The nature of muscle filaments is also what differentiates the tentacles and arms of cephalopods. In fact, the thick filaments of muscle fibers in the tentacles of cephalopods were found to be one-tenth the length of the filaments in arm muscles, and thus the maximum overall shortening velocity of the arm fibers would be significantly less than that of the tentacle (Kier, 2016). Additionally, while tentacles contain cross-striated muscles in their transverse muscle mass, arms contain obliquely-striated muscles which favor the slow bending and torsional movements that are favored for prey handling, as opposed to the rapid elongation necessary for prey strike (Gilly et al.,2020).
Elongation
A common movement of the tentacle by cephalopods such as the squid is elongation. Indeed, because the volume of the appendage must remain constant, an extension of the tentacle requires the contraction of muscles that decreases its cross-sectional area (Kier & Smith, 1985). As mentioned above, this occurs through the contraction of musculature, which lies perpendicular to the long axis of the tentacle, such as the transverse, circular, and radial muscles seen in Figures 5A and 5B (Kier & Smith, 1985). The tentacle is therefore not considered an auxetic structure, defined as an object whose overall volume changes as it undergoes geometrical variation (Stavric & Wiltsche, 2019). In fact, while the longitudinal increase in dimension of an auxetic structure increases its dimension in the transverse direction, the tentacle behaves like a typical elastic material which will compensate for a change in dimension in order to maintain a constant volume. The behavior of a material when it undergoes such a deformation as elongation can be described using Poisson’s ratio, which is the ratio between the negative transverse strain divided by the longitudinal strain. Indeed, this ratio is positive for the tentacle because the strains are always opposite in nature, with tension in one direction resulting in compression in the other. Auxetic structures, on the other hand, will have a negative Poisson’s ratio (Stavric & Wiltsche, 2019).
This muscle system of the tentacle possesses a biomechanical advantage in that it imitates the lever systems which are common in limbs containing a skeletal structure (Kier & Smith, 1985). Leverage occurs when either the input force results in a larger output force, or the displacement is amplified so that the resultant displacement/velocity is greater than the applied displacement/velocity (Kier & Smith, 1985). Such a system is demonstrated in Figure 6. In the case of tentacles that possess a high initial length-to-width ratio in the retracted state, a small decrease in the width will result in a proportionally larger (displacement) and faster (velocity) increase in the length (Kier & Smith, 1985). Not only does this mechanism benefit the organism in that only a small change in diameter is required to create a proportionately greater extension of the tentacle, but it also results in rapid prey strike (Kier, 2016). The ability to rapidly extend the tentacle can also be attributed to the high shortening velocity of transverse and circular muscle fibers, as described above (Kier, 2016).
The loliginid squid exemplifies this biomechanical advantage. In fact, this cephalopod’s tentacles can strike prey in 20-40 ms with a peak velocity of 2 m/s and a peak acceleration of 250 m/s2. This involves a 40-80% elongation of the appendage (Kier, 2016). Fascinatingly, these values are amongst the highest observed for an animal movement that does not rely on elastic energy storage mechanisms (Gilly et al., 2020). After striking, the tentacle’s suckers will adhere to the prey and the tentacle retracts to draw it closer, after which the arms are used to manipulate the subject (Kier, 2016).
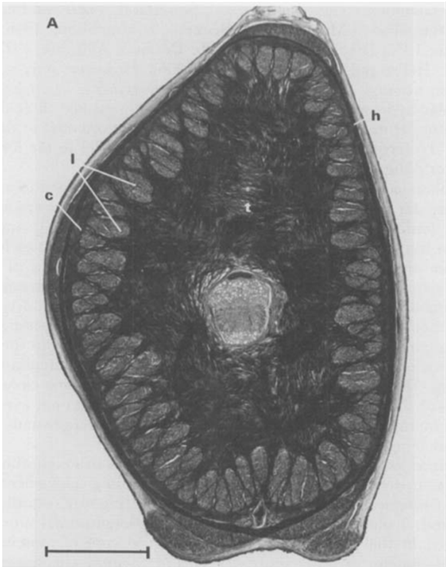
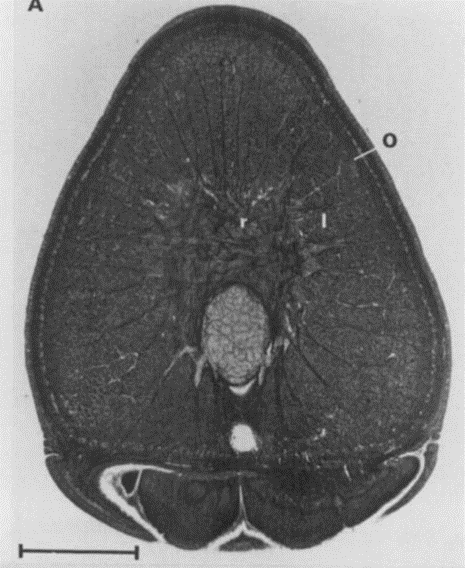
Fig. 5. A. Cross-section of a tentacular stalk of the squid, Loligo pealei (Adapted from Kier & Smith, 1985). Bundles of longitudinal muscle fibers (l), the circular muscle layer (c), the transverse muscle mass (t), and the helical muscle fibers (h) can be observed. B. Cross-section of the tentacle of the chambered nautilus, Nautilus pompilius. Radial muscle fibers (r), longitudinal muscle bundles (l), and a layer of obliquely-arranged muscle (o) can be observed.
Shortening
While the extension of the tentacle involves the contraction of transverse muscles, its shortening is often associated with the contraction of longitudinal muscle bundles that extend along the entire appendage (see Figure 5A and 5B) (Kier, 2016). Since these longitudinal muscles operate over a greater distance than the transverse muscles that control cross-sectional area, and given the resistance of the tentacle to volume change, there is a mechanical advantage to this leverage mechanism given that the force applied by the contracting muscles results in a proportionately greater resultant force applied to the transverse muscles (see Figure 6B) (Kier, 2016; Kier & Smith, 1985). In other words, because the displacement (d) of the transverse, circular, and radial muscle fibers is smaller than that of the longitudinal muscles, less work (W) is required to generate a given force (F) according to the equation:

This resultant force, therefore, induces the re-elongation of the transverse and circular muscle fibers and an increase in cross-sectional area. Consequently, the longitudinal and transverse muscles are antagonistic in nature (Kier, 2016; Kier & Smith, 1985).
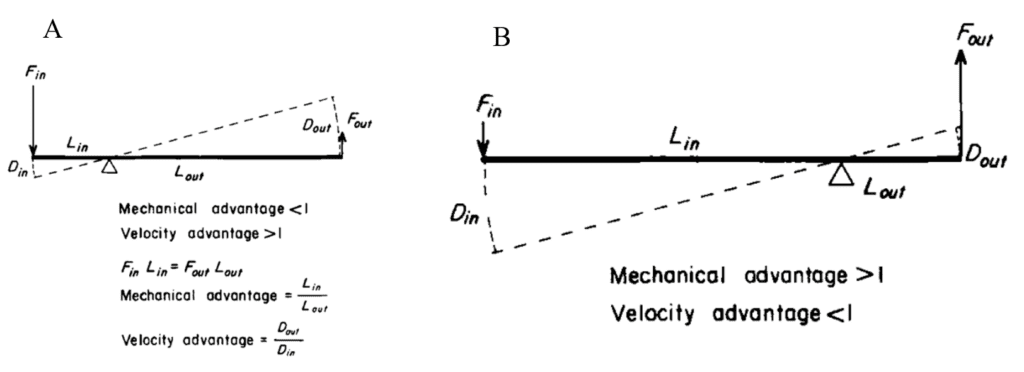
Fig. 6. A. Diagram depicting the velocity advantage of lever systems as found in the tentacles of squid (Adapted from Kier & Smith, 1985). When the circular muscles contract, the decrease in the diameter of the tentacle stalk, or Din, is smaller than the resulting longitudinal displacement of the tentacle during extension, Dout. The extension speed is therefore amplified in relation to the speed of the original movement. B. Diagram depicting the mechanical advantage of lever systems in which there is a high initial length-to-width ratio, as during shortening of the tentacle. In such a case, the input force of the contracting longitudinal muscles creates a larger output force applied to the re-elongating transverse, circular, and radial muscles.
Bending
One of the most important movements of a muscular hydrostat is bending. This occurs through the contraction of longitudinal muscles on one side of the appendage; however, this longitudinal compression force must be resisted on the opposite side to prevent shortening without bending (Kier, 2016; Kier & Smith, 1985). In order to resist shortening while maintaining the constant volume of the organ, the diameter of the tentacle must remain constant through the simultaneous contraction of transverse, radial, or circular muscles (Kier & Smith, 1985). This results in a unilateral decrease in length, as seen in Figure 7a. Alternatively, bending can occur by reducing the diameter of the tentacle via contraction of the transverse, radial, or circular muscles while maintaining the unilateral length through contraction of longitudinal musculature on one side of the appendage (see Figure 7b) (Kier & Smith, 1985).
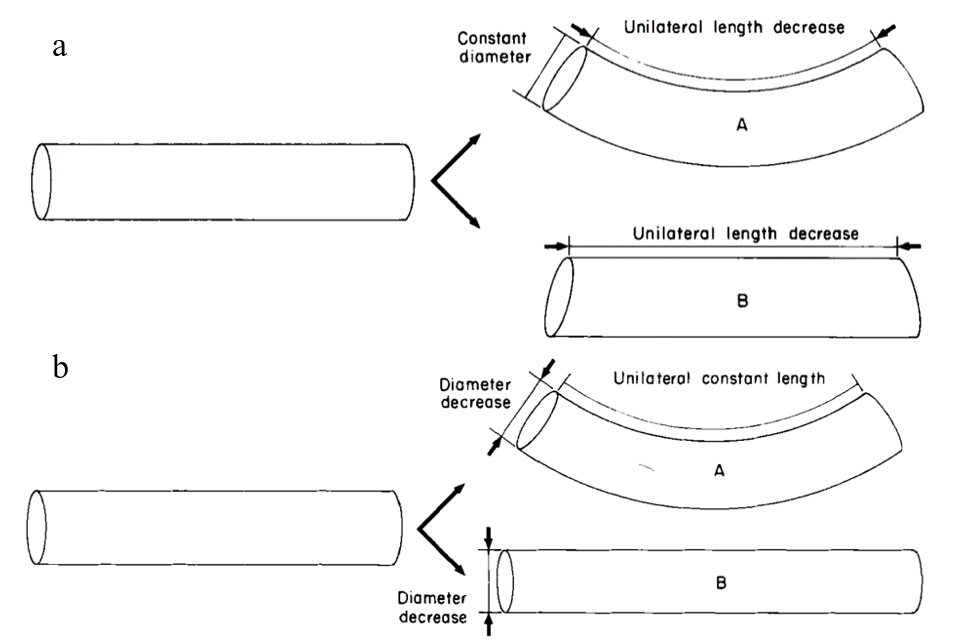
Fig. 7. a. Cylindrical model demonstrating the bending of a tentacle through a decrease in unilateral length and the maintenance of a constant diameter (Adapted from Kier & Smith, 1985). Without the latter mechanism, the tentacle would simply experience a decrease in unilateral length without bending, as in B. b. Cylindrical model depicting bending as a result of diameter decrease and constant unilateral length. Without the latter mechanism, the tentacle would increase in length without bending.
These longitudinal muscle bundles are present around the entire periphery of the organ, as seen in the tentacles of the Loligo pealei and Nautilus pompilius in Figures 5A and 5B, respectively. (Kier, 2016; Kier & Smith, 1985). This allows bending to occur in any plane (Kier & Smith, 1985), thus conferring a biomechanical advantage to organisms with tentacles, as opposed to non-flexible appendages used for predation, such as claws or pincers. Like tentacles, the arms of squid and cuttlefish contain especially large longitudinal muscles on the oral side of the cross-section to support prey handling during feeding (Kier, 2016).
The location of the longitudinal muscle bundles around the circumference of the tentacle is also mechanically advantageous. Indeed, the further these muscles are from the neutral axis of the structure, the greater the bending moment, M, according to the following equation, where d is the perpendicular distance from the neutral plane to the line of action of the contractile force, F (Kier & Smith, 1985):

Indeed, the rapid movement of the appendage in any plane requires a complex neuromuscular system that can coordinate the simultaneous actions of various muscles (Kier & Smith, 1985).
Torsion
During a prey strike, the elongation of the tentacle is often coupled with twisting so that the tentacle club containing the suckers strikes the prey (Kier, 2016). This torsion is created by the contraction of the right- and left-handed helical muscle layers surrounding the tentacle, thus causing it to twist about the longitudinal axis (see Figure 4). The direction of torsion depends on the handedness of the helical musculature, with the right-hand helix causing counter-clockwise torsion and the left-hand helix causing clockwise torsion (Kier & Smith, 1985). Again, the peripheral location of these muscles enables a larger moment to be created (Kier & Smith, 1985).
These helical muscles must also adjust their path length to accommodate changes in length of the tentacle during elongation and shortening (Kier, 2016). This can be described through changes in fiber angle, which is the angle between the helical muscle fibers and the long axis (Kier & Smith, 1985). These dimensions are demonstrated in the cylindrical model of Figure 8A below.
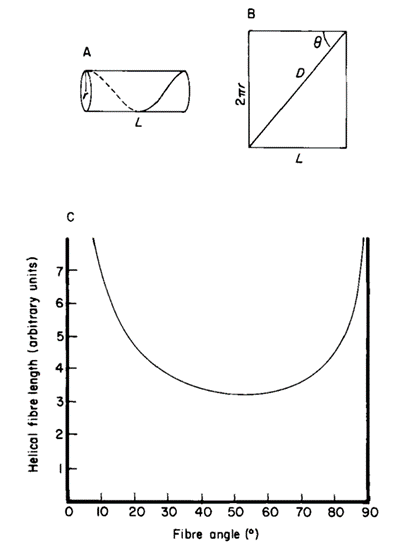
Fig. 8. A. Cylindrical model showing the dimensions of the tentacle, where D represents the length of the helical muscle, q is the fibre angle, and L is the length of stalk. B. Graph of the helical fibre length as a function of the fibre angle (Adapted from Kier & Smith, 1985).
As seen in Figure 8B, the fibers reach a minimum length when the fiber angle is 54°44′ and reach a maximum length at 0° or 90°. Therefore, helical muscle fibers with a fiber angle greater than 54°44′ (in a retracted tentacle) create force for torsion and elongation, while those with a fiber angle less than 54°44′ (in an elongated tentacle) generate force for torsion and shortening (Kier & Smith, 1985). For example, the fiber angle in the helical muscle layers of the squid tentacle was measured to be 67° in a retracted tentacle and approximately 36° in a fully extended tentacle (Kier & Smith, 1985). Furthermore, the simultaneous contraction of both the left- and right-handed helical muscle systems can be exploited during prey handling in order to resist torsional forces (Kier & Smith, 1985).
A similar process occurs in the arms of squid and cuttlefish, wherein the contraction of right- and left-handed oblique muscle fiber layers and the associated crossed-fiber connective tissue sheets are responsible for generating torsional forces. However, these arms do not vary in length as tentacles do (Kier, 2016).
Tendrils: a comparison to tentacles
Tendrils are the coiling appendages that some plants use for structural support. One very common tendril is that of Phaseolus Vulgaris, or the common green bean. Tendrils are not tentacles; however, they share a similar function in that they can perform omnidirectional movement and elongation in space. In his book On the Movements and Habits of Climbing Plants, Charles Darwin (1865) said, “it first places its tendrils ready for action, as a polypus places its tentacula” (p. 118). He was referring to tendril-bearing plants, making one of the first comparisons between tendrils and tentacles. Indeed, observing the movements of tendrils, they appear to perform very similar functions to tentacles. However their structures differ greatly. These structural differences are what allow tendrils to perform like tentacles while existing in a terrestrial environment.
Tendrils are only a few millimeters wide and vary in length from 3.8 cm to 40 cm (Isnard & Silk, 2009). The difference in mass alone plays a big role in explaining why tendrils can thrive without being submerged in water like tentacles: it is simply that they feel less force from gravity. While tentacles lack any form of a traditional skeleton, tendrils are made of cells that have cell walls, which is the traditional skeleton of most plants. Tendrils are usually formed from stems or leaves, resulting in a similar structure. Like a tentacle, a tendril is shaped like a cylinder which tapers at the tip. Tendrils often have a bend at the end called a hook. The convex side of the hook is called the dorsal side and the concave side is called the ventral side (Jaffe & Galston, 1968).
There is another form of support that tendrils use to remain rigid, and this is osmotic pressure. The cells on the dorsal and ventral sides of bean tendrils were shown to have different osmotic potentials (Millet et al., 1984). Not only does osmotic pressure keep tendrils rigid, but it also allows them to circumnutate. Circumnutation is the typical movement that tendrils are known for. In fact, it is the helix rotation that allows tendrils to wrap around structures providing support for the plant. Cells on the dorsal side of the tendril have higher water content than those on the ventral side, but this water content is not constant. These cells are to some extent able to reversibly elongate, which allows the tendril to circumnutate (Caré et al., 1998).
Gelatinous fibers were studied in redvine tendrils and have been proposed as another way tendrils bend (Meloche et al., 2007). The composition of the gelatinous fibers allows them to hold water better than the other fibrous layers of the cell wall. This results in them having the ability to shrink and create tension. These gelatinous fibers in the cell walls were not produced in redvine tendrils that failed to coil. In later studies it has been shown that for tendrils that are able to coil in many directions there is a layer of gelatinous fibers surrounding the tendril. However, for tendrils that were only able to coil in one direction, gelatinous fibers existed exclusively on the ventral side (Bowling & Vaughn, 2009). Figure 9 and Figure 10 show light micrographs of two types of tendrils with different gelatinous fiber positioning.
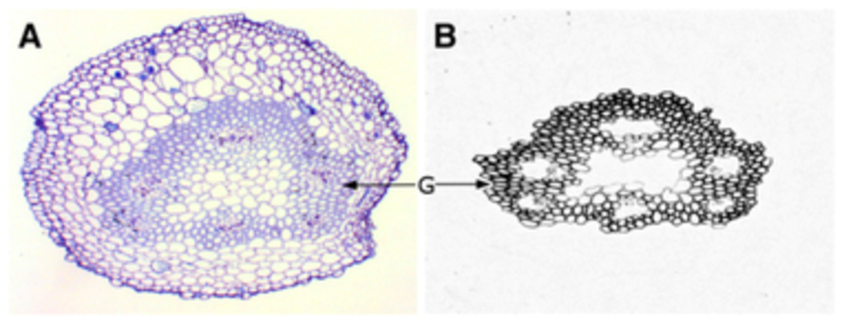
Fig. 9. A light micrograph of a redvine tendril showing the gelatinous fibers forming a ring around the center of the tendril (Bowling & Vaughn, 2009).
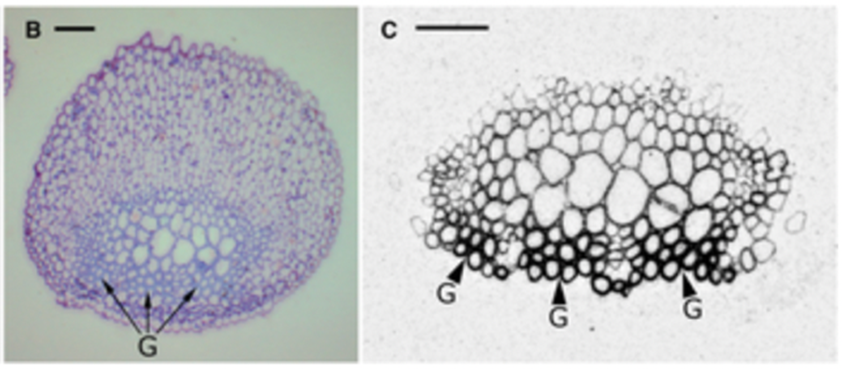
Fig. 10. This is a light micrograph of a balloon vine tendril showing gelatinous fibres on the coiling side (Bowling & Vaughn, 2009).
Overview of jellyfish anatomy
Jellyfish are one of the simplest multicellular organisms on the planet, yet they have such a fascinating design that allows them to swim and capture prey efficiently. A jellyfish consists of a bell, which is the umbrella shaped head, and tentacles/oral arms. Inside the bell, you will find the mouth, gut, and gonads, the latter being the reproductive organs. The tentacles, which hang from the bell as shown in Figure 11, are lined up with stinging cells called nematocysts that paralyze the prey. The oral arms, although different from jellyfish to jellyfish, usually hang from the mouth so that they can grab the paralyzed prey and lead it to the mouth. These arms are also lined up with nematocysts. Furthermore, the length of tentacles can vary amongst different jellyfish, where some might have tentacles that are shorter than the oral arms as shown in Figure 11, and other jellyfish might have them longer as seen in Figure 12.
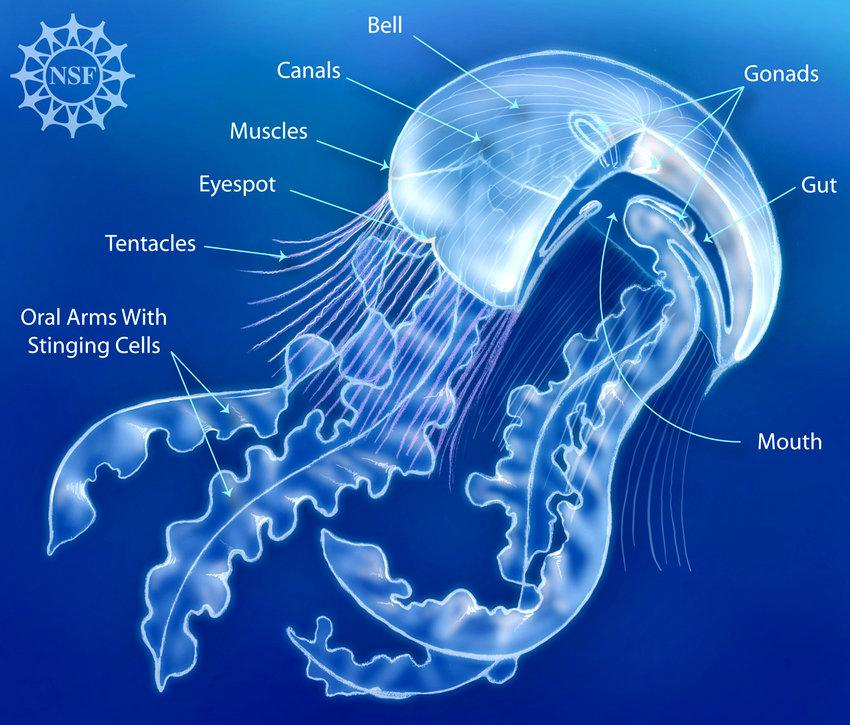
Fig. 11. A simple overview of the anatomy of a type of jellyfish in the Scyphozoa class (Courtesy of Deretsky, 2008).
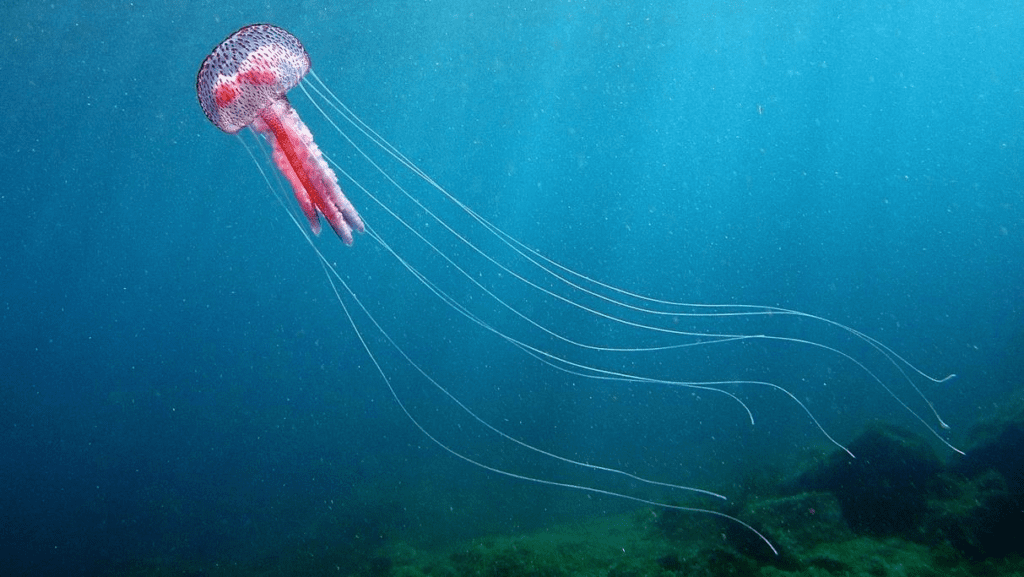
Fig. 12. The Pelagia Noctiluca jellyfish (Adapted from NATURSPORTS, DREAMSTIME).
Jellyfish tentacle structure
In most jellyfish, each tentacle branches out from a blade-like structure called the pedalium as shown in Figure 13. The pedalium contains the muscle fibers that allow the tentacle to reel back upwards toward the mouth when catching prey. The tentacle is composed of three layers shown in Figure 13: the epidermis (ectoderm), the mesoglea, and the gastrodermis (endoderm). The epidermis is lined up with nematocysts and cilia. The mesoglea contains muscles and collagen, which allows for contraction during predation (Stephanie & Richard, 2018). Finally, the gastrodermis contains densely packed vacuolated cells that help the jellyfish in feeding, protective response, and damage limitation (Gold et al., 2015).
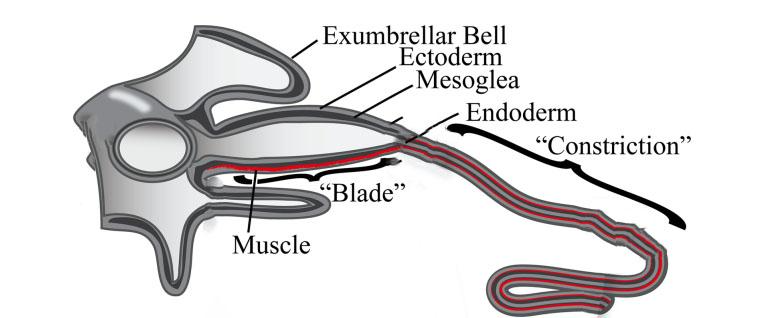
Fig. 13. The “Blade” or Pedalium narrows down to a tentacle, which consists of three layers: the Epidermis (Ectoderm), the Mesoglea, and the Endoderm (Gastrodermis) (Adapted from Structural and Developmental Disparity in the Tentacles of the Moon Jellyfish Aurelia, Gold et al., 2015).
How jellyfish tentacles affect swimming performance
Contrary to popular belief, jellyfish’s tentacles serve no purpose in a jellyfish’s locomotion. In fact, it has been found that the presence of tentacles slows down the jellyfish. According to a simulation done by Jason G. Miles and Nicholas A. Battista (2019), the more tentacles a jellyfish has, the shorter the distance covered per bell propulsion and the slower the swimming speed. In addition to this, the simulation also found that as the tentacles’ length increases, the swimming performance decreases. This is, however, only up to a certain length beyond which increasing the length does not significantly decrease swimming performance. The simulation also revealed that adding very short tentacles to the jellyfish has no effect on the swimming performance and equates to not having tentacles at all.
How jellyfish swim
To understand why tentacles are a drawback to jellyfish’s swimming performance, we first must understand how jellyfish swim. Jellyfish swim by pushing water against a “virtual wall,” which is created using vortices. When the jellyfish relaxes (opens up the bell), it creates a vortex at the edge of the bell, or the bell margin, that goes counterclockwise and underneath the bell as shown in Figure 14. When the jellyfish contracts, water along with the first vortex gets expelled, and at the same time, a new vortex forms at the bell margin that goes clockwise and goes downstream to meet the first vortex. When the two vortices meet, they create an area of high-water pressure, which acts as a wall. The water that is expelled from the bell behind the first vortex meets this wall and gives the jellyfish a thrust forward. At this point the jellyfish’s bell is relaxed once again and the cycle continues (Gemmell et al., 2020). Theoretically, the jellyfish could still move without creating the vortices using only Newton’s Third Law which states that every action has an equal and opposite reaction. The jellyfish creates an action which is expelling water from under the bell by contraction and this, in turn, will give the jellyfish a push in the opposite direction. However, the creation of the vortices creates a wall that allows the jellyfish to use much less energy for a bigger push, which makes it one of the most efficient swimmers in the ocean (Gemmell et al., 2013).
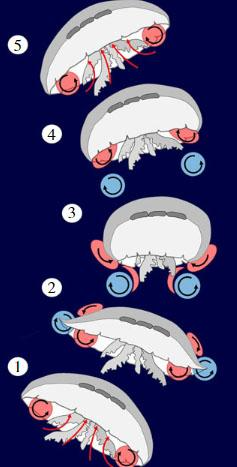
Fig. 14. (1) The bell is relaxed, and the first vortex is formed and trapped under the bell. (2) The bell starts to contract, and the second vortex starts to form. (3) When the bell fully contracts, the first and second vortices are expelled downstream and meet to create a virtual wall. (4) The bell begins to relax again and forms the first vortex. (5) The first vortex gets trapped under the bell and the cycle continues (Adapted from the most efficient metazoan swimmer creates a ‘virtual wall’ to enhance performance, Gemmell et al., 2020).
Why jellyfish tentacles affect swimming performance
Tentacles affect the jellyfish’s locomotion by slightly suppressing the vortices. When the vortices are expelled from the bell and bell margin, they bounce off the tentacles. As a result, rather than going directly downwards, the vortices travel laterally, which essentially creates a virtual wall with holes, making the push less effective. When the tentacles are very short, there is little to no surface area for the vortices to bounce off of, so very short tentacles do not affect the swimming performance. Similarly, when there are few tentacles, there are less surfaces for the vortices to bounce off of, and the more tentacles on the jellyfish, the higher the chance that the vortices will bounce and travel laterally. Likewise, when the tentacle’s length increases, it creates more surface area for the vortices to bounce from, but why does that effect subside after a certain length? This is because the vortices continue traveling downstream whether they bounce off or not, and eventually the vortex formation breaks because the momentum dies off or the vortices already meet before reaching the end of the tentacles (Miles & Battista, 2019).
Water physics
While tentacles are present in both aquatic and terrestrial organisms, these appendages have evolved to be particularly advantageous in an aquatic environment. Some of the properties of water must be discussed to understand why.
Viscosity is defined as the resistance of a fluid to a change in shape or movement and is quantified using units of Pascals ∙ seconds (Le Cam & Guilloret, n.d.). For water, this property is greatly dependent on temperature. In fact, its viscosity ranges from 1,79 × 10−3 Pa ∙ s at 0 °C to 0,653 × 10−3 Pa ∙ s at 40 °C (Le Cam & Guilloret, n.d.). A body moving through the water, therefore, experiences resistance due to the force required to displace water molecules.
Density is indeed another important property to consider. Defined as the ratio of the mass of a substance divided by the volume it occupies, a fluid with a greater density means that it possesses a greater inertia (Alexander, 2017; Le Cam & Guilloret, n.d.). Therefore, given that the density of water is 816 times greater than air at the same temperature and pressure (Le Cam & Guilloret, n.d.), water creates a greater resistance force, or drag, as an object moves through it (Alexander, 2017). This drag force Fd can be expressed with the following equation:

Here, Cd is the drag coefficient of the fluid, D is its density, A is the reference area of the body, and V is the velocity of the body relative to the fluid (Le Cam & Guilloret, n.d.). Therefore, when the velocity or reference area of an object increases, so does the resistance it experiences (Le Cam & Guilloret, n.d.). Additionally, because the density of water increases as its temperature declines, this gives rise to pressure changes which contribute to what is known as buoyancy.
Hydrostatic skeletons in squid tentacles have evolved to take advantage of the density and buoyancy of water. They contain no heavy structures like bones which allows them to rely on water for support, especially through the use of buoyancy forces that prevent them from sinking. Tentacle function, specifically elongation in prey capture, has evolved to minimize drag by moving in a linear forward motion (Van Leeuwen et al., 2000). Any bend in the tentacle during rapid elongation would increase the surface area facing the water and increase drag.
It would be difficult to compare the skeleton of a terrestrial organism to the musculature of squids because there are no close relatives that live on land. However, crab skeletons are of particular interest because there are both aquatic and terrestrial crabs. Crabs have a hydrostatic skeleton during molting, and it was shown that land-dwelling crabs have higher internal pressure and experience more stress within their skeleton (Taylor, 2018). Land crabs therefore experience more forces than aquatic crabs.
Conclusion
The specialized tentacle structure possessed by Cnidarians and Cephalopods has clearly evolved for the aquatic environment, and a similar structure would be impossible to utilize in a terrestrial environment. Water provides an external force to counteract gravity, allowing tentacles to evolve without a rigid skeleton. The hydrostatic skeleton of these structures that provides them with the right balance of rigidity and flexibility. One unique function exclusive to the aquatic environment is the rapid elongation of a squid’s tentacles when hunting, which could not be achieved if the tentacle had bones. Certain structures, such as the tentacles of jellyfish, have evolved in the aquatic environment and yet are a disadvantage to the organism due to drag. Tendrils in plants are functionally similar to tentacles; however, they contain more robust skeletons, which allow them to function on land. The animal kingdom is extremely complex and the variation within tentacle-bearing organisms is no exception. This paper has therefore attempted to outline the relationship between a tentacle’s structure and its environment.
References
Alexander, D. E. (2017). Chapter 3 – Fluid Biomechanics. In D. E. Alexander (Ed.), Nature’smachines: An introduction to organismal biomechanics (pp. 51-97). Academic Press. https://doi.org/10.1016/B978-0-12-804404-9.00003-7
Bowling, A. J., & Vaughn, K. C. (2009). Gelatinous fibers are widespread in coiling tendrils and twining vines [Article]. American Journal of Botany, 96(4), 719-727. https://doi.org/10.3732/ajb.0800373
Caré, A. F., Nefed’ev, L., Bonnet, B., Millet, B., & Badot, P. M. (1998). Cell elongation and revolving movement in Phaseolus vulgaris L. Twining shoots [Article]. Plant and Cell Physiology, 39(9), 914-921. https://doi.org/10.1093/oxfordjournals.pcp.a029454
Deretsky, Z. Jellyfish Anatomy, 2008. Available online: https://www.nsf.gov/news/mmg/mmg_disp.jsp? med_id=65101 (accessed on 29 September 2022).
Gemmell, B. J., Costello, J. H., Colin, S. P., Stewart, C. J., Dabiri, J. O., Tafti, D., & Priya, S. (2013). Passive energy recapture in jellyfish contributes to propulsive advantage over other metazoans. Proceedings of the National Academy of Sciences of the United States of America, 110(44), 17904–17909. https://doi.org/10.1073/pnas.1306983110
Gemmell, B. J., Du Clos, K. T., Colin, S. P., Sutherland, K. R., & Costello, J. H. (2020). The most efficient metazoan swimmer creates a ‘virtual wall’ to enhance performance. Proceedings. Biological sciences, 288(1942), 20202494. https://doi.org/10.1098/rspb.2020.2494
Giant squid anatomy [Online image]. Smithsonian. https://ocean.si.edu/ocean-life/invertebrates/giant-squid-anatomy
Gilly, W. F., Renken, C., Rosenthal, J. J. C., & Kier, W. M. (2020). Specialization for rapid
excitation in fast squid tentacle muscle involves action potentials absent in slow arm
muscle. Journal of Experimental Biology, 223(3), Article jeb218081.
https://doi.org/10.1242/jeb.218081
Gold D.A., Nakanishi N., Hensley N.M., Cozzolino K., Tabatabaee M., et al. (2015) Structural and Developmental Disparity in the Tentacles of the Moon Jellyfish Aurelia sp.1. 10(8): e0134741. https://doi.org/10.1371/journal.pone.0134741
Isnard, S., & Silk, W. K. (2009). Moving with climbing plants from Charles Darwin’s time into the 21st century [Review]. American Journal of Botany, 96(7), 1205-1221. https://doi.org/10.3732/ajb.0900045
Jaffe, M., & Galston, A. (1968). The physiology of tendrils. Annual Review of Plant Physiology, 19(1), 417-434.
Kier, W. M. (2012). The diversity of hydrostatic skeletons. Journal of Experimental Biology, 215(8), 1247-1257.
Kier, W. M. (1982). The functional morphology of the musculature of squid (Loliginidae) arms and tentacles [Article]. Journal of Morphology, 172(2), 179-192. https://doi.org/10.1002/jmor.1051720205
Kier, W. M. (2016). The musculature of coleoid cephalopod arms and tentacles. Frontiers in
Cell and Developmental Biology, 4(10). https://doi.org/10.3389/fcell.2016.00010
Kier, W. M. (1985). The musculature of squid arms and tentacles: Ultrastructural evidence for functional differences [Article]. Journal of Morphology, 185(2), 223-239. https://doi.org/10.1002/jmor.1051850208
Kier, W. M., & Smith, K. K. (1985). Tongues, tentacles and trunks: the biomechanics of
movement in muscular-hydrostats. Zoological Journal of the Linnean Society, 83(4),
307–324. https://doi.org/10.1111/j.1096-3642.1985.tb01178.x
Le Cam, R. & Guilloret, A. (n.d.). Les caractéristiques de l’eau. Apprendre à nager.
https://apprendre-a-nager.univ-rennes1.fr/natation/aller-plus-loin/les-caracteristiques-de-leau
Meloche, C. G., Knox, J. P., & Vaughn, K. C. (2007). A cortical band of gelatinous fibers causes the coiling of redvine tendrils: A model based upon cytochemical and immunocytochemical studies [Article]. Planta, 225(2), 485-498. https://doi.org/10.1007/s00425-006-0363-4
Miles J.G., Battista N.A. Naut Your Everyday Jellyfish Model: Exploring How Tentacles and Oral Arms Impact Locomotion. Fluids. 2019; 4(3):169. https://doi.org/10.3390/fluids4030169
Millet, B., Melin, D., Bonnet, B., Ibrahim, C. A., & Mercier, J. (1984). Rhythmic circumnutation movement of the shoots in phaseolus vulgaris l [Article]. Chronobiology International, 1(1), 11-19. https://doi.org/10.3109/07420528409059113
Simmons, S. L., & Satterlie, R. A. (2018). Tentacle musculature in the cubozoan jellyfish Carybdea marsupialis. The Biological bulletin, 235(2), 91–101. https://doi.org/10.1086/699325
Stavric, M., & Wiltsche, A. (2019). Geometrical elaboration of auxetic structures. Nexus Network Journal, 21(1), 79–90.
Stella, F., Obayashi, N., Santina, C. D., & Hughes, J. (2022) An experimental validation of thepolynomial curvature model: Identification and optimal control of a soft underwater tentacle. IEEE Robotics and Automated Letters, 7(4), 11410-11417.
Taylor, J. R. (2018). Aquatic versus terrestrial crab skeletal support: morphology, mechanics, molting and scaling. Journal of Experimental Biology, 221(21), jeb185421.
Van Leeuwen, J. L., De Groot, J. H., & Kier, W. M. (2000). Evolutionary mechanics of protrusible tentacles and tongues. Netherlands Journal of Zoology, 50(2), 113-140.