Mechanical and Material Design Principles of the Avian Egg Shell
Leticia Le Goff, Ula Mastej, Jake Pringle, Ryan Romero
Abstract
The design of the avian egg presents intricacies ranging from its mechanically durable architecture to its composition of bacterial protective materials. Characteristics such as the function, architecture, operation, and eggshell vulnerabilities are presented to outline the success of embryo survival and chick pipping. Protection against various external conditions and the regulation of gas and water exchange play a large role in determining shell strength and the survival of the embryo. Mineralization discussions as they relate to the shape and size of avian egg architecture then allow for a deeper explanation of shell strength. Regulation of required nutrients and gas exchanges with the wider environment all combine to determine the characteristics of the operation of the egg and ensure shell strength for an optimum development of the embryo. Finally, understanding the impact of hen nutrition and environmental factors allows for better food safety and increased protection of wildlife species.
Function
At the simplest level, the avian eggshell serves as a protective coat to the developing embryo until hatching can occur. Although the shell has considerably more functions, two of the arguably most important are protection and transport across the shell. Apart from protecting against mechanical impacts, the shell also offers protection against microbes and solar radiation. Additionally, the eggshell is responsible for regulating gas exchange and controlling water loss.
Protection
Mechanical Protection
One of the shell’s most important functions is to protect the egg’s content from mechanical impacts. The latter may generally be divided into two groups: impacts that result in crushing and those that result in cracking. Thus, the avian eggshell must be strong enough to withstand both of these impacts and support the mass of the developing embryo – all the while allowing hatching to occur (Board, 1982).
The two types of impacts – crushing and cracking – both play a large role in determining shell strength but in vastly different ways as the properties that prevent crushing do not necessarily prevent cracking. Firstly, for protection against crushing forces, the eggshell must be resistant to quasi-static loading, where forces are applied at such a slow enough rate that inertia becomes negligible (Board, 1982; Yavari, 2010). An example of quasi-static loading is brooding; shells must be able to easily support the weight of the parent without breaking. This leads researchers to theorize that “safety factors” are built into the shell which account for the extra weight added by the parents and prevent the eggs from being crushed (Board, 1982).
Next, cracking or fracturing is usually a result of shock loading, which is the opposite of quasi-static loading. In this case, the force exerted is a result of a sudden change in acceleration (Smith, “How to Calculate a Shock Load”). In shock loading, the force is applied to a specific point or small area and will result in a fracture along the shell. There are many causes of fractures, one of them being eggs hitting each other in the nest and, since nesting habits vary by species, some species have a higher chance of egg-to-egg collisions. For example, the domestic hen lays twelve eggs in an open nest, leading to a higher chance of collisions and consequently their shells need to be more resistant to shock loading (Board, 1982). Additionally, strength against shock loading is also needed to protect the egg from predators. Indeed, avian predators tend to break shells by repeatedly pecking one spot of the shell: this is an example of shock loading of a specific point. Sometimes, the egg’s shell is so strong that predators cannot break through solely by pecking and, in those cases, they might resort to dropping stones on the egg to break the shell. In particular, Egyptian vultures use this method to break the shells of the ostrich eggs (Board, 1982).
Thus, the avian eggshell protects the contents of the egg from mechanical impacts, both crushing forces from brooding and cracking forces from egg-to-egg collisions and predators. While shell strength is important for mechanical protection, a fractured shell is also more susceptible to bacteria. Hence, the shell also plays a vital role in bacterial protection.
Bacterial Protection
The shell’s pores are important for gas and water exchange, but microbes can also use them as a passage into the egg. This is where the topography and thickness of the cuticle, the topmost layer of the shell, come into play for they allow the cuticle to physically block bacteria from going through the pores. In fact, studies show that “increased roughness (Arnold and Bailey, 2000, as cited in D’Alba et al., “Antimicrobial properties of a nanostructured eggshell”) or nanostructural arrangements (Schumacher et al., 2007, as cited in D’Alba et al., “Antimicrobial properties of a nanostructured eggshell”), decrease bacterial attachment” and thicker cuticles are more effective at preventing bacteria from entering the egg (Chen et al., 2019; D’Alba et al., “Antimicrobial properties of a nanostructured eggshell”). Many species have cuticles formed by nanospheres of varying thicknesses. In particular, in the Australian brush turkey, the nanospheres forming the cuticle plug the shell pores, reducing the number of bacteria that can attach and penetrate into the egg (D’Alba et al., “What Does the Eggshell Cuticle Do?”).
Moreover, the nanospheres that form the cuticle are usually composed of calcium carbonate, but in some cases, such as in Australian brush turkey eggs, they can be made of calcium phosphate. Depending on the composition of the nanospheres, the cuticle layer will have different properties: calcium phosphate does not dissolve in water and is not digested by bacteria, which allows the cuticle to survive exposure to rain, mud, and high humidity – it is considered a superhydrophobic surface (D’Alba et al., “Antimicrobial properties of a nanostructured eggshell”). Hydrophobic cuticles, common in humid environments, prevent water and, by extension, bacterial attachment whereas hydrophilic cuticles are more common when nesting occurs in dry conditions (D’Alba et al., “What Does the Eggshell Cuticle Do?”). High hysteresis is also a common property of shell cuticles; this allows water droplets to remain “pinned to the surface and thus do not roll off” the shell (D’Alba et al., “Antimicrobial properties of a nanostructured eggshell”). Combining hydrophobicity with high hysteresis allows the cuticle to prevent trans-shell penetration more effectively, such as in Australian brush turkey eggs. In comparison, chicken eggs also have high hysteresis, but the cuticle is hydrophilic, meaning that water remains attached to the surface and will tend to spread across the shell, increasing the chances of penetration (D’Alba et al., “Antimicrobial properties of a nanostructured eggshell”).
As explained, the cuticle has the important function of limiting the amount of microbes forming colonies on the surface of the shell. In fact, studies have shown that removing the cuticle will significantly increase both the number of bacterial cells that attach to the eggshell and the risk of trans-shell penetration: “EDTA-treated shells were penetrated 2.5 times more often than control shells. Chicken eggshells were penetrated at higher rates than eggshells of any other species” (D’Alba et al., “What Does the Eggshell Cuticle Do?”). It is clear that the cuticle’s chemical and physical properties – that is its hydrophobicity, hysteresis and nanosphere structure – play an important role in protecting the developing embryo from bacteria.
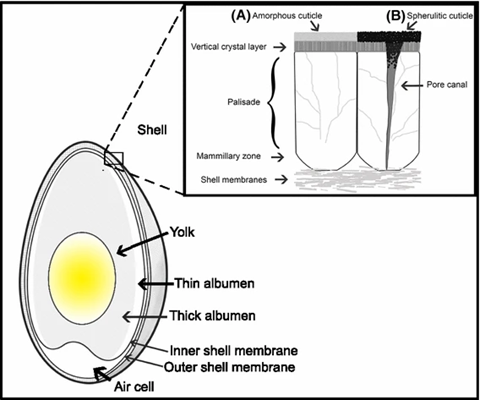
Solar and Ultraviolet Radiation
Finally, the shell protects the egg’s contents from radiation of varying wavelengths. As nesting conditions vary among species, some eggs are more likely to be subjected to high amounts of solar radiation, potentially leading to overheating. When the cuticle was experimentally removed, there was a noticeable increase in UV-chroma; this strongly suggests that one of the cuticle’s functions is to “[modulate] UV-reflectance of white eggshells” (Fecheyr-Lippens et al., 2015). For instance, in one study, cuticle removal showed a 5% increase in UV-chroma (D’Alba et al., “What Does the Eggshell Cuticle Do?”). In this particular work, all of the studied species have nanosphere cuticles and tend to be subjected to high levels of radiation. To account for this reflectance, the organic and inorganic compounds that compose the cuticle are thought to selectively absorb UV-wavelengths (Fecheyr-Lippens et al., 2015). By selectively absorbing certain wavelengths, the egg ensures that it does not overheat, which can otherwise be extremely damaging to the developing embryo.
Gas and Water Exchange
Gas Exchange
The eggshell is also responsible for controlling gas exchange needed for embryonic development through pores found in the shell. Pores are air channels that are formed as a result of incomplete fusion of adjacent crystals, and they function as a communication pathway between the outside environment and the inside of the egg (Toledo et al., 1982). Gas exchange through these pathways occurs by diffusion – CO2 and O2 pass through the pores in a process driven by the differing concentrations across the shell (Paganelli, 1980).
While the pores are the primary means through which gas exchange occurs, the cuticle plays an additional role in controlling this exchange. As previously mentioned, the cuticle can plug pores, which is useful in preventing water and bacteria from entering the cell. However, this can also impede gas flow. Interestingly, thinner cuticles decrease gas conductance more than thicker cuticles. This is due to the fact that thinner cuticles are composed of smaller spheres which are packed more closely together, forming clusters that are less permeable (D’Alba et al., “What Does the Eggshell Cuticle Do?”). Hence, the cuticle is vital in regulating gas exchange through the pores.
Water Regulation
Since the main purpose of the pores is the exchange of gases, their size is optimal for the exchange of O2 and CO2. However, an oxygen molecule is larger than a water molecule, meaning that the pores also allow water vapor to pass through the shell. Water evaporation is important to ensure that the amount of water in the egg remains constant throughout the whole incubation period and the pores allow for this to occur (Board, 1982).
At the same time, the egg must be able to regulate water conductance in order to prevent unnecessary evaporation. In particular, guillemots incubate their eggs by placing them under their webbed feet, rather than in a nest. This leads to a larger water vapor gradient and can cause excessive water loss, something that could be harmful to the embryo (D’Alba et al., “What Does the Eggshell Cuticle Do?”). For this reason, the cuticle has the vital role of regulating water exchange across the shell. In experiments, when the cuticle is removed, flooding of the egg through the pores is much easier, proving that the cuticle controls water conductance (Solomon, 2010). An example of this regulation is seen in the case of the Adélie penguin: they have evolved thick cuticles which reduces the rate of water evaporation and thus protects the embryo (D’Alba et al., “What Does the Eggshell Cuticle Do?”). Consequently, the cuticle and the shell pores work together in a similar fashion as in the case of gas exchange to control and regulate water evaporation conductance.
Architecture
It is crucial to understand the complexity of an avian egg’s architecture to then analyze properly its physical, ecological, and survival related properties. Doing so requires detailed explanation of both the structure and microstructure of an egg. The former will provide a comprehensive view of an eggshell’s physical properties with a focal point on shape and size as they relate to force dissipation. The latter allows for a breakdown of the crystal formations, organic protein matrix, and a multitude of other nano-properties of eggs that are to then be later complemented to the egg’s functions. Furthermore, exploring the microstructure of an eggshell’s architecture will highlight its active nature which will in turn reveal the fascination of shell pipping.
Structure
Governing Principles of Avian Egg Shape
Architecture specifies the plans and execution behind a given structure. It is for this reason that shape and its interrelation to mechanics is a suitable starting point for analysis of an avian egg’s structure and thereby architecture. Eggshell shapes vary incredibly across species. An observational study incorporating approximately 1 400 species produced the morphospace seen in Fig. 2. As shown, this morphospace encompasses shapes ranging from more elliptical and symmetric to more elliptical and asymmetric. From this, fundamental properties of avian egg architecture begin to surface. Namely, the claim on shell shape diversity is confirmed: although there is a noticeably dense region of the morphospace, across the 50 000 eggs used for the generation of the morphospace, there is a significant spectrum in shell shape (Stoddard et al., “Avian egg shape: Form, function, and evolution”). Such observed shape variance is inherent to the mechanics that makeup shell architecture.
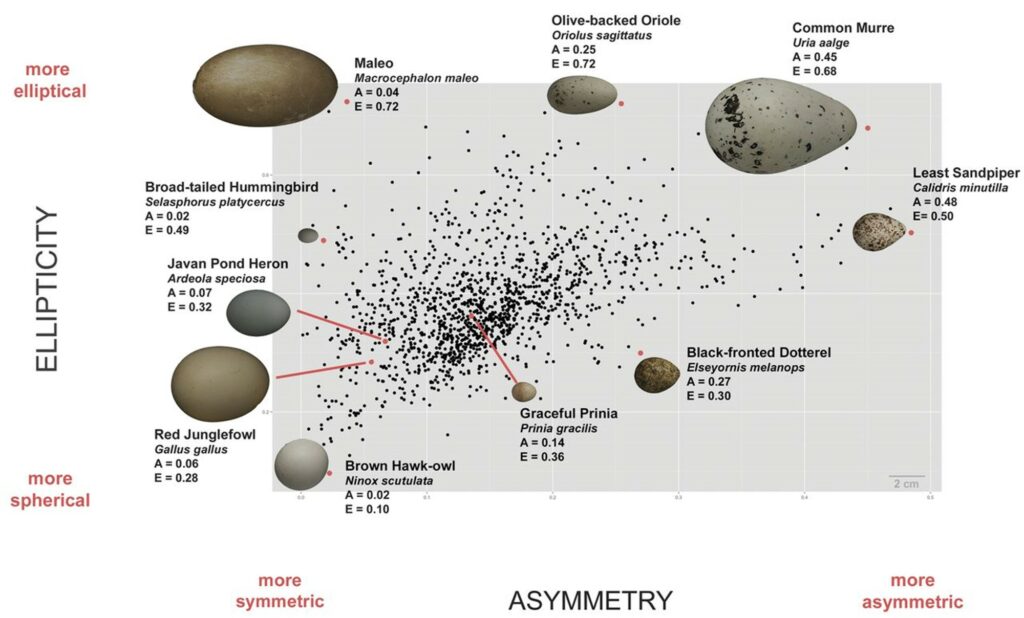
Specifically, recent research stresses the importance of looking at the pressure systems surrounding egg membranes to extrapolate predictions on shape. Eggshells encompass a membrane that has incredibly elastic properties, which paired with the eggshell’s ability for elastic distortion, courtesy of its calcite mineral composition, provide the primary basis for eggshell ellipticity of its architecture. Notably, compression simulations of eggshells have shown that a tremendous amount of an avian eggshell’s strength can be credited to the elastic properties of the architecture. In the case of point loads generated at avian egg poles, Young’s Modulus was determined for “spheroidal shell[s] under point load from a force-displacement curve” (Hahn et al., 2017). As an example, the average value for white-AA chicken eggs was 27.5GPa (Hahn et al., 2017). Fig. 3 outlines this exact phenomenon. As point loads increase, the greatest tensile stresses are actually observed at an angle below the pole. Thus, upon application of point loads to various regions of an egg, it was found that the axisymmetric nature of an egg’s architecture is such that crack propagations are limited (Hahn et al., 2017). As opposed to cracks being able to transmit through their axis of symmetry, circumferential cracks occur, allowing larger stresses to be placed on the shell’s architecture before crack deflection is no longer sound (Hahn et al., 2017). Such a mechanism allows for individual vertical cracks to occur along the shell before propagations align to create horizontal splitting of the shell. As well, local bending of the shell at the application region of loads increases the surface contact for pressures, resulting in “stress relief […] limiting the growth of initial cracks” (Hahn et al., 2017). Again, this exemplifies that the structure of an eggshell has developed in such a manner that the architecture best meets the function of protecting egg contents.
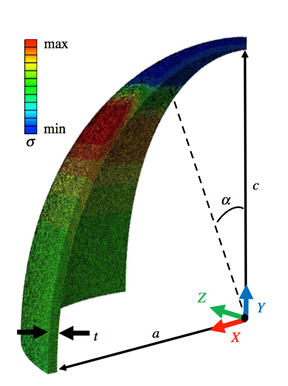
Based on variations in pressure applied to the eggshell surface – and therefore on the egg membrane – as well as imbalances in the membrane’s material composition, mathematical equations have been designed to mimic the architecture of the egg along axial and azimuthal directions. Through analysis of stress and strain ratios as well as the examination of the extensions of the egg membrane along the aforementioned directions as they relate to the curvilinear material, Eq. 1 and Eq. 2 below
μ(σ)={B(σ)\over A(σ)}
(1)
p(σ)={P\over (A(σ))}
(2)
indicate the mathematical model that allows to determine egg shape (Stoddard et al., “Avian egg shape: Form, function, and evolution”). Eq. 1 represents the ratio of the azimuthal stretching stiffnesses to the thickness-integrated axial stiffnesses and Eq. 2 et al., 2017). Overall, solving these mathematical relations relating to one another allows for governing of the egg’s asymmetry and ellipticity (Stoddard et al., “Avian egg shape: Form, function, and evolution”). Note also that these functions are spatially dependent as avian egg membranes are considered non-uniform (Stoddard et al., “Avian egg shape: Form, function, and evolution”). Although discussions of microstructures of membranes and shells are to ensue, it can be said that the membrane’s thickness, pressure, and other material properties vary across the entire egg. Whilst the egg remains axisymmetric, avian egg membranes are asymmetric between poles and thus maintain ranges of pressures throughout the egg yielding distortions in shape (Stoddard et al., “Avian egg shape: Form, function, and evolution”). Beyond this, further discussion of egg shape is tied to evolutionary hypotheses presented in later sections.
Size and Its Derivation from Force Toleration
Finally, similar techniques as shown above demonstrate shell thickness to be a stakeholder in the architecture that makes up the strength of avian eggs. It is a misconception to assume that the contents of variously sized eggs have an effect on robusticity; the commonly accepted theory is that an air sac exists inside of the egg and thus hollowed ellipsoid stress tests are a valid means to infer the effects of shell thickness on architectural strength. Using Weibull analysis, found moduli for hollowed avian eggs show that an eggshell can be considered a “technical ceramic” (Hahn et al., 2017). Under this categorization, stress tests performed along the major axis of an avian egg demonstrated that, as shell size increased, the force of failure subsequently increased. Statistical modelling across a sufficient sample size mirrored an r-squared value of 0.95 relating shell size to failure strength (Hahn et al., 2017). This is in part due to the larger surface contacts for force dissipation that occur as shell size increases, much like previously mentioned arguments when discussing elasticity. Moreover, it is noted that a thinner shell allows for a larger force of failure, again attesting to the properties of elasticity.
Microstructure
Architectural Composition of the Avian Eggshell
However, avian egg architecture is further rooted in its microstructure framework. Aforementioned details of structure are elaborated when considering the composition, orientation, and grain size of eggshells and membranes. As mentioned by Rodriguez-Navarro and colleagues, “the elastic and mechanical behavior of a ceramic in particular, depend strongly on its microstructure” (2002). Essentially, eggshells can be thought of as an architecture based on biomineralization, primarily of calcite. Much like other ‘skeletons’, avian eggshells are hierarchically organized. This factor is what contributes to the array of microstructures found across the mammillary, central palisades, and the vertical crystal layers of the shell (Rodriguez-Navarro et al., 2002). Particular to grain size, atomic force microscopy (AFM) attributed the hierarchical nature of eggshell architecture by revealing the Feret diameter increase of nanostructures from the outer vertical crystal layer to the inner mammillary layer (Rodriguez-Navarro et al., 2002). Then, observing the eggshell’s palisade layer from top to bottom, the maximum size of minerals was seen at the equator. This gradient in microstructure size then extends to account for 0.2 to 0.4 of the overall thickness, promoting above discussions that loads exerted on the egg were dissipated towards areas between the equator and poles for increased elasticity and stress toleration (Rodriguez-Navarro et al., 2002). However, the size and shape of minerals within the eggshell vary such dissipation and therefore have say in altering egg architecture. A prime example is quail eggs, whose cuticles making up their shell microstructure tend to be more curved than other nanostructures like those of the rounded chickens, guinea fowl, and geese. Such curved microstructures allow for increased “pre-existing crack nucleation sites” and, in turn, reduce the failure force sustained by quail eggs (Hahn et al., 2017). Consequently, attention to microstructure arrangements show the effect of crack propagation through avian eggshell architecture.
Orientation of Calcite Crystals
The development of eggshells explains the orientation of minerals within their architecture. Beginning with amorphous calcium carbonate accumulations on the egg membrane, intricate microstructures of calcite columns occur (Athanasiadou et al., 2018). Despite their columnar form, they have noticeably misaligned orientation throughout the shell’s layers. Electron backscatter diffraction identifies that a degree of randomness in calcite formation makes up eggshell architecture, successive to the varied blocking of the shell’s organic matrix (Rodriguez-Navarro et al., 2002). This is in contradiction to calcite found in ceramics previously used in stress testing who display far more precise scatter patterns (Rodriguez-Navarro et al., 2002). To explain this, light microscopy of the organic matrix making up the eggshell’s architecture found osteopontin (OPN) to be an abundant matrix protein (Athanasiadou et al., 2018). Although other fibrous proteins such as collagen make up membranes of avian eggs, contributing to its elastic properties, attention is drawn to OPN due to its effect on calcite formation. Indeed, its presence showed OPN to be a regulatory molecule for calcite mineralization (Athanasiadou et al., 2018). The random alignment of the eggshell’s microstructure is, to an extent, based on the relationship of the concentrations of OPN to mineralization. OPN experimentation with synthetic calcite formation proved such a relationship as the size of minerals varied by approximately 50 nanometers as concentration was ranged (Athanasiadou et al., 2018). As random orientation is stated to increase as calcite columns lengthen (Rodriguez-Navarro, 2002), the varying OPN concentrations account for altered inhibition of calcite growth, potentially accounting for the random mineral architecture of the shell (Athanasiadou et al., 2018).
Size of Minerals and the Impacts on Architectural Strength
From such oppositions, it is evident that columnar calcite misalignments are a necessary component for the function of eggshell architecture. Analysis provided by x-ray diffraction shows that calcite crystals prefer their planes to be parallel to the eggshell boundary, yet, as columns grow in width within the palisade layer and thickness moving outwards from the egg’s center, orientation becomes more scattered whilst texture turns smoother (Rodriguez-Navarro et al., 2002). This represents a dynamic architecture that ensures crack propagation from the exterior is as dampened as possible. The aforementioned definition of eggshell material being like that of ceramic indicates that cracking of an egg occurs from initiation at a critical point due to the brittle nature of calcite crystals (Hahn et al., 2017). By requiring cracks to navigate a random alignment of calcite minerals, “grain boundaries may prevent or hinder fracture” (Rodriguez-Navarro et al., 2002). Especially when calcite columns attain preferred orientation to the eggshell, cracks are even more hindered by such architecture as cleavage planes of crystals are parallel to the direction of propagation.
Operation
When terrestrial vertebrates began to colonize habitats out of water, the development of the amniotic egg was essential to ensure the protection and survival of the embryo (Stoddard et al., “Avian egg shape: Form, function, and evolution”). Of course, as stated before, one of the most important functions of the shell is to protect the chickling from the new external environment and also new environmental conditions whilst ensuring its growth. Understanding the key functions of the avian egg provides the basics needed to understand how the egg is formed at different stages and how the different steps to forming the shell affects the chickling. Finally, environmental conditions can also influence the operation of the shell and monitor the outcome of the egg.
Egg Growth Stages
The formation stages of the egg are primordial for the correct operation of the developing embryo. Essentially, the creation of the egg relies on having three protective layers: the shell and two underlying membranes, which are laid down in less than 24 hours as the egg cell passes down the oviduct of the hen into the uterus (Rahn et al., 1979). Over the 21 days of incubation, a typical egg weighing 60 g will need around 6 L of oxygen and give off 4.5 L of carbon dioxide and 11 L of water vapor. After 21 days, the egg will weigh 51 g due to water loss. The formation of the egg needs to provide an adequate structure for this process so that the shell is able to perform gas exchange with the outside, all the while keeping the necessary nutrients and oxygen requirements for the embryo.
Enclosure of the Yolk and Albumen
In the first place, an enclosed membrane is deposited around the sphere of the yolk, and also around the albumen. The yolk contains lipids and proteins required for embryonic growth, whilst the albumen mainly contains water and proteins which have specific roles in maintaining the viscosity of the thick albumen and protecting the yolk from attacks by microorganisms (“The Avian Egg” | Poultry Hub Australia, 2017). The albumen is made up of 90 % water and 10 % proteins, and, in consequence, the albumen is the primary source of water for the embryo (Willems et al., 2014). Water is crucial for the embryo because during its incubation, the egg needs to lose 12-14 % of its water content in order to create an air unit for the lung respiration process to begin. At the same time, not enough water means dehydration of the embryo and so the perfect level of water must be met in order for the bird’s survival.
Internal Pressures in the Isthmus Oviduct and Shell Gland
After having an enclosed membrane over the yolk and the albumen, the future egg moves through the final parts of the oviduct. There, an internal pressure is created onto the pouch encased by the membrane, which arises from the fluids being actively pumped into the pouch, differential elasticity of the isthmus – the last part of the oviduct – wall and muscular contractions from the mother. The combination of these factors establishes the egg shape, but it is above all in the isthmus where the egg receives an additional membrane deposition that hardens the overall egg and determines the elongated and asymmetrical shape of the egg. After leaving the isthmus, the egg enters the shell gland where a biomineralized calcium carbonate shell is deposited and indicates the end of the shell formation. The provenance of this calcium surprisingly comes from the breakdown of the medullary bone of the hen. In less than a day, a hen mobilizes 10 % of her total bone substance which will be destroyed and contribute to the shell formation process of the egg (Taylor, 1970). Acquiring a strong shell that still manages to allow passage of air and water vapor determines the survival of the embryo since it needs protection from the environment all the while having an exchange of oxygen, carbon dioxide and water vapor.
Membrane Properties and Consequences on Shell
Variations in membrane material properties such as the fiber composition, the thickness of the membrane and the general elasticity lead to distortion forces in different directions and as a result to different egg structures and shapes. However, having a different shape or size does not mean a different basic operation process is needed for the egg which still needs to breathe and provide the required nutrients to the developing embryo. It is important to say that the inner membrane represents the first barrier the chickling has to break through during hatching. This membrane is proven to be elastic, making it possible to contain the albumen and yolk, but as soon as the chickling is ready to start the lung respiration process, the membrane is easily broken with the beak of the chickling and thus reaches the air unit of the egg. One of the key factors from which the shape of the egg can diversify itself lies with the different membrane properties, but this does not affect the operation of the egg.
Environmental Impact on Operation
Although egg formation mainly focalizes on the biophysical aspect, the life history of the organism and the development of the embryo inside the shell, it is also essential to point out the important influence the abiotic environment has on an egg. The abiotic environment includes a wide range of factors that may be unfavorable and detrimental to the eggshell and therefore to the development of the embryo. In this case, studies have shown that the operation of an eggshell can vary because of environmental conditions to better deal with harsh temperatures, direct sunlight exposure and arid climates.
Environmental Impacts on the Shell Conductance and Pore Geometry Density
Predominantly, when thinking about influence from environmental conditions on the egg, studies tend to pay attention to the influence it can have on the shape of the egg. Indeed, we find a wide range of shapes in eggs with some being more elongated and elliptical whilst others are more spherical and orbicular. But do these shapes have a direct link with external factors such as temperature and atmospheric conditions? Essentially, the egg needs to be optimized to face the environmental conditions in which it is located. This suggests that certain characteristics of the egg, including its shape, must be altered to further increase its prospects of survival in the specific environment. Studies have shown that eggs found in deserts, in high altitudes or in high temperatures have relatively lower shell conductance (Duursma et al., “Variation in avian egg shape and nest structure”). When speaking of shell conductance, it is convenient to also mention pore geometry as they correlate with each other. Both are essential aspects of the shell as they represent the only contact with the external world and therefore provide oxygen to the embryo. Every nutrient that is not available to the embryo inside the membrane of the eggshell comes from the external environment and passes through these pores. When an egg has more exchanges, the shell conductance is said to be higher. In more general terms, these characteristics allow the egg to “breath” by diffusion through thousands of microscopic pores in the shell (Rokitka and Rahn, 1987).
Remarkably, the density and the amount of these traits can affect the egg shape and hatchability but in a reverse manner, the egg shape also influences the shell conductance. So size, shape and shell conductance coincide to build and create the perfect habitat for the embryo’s growth inside the egg.
The determination of the dimensions and the number of pores is established in the uterus before the egg is laid and then remains unchanged. Recognizing that eggshells in different environments have different shell conductance levels reveals the intricate work of evolution on eggshell operation. Notably, shell conductance and pore density vary across an egg due to its geometrical shape and so the blunt end of an egg always has a greater conductance value than the rest of the surface of the egg (Rokitka and Rahn, 1987). Eggshell conductance is determined by the structure’s components which are in turn influenced by the functionality of the eggshell but also by the size of the egg. As the size of the egg tends to be larger, so does the eggshell conductance (Shafey, 2016). Thus, high eggshell conductance for large eggs will probably initiate a loss of extra water but will allow additional gas exchanges since the conductance is more important. Consequently, having a lower shell conductance limits the water loss all the while permitting the shell to perform gas exchanges for the embryo.
During its maturation, an egg must lose about 12 % of its initial weight as water vapor (Shafey, 2016), so gas exchanges with the outside are fundamental for the survival of the embryo. Nevertheless, they may lead to harmful situations if they are not adequately controlled. For that reason, the determination of these characteristics concerning the shell conductance but also the pore geometry is key to the embryo survival. In addition to this, pore geometries also vary with external conditions and studies noted that when shell conductance decreased, so did the pore geometries to limit the exchange surface area with external ecosystems. As a result, shell conductance tends to be lower for eggs exposed to high temperatures and found in arid climates compared to eggs subject to more humid weather conditions and climates that had higher conductance (Shafey, 2016).
Temperatures as an Environmental Factor
When speaking of environmental factors, this suggests an environment with extreme climatic conditions related to the temperature, which increases the drying capacity of the air and therefore can have significant repercussions on the gas exchanges and water loss of the avian egg. Taking a closer look at ambient temperatures, studies have shown that egg shapes widely vary depending on the climate they find themselves in. Using eggs from avian birds in Australia, where the most extreme climates exist on earth, it has been noticed that egg elongation decreases in these hotter and drier areas (Heenan, 2013). This characteristic can be explained by comparing the weather Australia has and what that means for the shell operation are. Having a dry and arid environment means that water loss needs to be limited as much as possible so to not lead to the dehydration of the embryo. By this, with the reduction of the elongation, there is less surface area of the shell which means there is less density of pore geometry which ultimately reduces the possibility of evaporation and keeps essential nutrients inside the shell.
Having a temperature that is too elevated may lead to an unhealthy embryo with reduced chances of survival due to dehydration. Hence, it is of utmost importance that, for a perfect development, the egg must find an environment with a relatively small temperature range so that it does not need to keep adapting to the ever-changing temperatures. Nonetheless, environmental factors are also related to the vegetation available meaning how much shade is available and whether this factor can diminish direct sunlight exposure for the egg. These aspects show how complex but also crucial finding the perfect environment is for the development of the egg (Heenan, 2013).
Influence of the Nest as an Environmental Factor
Nest building is a shared behavioral characteristic in the avian species, consisting of a construction of a burrow with key architectural designs, depending on the species and on the environment, in which birds lay eggs and raise their offspring. Seeing how nests are fundamental for the development of the chicklings, it is also very important to notice that nests are also fundamental for the embryogenesis stage of the chickling (Mainwaring et al., 2014). Of course, avoiding predation is an omnipresent concern for birds when building their nests, but other factors such as the temperature range, the humidity levels and the architecture of the structure are also just as likely to determine the location of the nest. Studies have shown that the design of a nest greatly influences the microclimate generated within the nest cup which is then experienced by the parents and offspring (Mainwaring et al., 2014). Overall, the geographical distribution of the egg shape and the nest type should reflect the selective pressures of key environmental parameters that influence the development of the egg. Those parameters are, for example, the ambient temperature and the drying air capacity which, in turn, alter the humidity and the water loss levels of the egg. Size, structure and composition of the nest all contribute to creating a microclimate for the egg, thus determining the degree of exposure the egg will receive from the wider environment (Heenan, 2013). Referring to avian eggs located in Australia, it has been pointed out that species with domed roof nests had more progeny in these hotter and drier areas (Heenan, 2013). The reason for this choice of architecture is that having a domed roof nest provides not only better hiding and better protection from predators, but it mostly provides shade and limits sun UV radiation, that can penetrate the shell and be harmful for the embryo, in an environment with insufficient plant coverage (Heenan, 2013).
In general, nests that are enclosed – cavity, burrow, domed structure with roof – are beneficial to species living in arid and hot regions as they are more favorable for keeping a desirable temperature and ideal levels of humidity (Heenan, 2013). We can think of these types of nests as larger incubators in which optimal conditions for development are maintained throughout. Taking the example of nests in hot and arid regions, having an enclosed nest would mean having a better control of the conditions inside the nest. This would mean being able to regulate the temperature so as to not risk exposing the egg to an increase in water loss. This is an example which indicates that having a defined nest architecture also determines how the egg will react in that environment. Parameters like the temperature, the altitude, the materials used in the nest are crucial since they act on the operation of the egg and the survival of the embryo. In the end, 33.7 % of passerines on the Australian continent build dome-shaped nests rather than cup-shaped nests which is a slightly higher percentage than anywhere else in the world. Moreover, worldwide there are only 28.5 % of all nest-building families that construct domed nests compared to 71.5 % for cup-shaped nests (Price and Griffith, 2017). Thus, it is possible to expect domed shaped nests and rounder shaped eggs to be more commonly found in hot and arid environments because of their impact on the operation of the egg.
Since the egg is a fixed structure with a defined function and limited operational changes, environmental conditions which are ever changing represent one of the egg’s limits since it cannot adapt itself freely to bear the violent surroundings of the ecosystem it is laid in. This is why being in the best environment is crucial for the growth of the egg and knowing how an egg can make adjustments to help its development in these climate conditions will better its chances of survival. In more general terms, we can say that environmental conditions influence certain combinations of nest types and egg shape because, when combined, they create a beneficial microenvironment for the egg.
Vulnerabilities of Avian Eggshell Structure
One of the greatest issues with eggshell structure affecting avian species is eggshell thinning. This change to the structure of the egg directly impacts the ability of the eggshell to fulfill its main function of protecting the bird embryo, most significantly from physical impact from the exterior. Eggshell thinning poses a major risk to wild birds as it makes it easier for the eggshell to break, and thus more difficult for young birds to survive to the pipping stage. Additionally, it also poses issues in the chicken egg industry, where 10-20 % of chicken eggs crack therefore allowing the contamination of eggs by diseases such as salmonella (McKee, 2018). The identification of what causes eggshell thinning and how nature or humans may aid in fixing it is thus a major concern for both ensuring the survival of wild avian species and food safety of the human diet.
Dietary Impact and Supplementation
A large contributor to thinning eggshells, most noticeable in the chicken egg industry, is the low calcium diet of the hen. During shell formation, there are typically high levels of calcium in a hen’s bloodstream, up to 30 mg/dL, with calcium levels being lower during non-shell forming days (Joyner, 1999). In a study done on Single Comb White Leghorn hens, it was found that, with increased calcium intake, calcium retention per gram increased in hens. Furthermore, the study concluded that calcium consumption was correlated with greater shell strength, linearly correlated with calcium retention, and quadratically correlated with increased shell weight and grams of shell calcium. However, this relationship breaks down at high calcium intake levels, namely 4.5 % calcium in diet (Clunies et al., “Calcium and Phosphorus Metabolism and Eggshell Formation of Hens”). It follows that by increasing calcium in the diet of hens, businesses are able to cause thickening of eggshells when hen eggs are too thin. Thus, they can prevent breakage, and so ensure better food safety and that more eggs appropriate for sale are produced.
It is important to note that other large factors in considering calcium retention in hens are phosphorus and estrogen levels, and so simply raising calcium percentage in diet will not necessarily always provide the anticipated results. Estrogen levels are correlated with the production of more blood calcium binding proteins, allowing for higher calcium retention rates. Nevertheless, when the egg is in utero, hens can deplete their entire blood calcium in 8 to 18 minutes and so intestinal absorption and bone mobilization of calcium are also needed to replenish blood calcium (Joyner, 1999). Since calcium is also taken from bone when blood calcium levels are low, it is important to build strong bones in laying birds, for which phosphorus is essential. Ideal ratios of calcium to phosphorus have been developed for various types of birds. For example, psittacine hens show a 2:1 ratio (Joyner, 1999). Furthermore, these ratios tend to be higher when shell production is occurring, as more calcium is going towards shell formation than bone formation of the hen (Clunies et al., “Calcium and Phosphorus Metabolism and Eggshell Thickness in Laying Hens”). Thus, if supplementation is needed to strengthen eggshell thickness, not only calcium should be considered but also phosphorus and estrogen supplementation.
Impact of Changing Climate
In various studies, a correlation has been found between rising temperatures and decreasing eggshell thickness. It is important to note that this is due not to lack of dietary calcium in the diet or body reserves, but rather the effects of higher temperature on the hen’s internal environment. It is most likely due to rising pH in the blood of the hen. This alkalosis occurs as a result of high-temperature-induced frequent panting, which removes carbon dioxide (or carbonate) from the bloodstream (Smith, “Changes in the average weight and shell thickness of eggs”). Interestingly, Frank and Burger have found that chronic exposure to regimes designed to increase carbon dioxide/carbonate levels in the blood result in thicker eggshells, regardless of what the effect is on pH (Frank and Burger, 1965). Although the exact reason for which shell thickness decreases due to temperatures is largely open to debate, a relationship has been observed between eggshell weight and temperature. In White Leghorn pullets, it is as follows (Smith, “Changes in the average weight and shell thickness of eggs”):
W = 79.880 - 0.421 (0.2T - 16) - 1.029 (0.2T - 16)^2
(3)
where W is shell weight in mg/cm3 and T is environmental temperature. This suggests a temperature range within which eggshell weight is optimized for every given species. Global warming is bound to shift the average global temperature away from ideal temperatures for eggshell production for several species of birds, thus causing thinner eggs more prone to breakage.
In observing the effect of temperature on eggshell thickness, it is imperative to observe the effect on the microscopic structure of the eggshell itself to fully understand its effect. The spongy matrix and mammillary layer of the shell are where most minerals are deposited, including magnesium and calcium, both of which combine with carbonates. In normal conditions, the calcified shells are composed of 2/3 of a spongy layer. Nonetheless, at high temperatures of 29.5 oC and humidity 75 – 80 %, eggs have much thinner spongy layers and disturbed columnar structure (El-Boushy, 1966). These changes result in a weaker shell structure at the microscopic level, making the shell more prone to breakage.
Coupled with reduced eggshell thickness, another effect of changing climate – namely humidity – causes changes to the ability of eggshells to create a proper microclimate for the embryo. One of the main functions of the eggshell is to regulate gas exchange, allowing for slow loss of water vapor within the shell during the process of incubation. At increasing temperatures, and lowering humidity, water vapor loss will occur more quickly and the chick will dehydrate. This effect is further magnified when eggshells are thinner (Veldsman et al., 2019). If humidity is higher outside, water vapor loss does not occur as much and it becomes more difficult for chicks to fill their lungs fully upon piping (Noiva et al., 2014). Thus, changes in humidity interfere with an eggshell’s ability to provide the correct microclimate for the egg and thinner shells further exacerbate this issue.
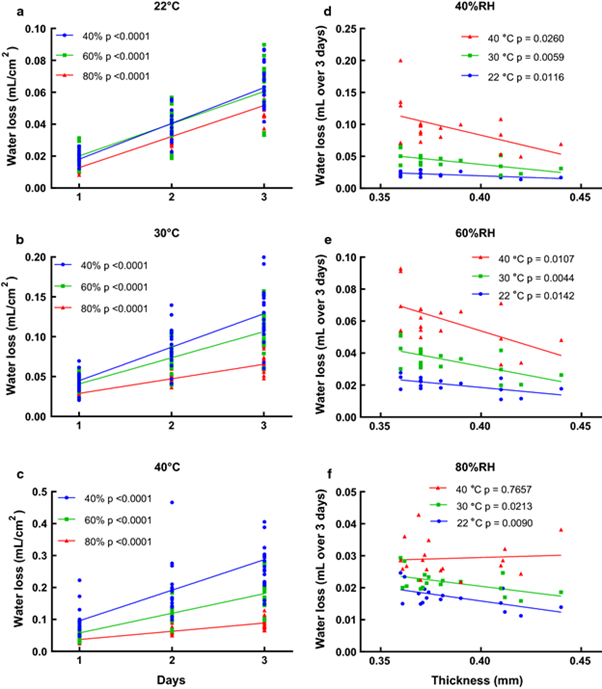
Correcting for the issue of a changing climate is not done easily. In the chicken egg industry, factory owners have direct control over temperature and humidity and so they can adequately provide an optimal environment for eggshells to properly form and fulfill their function. This cannot be done for wild birds. However, there have already been some observed changes in wild avian species’ behaviors that indicate an adaptation to the new climate. For instance, red-backed shrikes in Poland arrive at breeding grounds earlier to be able to lay eggs in more optimal conditions (Tryjanowski et al., 2004). In Australia, many bird species were found to lay eggs when vegetation and food resources were adequate and not necessarily at a given time of year (Duursma et al., “Variation in the timing of avian egg‐laying”). This research suggests that an adaptive and opportunistic approach is used to determine breeding time and so many avian species will be able to compensate for some of the climate changes by choosing another time to breed.
Impact of Environmental Pollution: Chemicals
Environmental pollutants are also known to interfere with the architecture of an eggshell, causing it to be thinner. Pollutants typically have a greater effect on birds of prey, due to bioaccumulation in their diets. Common pollutants that cause shell thinning include organochlorines often found in pesticides. The exact pollutant by which shell thinning is caused is determined by the environment, the species and what pesticides are common in a given area. DDEs and other members of the DDT group of insecticides have caused damage in North America, whereas cyclodienes have caused more damage in Britain (Cooke, 1973). Thus, exact impact is determined by individual species.
DDEs’ main impact appears to be on shell thinning and porosity. Burton. F.G. et al. have found that grey heron eggs with high contents of DDE tend to be more porous than those with low DDE content. Furthermore, the eggs high in DDE tend to have 3 – 10 % more water loss than the average grey heron egg. Interestingly, ducks and kestrels fed DDT had thinner eggs, but not more porous ones (Burton et al., 1986). The precise impact of DDT and related compounds is therefore not clear and appears to be species dependent. Generally, it can be said to interfere with the protective function of the eggshell, making it easier to break and sometimes interfere with gas exchange modulation.
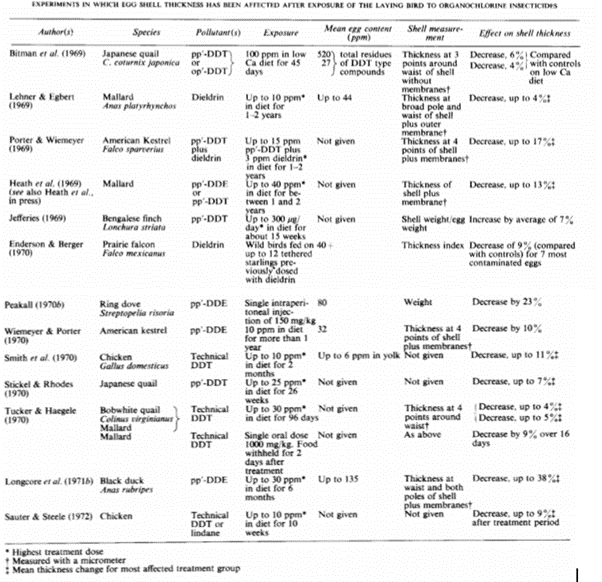
Resolving the problem of pesticide impact on birds of prey is largely in the hands of regulating their use. Lowering the amount of pesticide use allows for less accumulation in the diet of birds of prey. However, if a species has been severely impacted, more human intervention is required to ensure its survival. It may be required to breed avian species in captivity temporarily and provide supplementation in the form of calcium, phosphorus, estrogen, vitamin D and probiotics to increase shell thickness. Following the ban of DDT in 1972 in the United States, much effort has been put specifically into saving the bald eagle using these methods, resulting in a return of the species to the wild and removal of the endangerment title in 2007 (“Bald Eagle Fact Sheet” | U.S. Fish and Wildlife Service, 2019). Thus, there is reasonable hope that human intervention can aid in ensuring the survival of all falcons, eagles, pelicans and other avian species impacted by environmental pollution.
Conclusion
As explained by the function, the architecture, the operation and the limits of the egg structure, the morphospace and the characteristics of the shell determine the strength of the egg. In part, the eggshell composition controls the shell thickness, the degree of shell conductance and the shell deformation which, in turn, all act on the resistance of the shell in comparison to external forces. Nonetheless, ensuring the shell is capable of protecting the embryo by including a link with the wider environment through pores in the microstructure and being able to adapt the egg to increase the chances of survival in a harsh environment, also translates to weakening the structure of the shell to provide the embryo protection and the required nutrients for development. Thus, during the progression of the incubation, the shell weakens which then allows the chickling to break the structure easily and begin hatching. Being able to determine these properties on the eggshell structure can help reduce the impact humans have on egg reproduction and improve food safety concerning eggs.
References
Arnold, J. W., & Bailey, G. W. (2000). Surface finishes on stainless steel reduce bacterial attachment and early biofilm formation: scanning electron and atomic force microscopy study. Poultry Science, 79(12), 1839-1845. doi:10.1093/ps/79.12.1839
Athanasiadou, D., Jiang, W., Goldbaum, D., Saleem, A., Basu, K., Pacella, M. S., . . . McKee, M. D. (2018). Nanostructure, osteopontin, and mechanical properties of calcitic avian eggshell. Science Advances, 4(3), eaar3219. Retrieved from https://advances.sciencemag.org/content/advances/4/3/eaar3219.full.pdf
Australia, P. H. (2017, 2021). The Avian Egg. Retrieved from https://www.poultryhub.org/anatomy-and-physiology/the-avian-egg
BOARD, R. G. (1982). PROPERTIES OF AVIAN EGG SHELLS AND THEIR ADAPTIVE VALUE. Biological Reviews, 57(1), 1-28. doi:https://doi.org/10.1111/j.1469-185X.1982.tb00362.x
Burton, F. G., Marquiss, M., & Tullett, S. G. (1986). A note on eggshell porosity, nest humidity and the effects of DDE in the grey heron (Ardea cinerea). Comp Biochem Physiol C Comp Pharmacol Toxicol, 85(1), 25-31. doi:10.1016/0742-8413(86)90047-2
Chen, X., Li, X., Guo, Y., Li, W., Song, J., Xu, G., . . . Zheng, J. (2019). Impact of cuticle quality and eggshell thickness on egg antibacterial efficiency. Poultry Science, 98(2), 940-948. doi:10.3382/ps/pey369
Clunies, M., Parks, D., & Leeson, S. (1992a). Calcium and Phosphorus Metabolism and Eggshell Formation of Hens Fed Different Amounts of Calcium. Poultry Science, 71(3), 482-489. doi:10.3382/ps.0710482
Clunies, M., Parks, D., & Leeson, S. (1992b). Calcium and Phosphorus Metabolism and Eggshell Thickness in Laying Hens Producing Thick or Thin Shells. Poultry Science, 71(3), 490-498. doi:10.3382/ps.0710490
Cooke, A. S. (1973). Shell thinning in avian eggs by environmental pollutants. Environmental Pollution (1970), 4(2), 85-152. doi:10.1016/0013-9327(73)90009-8
D’Alba, L., Jones, D. N., Badawy, H. T., Eliason, C. M., & Shawkey, M. D. (2014). Antimicrobial properties of a nanostructured eggshell from a compost-nesting bird. Journal of Experimental Biology, 217(7), 1116-1121. doi:10.1242/jeb.098343
D’Alba, L., Torres, R., Waterhouse, G. I. N., Eliason, C., Hauber, M. E., & Shawkey, M. D. (2017). What Does the Eggshell Cuticle Do? A Functional Comparison of Avian Eggshell Cuticles. Physiol Biochem Zool, 90(5), 588-599. doi:10.1086/693434
D’Alba, L., & Shawkey, M. D. (2015). Mechanisms of antimicrobial defense in avian eggs. Journal of Ornithology, 156(1), 399-408. doi:10.1007/s10336-015-1226-1
Duursma, D., Gallagher, R., & Griffith, S. (2018). Variation in the timing of avian egg‐laying in relation to climate. Ecography, 42. doi:10.1111/ecog.03602
Duursma, D., Gallagher, R. V., Price, J. J., & Griffith, S. C. (2018). Variation in avian egg shape and nest structure is explained by climatic conditions. Scientific Reports, 8(1), 4141. doi:10.1038/s41598-018-22436-0
El-Boushy, A. R. (1966). Egg shell quality and microstructure as affected by vitamin C, other feed additives and high environmental temperatures. (PhD). Wageningen University & Research, Wageningen. Retrieved from https://edepot.wur.nl/187947
Elblbesy, M. A. (2018). Microscopic Monitoring of Erythrocytes Deformation under Different Shear Stresses Using Computerized Cone and Plate Flow Chamber: Analytical Study of Normal Erythrocytes and Iron Deficiency Anemia. BioMed Research International, 2018, 6067583. doi:10.1155/2018/6067583
Fecheyr-Lippens, D. C., Igic, B., D’Alba, L., Hanley, D., Verdes, A., Holford, M., . . . Shawkey, M. D. (2015). The cuticle modulates ultraviolet reflectance of avian eggshells. Biology Open, 4(7), 753-759. doi:10.1242/bio.012211
Frank, F. R., & Burger, R. E. (1965). The Effect of Carbon Dioxide Inhalation and Sodium Bicarbonate Ingestion on Egg Shell Deposition. Poultry Science, 44(6), 1604-1606. doi:10.3382/ps.0441604
Hahn, E. N., Sherman, V. R., Pissarenko, A., Rohrbach, S. D., Fernandes, D. J., & Meyers, M. A. (2017). Nature’s technical ceramic: the avian eggshell. Journal of The Royal Society Interface, 14(126), 20160804. doi:doi:10.1098/rsif.2016.0804
Heenan, C. B. (2013). An Overview of the Factors Influencing the Morphology and Thermal Properties of Avian Nests. Avian Biology Research, 6(2), 104-118. doi:10.3184/003685013×13614670646299
Joyner, K. L. (1999). Theriogenology In Avian medicine: principles and application (pp. 748-804): Wingers Publishing, Inc.
Mainwaring, M. C., Hartley, I. R., Lambrechts, M. M., & Deeming, D. C. (2014). The design and function of birds’ nests. Ecology and Evolution, 4(20), 3909-3928. doi:https://doi.org/10.1002/ece3.1054
McKee, M. (2018). Cracking eggshell nanostructure. Retrieved from https://www.mcgill.ca/channels/channels/news/cracking-eggshell-nanostructure-286264
Noiva, R. M., Menezes, A. C., & Peleteiro, M. C. (2014). Influence of temperature and humidity manipulation on chicken embryonic development. BMC Veterinary Research, 10, 234. doi:10.1186/s12917-014-0234-3
PAGANELLI, C. V. (2015). The Physics of Gas Exchange Across the Avian Eggshell1. American Zoologist, 20(2), 329-338. doi:10.1093/icb/20.2.329
Price, J. J., & Griffith, S. C. (2017). Open cup nests evolved from roofed nests in the early passerines. Proceedings of the Royal Society B: Biological Sciences, 284(1848), 20162708. doi:doi:10.1098/rspb.2016.2708
Rahn, H., Ar, A., & Paganelli, C. V. (1979). How Bird Eggs Breathe. Scientific American, 240(2), 46-55. Retrieved from http://www.jstor.org/stable/24965119
Rodriguez-Navarro, A., Kalin, O., Nys, Y., & Garcia-Ruiz, J. M. (2002). Influence of the microstructure on the shell strength of eggs laid by hens of different ages. British Poultry Science, 43(3), 395-403. doi:10.1080/00071660120103675
Rokitka, M. A., & Rahn, H. (1987). Regional differences in shell conductance and pore density of avian eggs. Respiration Physiology, 68(3), 371-376. doi:10.1016/S0034-5687(87)80021-X
Schumacher, J. F., Carman, M. L., Estes, T. G., Feinberg, A. W., Wilson, L. H., Callow, M. E., . . . Brennan, A. B. (2007). Engineered antifouling microtopographies – effect of feature size, geometry, and roughness on settlement of zoospores of the green alga Ulva. Biofouling, 23(1), 55-62. doi:10.1080/08927010601136957
Service, U. S. F. a. W. (2019, 5 May 2020). Bald Eagle Fact Sheet. Retrieved from https://www.fws.gov/midwest/eagle/Nhistory/biologue.html
Shafey, T. M. (2002). Effects of Egg Size and Eggshell Conductance on Hatchability Traits of Meat and Layer Breeder Flocks. Asian-Australas J Anim Sci, 15(1), 1-6. doi:10.5713/ajas.2002.1
Smith, A. J. (1974). Changes in the average weight and shell thickness of eggs produced by hens exposed to high environmental temperatures—A review. Tropical Animal Health and Production, 6(4), 237-244. doi:10.1007/BF02383283
Smith, B. (2017, 25 April 2017). How to Calculate a Shock Load. Retrieved from https://sciencing.com/calculate-weight-per-linear-foot-8066865.html
Solomon, S. E. (2010). The eggshell: strength, structure and function. British Poultry Science, 51(sup1), 52-59. doi:10.1080/00071668.2010.497296
Stoddard, M. C., Yong, E. H., Akkaynak, D., Sheard, C., Tobias, J. A., & Mahadevan, L. (2017). Avian egg shape: Form, function, and evolution. Science, 356(6344), 1249-1254. doi:10.1126/science.aaj1945
Taylor, T. G. (1970). How an eggshell is made. Scientific American, 222(3), 88-95. doi:10.1038/scientificamerican0370-88
Tryjanowski, P., Sparks, T. H., Kuczyński, L., & Kuźniak, S. (2004). Should avian egg size increase as a result of global warming? A case study using the red-backed shrike (Lanius collurio). Journal of Ornithology, 145(3), 264-268. doi:10.1007/s10336-004-0035-8
Van Toledo, B., Parsons, A. H., & Combs, G. F. (1982). Role of Ultrastructure in Determining Eggshell Strength. Poultry Science, 61(3), 569-572. doi:10.3382/ps.0610569
Veldsman, L. m., Kylin, H., Bronkhorst, P., Engelbrecht, I., & Bouwman, H. (2020). A method to determine the combined effects of climate change (temperature and humidity) and eggshell thickness on water loss from bird eggs. Environmental Geochemistry and Health, 42(3), 781-793. doi:10.1007/s10653-019-00274-x
Willems, E., Decuypere, E., Buyse, J., & Everaert, N. (2014). Importance of albumen during embryonic development in avian species, with emphasis on domestic chicken. World’s Poultry Science Journal, 70(3), 503-518. doi:10.1017/S0043933914000567
Yavari, A. (2010, 23 June 2010). quasi static loading. Retrieved from https://imechanica.org/node/8437