The Biochemical Features of Tardigrades and Their Roles in Maintaining Cellular Functional Integrity
Luccia Jabbour, Eden Karp-Foster, Jeremy Lachance, Wadi Zahka
Abstract
Extensive studies have been carried out regarding tardigrades’ so-called tun state as a means of survival in the face of all sorts of harsh environmental conditions. While the surviving capabilities of tardigrades in inhospitable habitats are outstanding and worth examining, many of the ways by which they succeed in maintaining their physiological integrity from within, on the biochemical level, are as promising in terms of bioengineering applications, whether it be related to how cryptobiosis is signaled in cells or to how they mitigate adverse biochemical reactions occurring inside of them, such as those involving DNA damage, reactive oxygen species (ROS) and heavy metals. Cryptobiosis is essentially triggered by the presence of ROS and the consequent oxidation of cysteine, as well as by a deficiency in ATP in anhydrobiosis. Bioprotectant molecules and a reduction in mitotic activity are also key factors for the maintenance of cellular integrity in the tun state. As for DNA protection, tardigrades generally rely on both DNA shielding and DNA repair mechanisms, while specialized enzymes protect tardigrades against ROS and heavy metals, which are toxic due to their propensity to cause oxidative stress.
Introduction
Tardigrades, commonly referred to as “water bears” or “moss piglets”, are very small invertebrates (body length spanning from 0.05 mm to 1.2 mm), and close relatives to arthropods, belonging to the phylum Tardigrada. Their initial discovery in 1773 is credited to Johann August Ephraim Goeze, who bestowed upon them the name “water bears” due to their bear-like movements. However, three years later, Lazaro Spallanzani modified their name to “Tardigrada”, which translates to “slow stepper” (Macdonald, 2004).

Figure 1: Scanning electron micrograph of a tardigrade (Britannica, 2023)
Tardigrades are remarkable microscopic organisms characterized by their short and plump barrel-shaped bodies. They exhibit a distinctive appearance with four pairs of limbs, each terminating in four to eight claws or suction disks. The tough cuticle covering their bodies resembles the exoskeleton of insects and other arthropods, and like insects, tardigrades undergo molting to shed their cuticle during growth. The body is divided into two parts: a head region and a body with fused segments. The head is composed of a tubular mouth encircled by stylets used for piercing plant cells, algae, and other organisms for sustenance. A triradiate, which is a muscular pharynx that leads to a short tubular esophagus, is present in the mouth. It continues down to the intestine, occupying most of the organism’s length. The body cavity of the tardigrade is a hemocoel filled with fluid, serving as a medium for oxygen and water transport since tardigrades lack specialized organs for respiration or circulation. Their brains are bilaterally symmetrical, paired with neuron clusters, and are connected to a large ganglion below the esophagus, giving rise to a double ventral cord that extends throughout the body. The ventral cord contains ganglions on each segment of the body where lateral nerves extend towards the limbs. Additionally, many tardigrades exhibit a pair of compound cup-shaped eyes and sensory bristles distributed across the head and the body. Finally, tardigrades exhibit diverse reproductive strategies depending on the species, showcasing both sexual and asexual modes of reproduction. Typically oviparous, these microscopic organisms engage in external fertilization in most species. During this process, the slightly larger female Tardigrade undergoes molting, shedding its cuticle to lay eggs within it. These eggs are then fertilized externally by the male. However, certain species employ internal fertilization, wherein the male deposits sperm inside the female’s cuticle, offering a variation in their reproductive mechanisms (Weronika, 2017).
The tardigrades possess remarkable polyextremophilic traits, thriving across various ecosystems from mountain tops to deep seas, mountain volcanoes, intertidal zones, deserts, urban areas, and even Arctic ice niches (Reyes et al., 2023). However, this species’ preferred habitats are freshwater mosses and lichens. What makes the tardigrades ripe for research is their ability to withstand very harsh and extreme environments, such as challenging terrains, due to their microscopic size, highly fluctuating conditions, rapidly changing humidity, and extreme temperatures.
These organisms are able to survive hostile environmental conditions because of their ability to perform cryptobiosis, a state of lowered metabolic activity and desiccation (Macdonald, 2004). Tardigrades were even experimented on by the European Space Agency, which decided to send two tardigrade species (Richtersius coronifer and Milnesium tardigradum) to space at low Earth orbit during the FOTONM3 mission. The samples were exposed to space vacuum and UV radiation and survived (Jönsson, 2008).
In this study, several biochemical features of tardigrades are highlighted, encompassing the chemical operations underlying their cryptobiotic strategies, their resistance to different mechanisms, such as radiation and toxic substances, and the armor built notably in response to environmental stress, that is antioxidant defenses.
Reactive oxygen species (ROS) is a key concept in these biochemical features of tardigrades. On one hand, exposure of tardigrades to toxic substances leading to ROS production contributes to oxidative stress. On the other hand, this triggers the metabolic shutdown of tardigrades, authorizing them to enter cryptobiosis, a complex but adaptive strategy used by tardigrades. Therefore, ROS, despite being a source of damage, tardigrades nonetheless are able to cope with them thanks to powerful antioxidant enzymes.
Tardigrades are able to maintain the integrity of their genome and preserve their microscopic structure, which would both be severely jeopardized, especially in the face of harsh environmental conditions, if it were not for their latter essential adaptations.
Chemical mechanisms underlying the tardigrade’s cryptobiotic strategies
Tardigrades are well-known for their ability to enter a state of dormancy in response to life-threatening environmental conditions. While in this dormant phase, their metabolism is drastically reduced and they become inactive, enabling their survival during periods of deleterious conditions. Anhydrobiosis is the most commonly studied dormancy strategy, though it is only observed in Eutardigrades (one of two main tardigrade groups). The process entails the expulsion of up to 98% of the body’s water content over a few hours, causing the tardigrade to become completely desiccated and able to maintain a low metabolic rate. Studies in the late twentieth century established that tun formation, wherein the tardigrade contracts its permeable cuticles, is a key component of the success of anhydrobiosis (Crowe, 1972).
Anhydrobiosis is not the only dormancy strategy pursued by tardigrades. In fact, tardigrades are the only family in the animal kingdom observed to undergo both quiescent and diapause forms of latency. Quiescence is exogenous; it is induced and concluded by external environmental factors, including temperature, salinity, and oxygen levels. It can occur at any stage of the life cycle (Bertolani, 2004). On the other hand, diapause is endogenous; it is induced by environmental factors but regulated and concluded by internal physiological mechanisms. It often follows seasonal, cyclical changes and, “in contrast to quiescence, does not automatically arrest with the termination of adverse conditions” (Bertolani, 2004). In tardigrades, quiescence takes the form of cryptobiosis while diapause is comprised of encystment (the formation of a protective cyst around the body), cyclomorphosis (seasonal changes in phenotype), and resting eggs (delayed hatching) (Guidetti and Rebecchi, 2011). Additionally, diapause and quiescence can occur either as individual processes or at the same time, as they are driven by separate mechanisms.
Despite the fact that quiescent strategies are so integral to tardigrade’s survival, relatively little is known about forms of quiescence other than anhydrobiosis. Moreover, it is only recently that the mechanisms behind these strategies have begun to be studied in depth. Mapping the underlying chemical processes facilitating cryptobiosis is critical to understanding not only the survival strategy of the tardigrade as a whole but also nature’s approach to battling difficult environmental challenges.
Forms of cryptobiosis in tardigrades
As mentioned previously, anhydrobiosis is not the only cryptobiotic dormancy strategy in tardigrades, though it is the most heavily researched and the most well-understood. Other forms of cryptobiosis occurring in tardigrades include cryobiosis, anoxybiosis, osmobiosis, and possibly chemobiosis. Each type of cryptobiosis is brought about by different environmental conditions and each presents different advantages.
Firstly, anhydrobiosis is triggered by conditions leading to rapid evaporative water loss and is generally observed in terrestrial tardigrades (Northcote-Smith, 2012). During the anhydrobiotic process, tardigrades “contract their body into a so-called tun, losing most of their free and bound water (>95%)” (Bertolani, 2004). By entering a state of near-total desiccation at a controlled rate, through anhydrobiosis, tardigrades are able to slow metabolic rate and survive such adverse environmental conditions.
Cryobiosis, meanwhile, is induced by extremely low temperatures and is characterized by the ability to survive both freezing and thawing. It is observed not only in polar regions (Somme & Meier, 1995) but also in temperate or tropical alpine areas (Halberg et al. 2009, Hengherr et al. 2009, Rebecchi et al. 2009). Cryobiotic tardigrades have been shown to survive temperatures as low as -196 °C and cooling rates as rapid as 30 °C/min (Ramløv and Westh, 2001). Unlike in anhydrobiosis, tardigrades undergoing cryobiosis do not enter a full tun state, though they may have a partially contracted body (Møjberg and Neves, 2021).
Anoxybiosis is triggered by low oxygen tension in aquatic environments. Anoxybiotic tardigrades are characterized by “a turgid and elongated body” and do not form tuns (Møjberg and Neves, 2021). However, it should be noted that there is some debate regarding the ability of tardigrades to survive anoxic conditions. According to Guidetti et al., “there is little clear experimental evidence to support anoxybiotic ability in tardigrades” (2011). Despite this, Guidetti et al. note that a species of tardigrade was observed to be able to survive up to six months in asphyxial conditions (in rotting barnacles) (Kristensen & Hallas, 1980).
Osmobiosis is induced by high osmotic pressures. Like anhydrobiosis, it leads to the formation of a tun (Møjberg and Neves, 2021). This is speculated to occur to prevent fluid loss due to the osmotic process in high-pressure environments.
Finally, chemobiosis is triggered by high toxin concentrations in the surrounding environment. Preliminary studies suggest that it can lead to tun formation (Smythers et al., 2023). However, very little research exists on chemobiosis in tardigrades as an adaptive form of cryptobiosis.
These dormancy strategies are crucial to ensuring that the tardigrade is able to survive adverse environmental conditions. However, it is impossible to fully grasp these strategies without an understanding of the mechanisms behind them. Research on the chemical processes underlying the various forms of cryptobiosis is still in its infancy; however, several promising advances have been made in recent years, particularly in the consideration of cryptobiotic forms that induce tun formation.
Inducing cryptobiosis
Though there is not a wealth of information surrounding the mechanisms behind many forms of tardigrade cryptobiosis, there is even less surrounding the successful induction of cryptobiosis via environmental triggers. Much of the past literature has focused specifically on anhydrobiosis since this is perhaps the easiest form of cryptobiosis to study. Recent breakthroughs, however, have led to the discovery of a mechanism that is potentially responsible for inducing all forms of cryptobiosis observed in tardigrades.
Reversible cysteine oxidation and ROS
In one of the first empirical studies of chemobiosis in tardigrades, it was demonstrated that tardigrades expel their water content even without low humidities (as in anhydrobiosis) and high osmotic pressures (as in osmobiosis) (Smythers et al., 2023). According to Smythers et al., this suggests that “water expulsion during tun formation is an active process rather than the result of spontaneous flux, and that the mechanism(s) of expulsion could be mediated by oxidation or oxidative signaling” (2023). In fact, it is shown that reversible cysteine oxidation, as mediated by reactive oxygen species (highly reactive chemicals composed of water, dioxygen, and hydrogen peroxide), plays a significant role in triggering cryptobiosis in tardigrades.
Reversible cysteine oxidation is a process that occurs on cysteine – an amino acid that plays a crucial role in metabolism regulation – wherein the thiol group of cysteine undergoes reversible oxidation and loses electrons to ROS. This process has been shown to induce tun formation in tardigrades. For instance, Smythers et al. demonstrated that blocking cysteine oxidation leads to a significant decrease in tun formation, as demonstrated in Figure 2. This was accomplished through the use of iodoacetamide (IAM) and N-ethylmaleimide (NEM), both of which are shown to “irreversibly bind to reduced cysteine thiols and block oxidation” (Smythers et al., 2023). Specifically, cysteine oxidation was shown to play a role in limb contraction during the formation of the tun.

Fig. 2. Graph depicting % tun formation for tardigrades with non-blocked cysteine oxidation, tardigrades with cysteine oxidation blocked through IAM, and tardigrades with cysteine oxidation blocked through NEM. The graph illustrates the significant decrease in tun formation following cysteine oxidation blocking, suggesting that this process is critical to tun formation (Smythers et al., 2023).
However, even in cryptobiotic forms where tun formation does not occur, such as cryobiosis, it is still the case that cysteine oxidation is necessary for tardigrade survival. NEM exposure prior to freezing of tardigrade specimens was shown to result in tardigrades “becoming more linear and distended during freezing, likely contributing to the lack of any survivors following reheating to room temperature” (Smythers et al., 2023). Thus, reversible cysteine oxidation is critical not only to quiescent strategies involving tun formation but also to non-tun-forming varieties of cryptobiosis. These findings indicate that reversible cysteine oxidation plays a role in triggering not just tun formation, but cryptobiosis itself.
Further experiments showed that “environmental ROS or the intracellular production of ROS in the presence of external stressors triggers tardigrades to enter cryptobiosis” (Smythers et al., 2023). Essentially, it is ROS accumulation in intracellular regions that triggers reversible cysteine oxidation and, in turn, cryptobiosis, as summarized in Figure 3. ROS itself is regulated by ion influxes, particularly the influx of calcium through the voltage-gated anion channel 2 (VDAC2). This implies that ROS levels are modulated by the flow of calcium through VDAC2, and that “VDAC2 closes to decrease flux in response to external stressors” (Smythers et al., 2023).
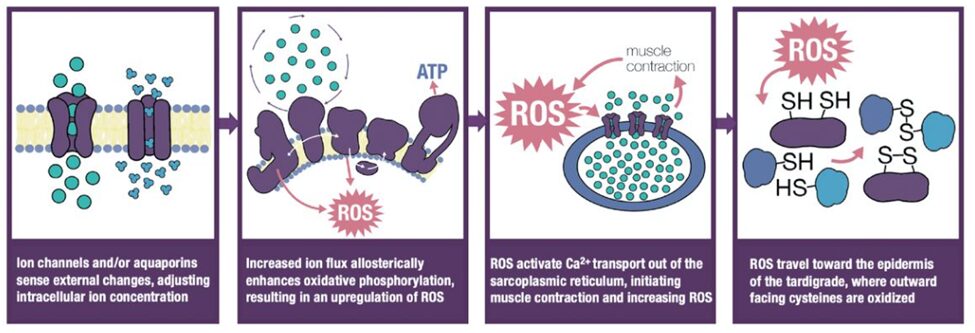
Fig. 3. Graphical depiction of ROS mediation of cysteine oxidation within the cell (Smythers et al., 2023).
In summary, reversible cysteine oxidation is a central factor in inducing cryptobiosis in both tun and non-tun forming types. It is regulated by intracellular ROS levels, which itself is modulated by the flux of calcium through the channel VDAC2.
APMK and protein phosphatase activity in anhydrobiosis
In anhydrobiosis specifically, where there is a far greater wealth of research, the enzyme AMP-activated protein kinase (AMPK) has been demonstrated to play a critical role in regulating cellular growth and adaptively reprogramming metabolism through transcriptional changes (Mihaylova and Shaw, 2011). AMPK is activated when intracellular ATP levels are lowered, meaning it can be activated in response to resource scarcity.
In order to test the hypothesis that AMPK activity is required for inducing anhydrobiosis in tardigrades, Kondo et al. developed an experiment, summarized in Figure 4, in which an H. exemplaris tardigrade specimen was pre-treated with AMPK inhibitor solution (2019). The study found that AMPK inhibition “significantly impaired anhydrobiotic survival,” as shown in Figure 4.
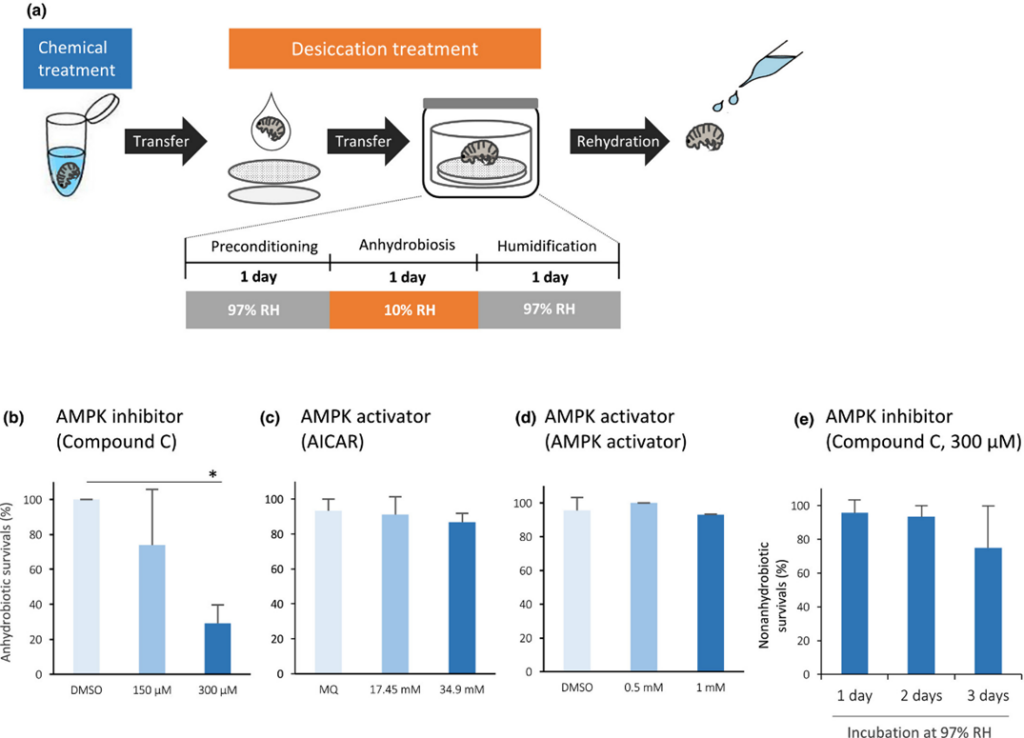
Figure 4 (a): Graphical depiction of the experiment performed by Kondo et al., in which tardigrades were treated with an AMPK inhibitor solution before anhydrobiosis (2019).
Figure 4 (b) – (e): Charts depicting a decrease in anhydrobiotic survival following AMPK inhibition (b), little to no change in anhydrobiotic survival following AMPK activation (c) (d), and little change in non-anhydrobiotic survival following AMPK inhibition. These results suggest that AMPK activity occurs during anhydrobiosis, since additional activation has no effect (c) (d), and is critical to anhydrobiotic survival (b), but is not necessary when anhydrobiosis does not take place (e) (Kondo et al., 2019).
It was also shown that the enzyme protein phosphatase 2A (PP2A) plays an important role in regulating AMPK activity. PP2A is shown to increase dephosphorylation levels of AMPK during periods of mild desiccation stress (Kondo et al., 2019). In fact, both PP1A and PP2A, as well as de novo gene expression, are required for anhydrobiosis to be carried out successfully (Kondo et al., 2015).
Protective mechanisms of cryptobiosis
Inducing quiescence (and often tun formation) is not the only factor that must be considered in the pursuit of dormancy strategies. Existing in this state has many advantages for the tardigrade, but it is also incredibly taxing on the body and has the potential to cause considerable damage even to the DNA itself, requiring mitigation through supplemental evolutionary solutions.
Desiccation bioprotectants and cellular remodeling: an overview
A wide range of bioprotectant molecules, depicted in Figure 5, are involved in increasing the tardigrade’s desiccation resistance, improving its ability to avoid desiccation-induced damage to cell membranes, proteins, and even DNA. Several such bioprotectants have been discussed in a previous essay; however, they still bear repeating.
Firstly, Late Embryogenesis Abundant (LEA) proteins and Heat-shock proteins (Hsps) are proposed to play a role in preventing the aggregation of proteins during desiccation and hence act as “molecular shields and chaperones” (Møbjerg and Neves, 2021). In particular, Hsps are observed to bind to proteins denatured by water loss, thereby preventing them from aggregating within the cell (Wang et al., 2014). During rehydration meanwhile, up-regulated Hsps are found to “reinitiate productive protein folding pathways and re-establish membrane fluidity” (Wang et al., 2014). This is particularly relevant considering that rapid rehydration following cryptobiotic reversal has the potential to cause cellular damage, as discussed further in section 2 (Rinehart and Denlinger, 2004).
Tardigrade-specific proteins (TDPs) are proposed to play a role in stabilizing the cell and its elements both by replacing water during desiccation but also by forming a glass-like matrix within cells, “physically preventing protein denaturation, protein aggregation, and membrane fusion” (Boothby et al., 2017). Heat-soluble tardigrade-specific intrinsically disordered proteins, including cytoplasmic, mitochondrial, and secreted abundant heat-soluble proteins (CAHS, MAHS, and SAHS), are specifically associated with desiccation tolerance during the process of anhydrobiosis.

Fig. 5. Graphical summary of cellular bioprotectants involved in cryptobiosis and rehydration. Note that Dsup is discussed in Section 3 (Møjberg and Neves, 2021).
Beyond these bioprotectants, it is also found that the cytoskeleton of the cell will undergo changes in its structural makeup in order to withstand the pressures caused by volume changes during desiccation and rehydration. In particular, it is found that cytoskeleton components such as beta-actin and beta-tubulin are downregulated, while cofilin is upregulated (Wang et al., 2014). Cofilin is involved in binding and disassembling actin filaments. These changes enable the cytoskeleton to weather the deformative forces created during desiccation and rehydration, presumably by making the cytoskeleton less rigid and more flexible.
Reduction in mitotic activity
In response to the induction of cryptobiosis, it is also observed that the tardigrade reduces its rate of cellular reproduction through the suppression of elements involved in mitotic activity. It is possible that this enables the tardigrade to reserve energy for essential functions during dormancy. In particular, it is observed that cryptobiosis induces downregulation of tardigrade-specific elongation factors, which facilitate translation elongation, i.e., the process of adding additional amino acids to a polypeptide chain during protein synthesis (Wang et al., 2014). Additionally, two types of histone-encoding transcripts, namely H3.3 and H4, are found to be repressed in the cryptobiotic state. In fact, histones are “the main protein component of the chromatin and important for DNA packing and gene expression regulation” (Wang et al., 2014). The fact that elongation factors and histone production are suppressed suggests that there exists a specific mechanism to reduce mitotic activity in tardigrades following the induction of cryptobiosis.
The tardigrade’s maintenance of its genomic integrity
Most tardigrades’ extreme and more common environments pose a risk to their corporal integrity. Although it is well documented that tardigrades can withstand very harsh conditions while in their so-called tun state, this latter mechanism brings itself a natural tendency to cellular denaturation, down to the level of genes (Neumann et al., 2009). In fact, Neumann’s study states that the severity of the damages to DNA observed after recovery from the tun state are proportional to the time spent in such state by a tardigrade, Milnesium tardigradum, in this case; in fact, (2.09 ± 1.98)% of the DNA were damaged after two days in the tun state, whereas (23.66±7.56)% of it were damaged after ten months in the tun state (Figure 6).

Figure 6: Percentage of damaged DNA with respect to the time spent in the tun state. %DNA “in tail” refers to the technique used by Neumann et al. to visualize damaged DNA, that is single gel electrophoresis (comet assay). Fragments being of smaller size, they are expected to end up in the “tail” of the gel, meaning its end, after an electric field is applied [Adapted from Neumann et al., 2009].
The more significant damages after a longer time are perhaps more visible when observed qualitatively on a gel used in electrophoresis (Figure 7).

Fig. 7. Milnesium tardigradum‘s DNA after different times in the tun state. A: Negative control (no time in the tun state). B: Two days in the tun state. C: Six weeks in the tun state. D: Ten months in the tun state (Neumann et al., 2009).
Among the possible explanations for DNA denaturation in the tun state, it is hypothesized that an accumulation of reactive oxygen species (ROS), which would cause the oxidation of the DNA, is more difficult to eliminate over time in the absence of metabolism and energy-requiring antioxidative defenses, despite tardigrades’ high ability to mitigate oxidative stress.
Moreover, extreme temperatures, especially hot temperatures, are known to be detrimental to tardigrades’ DNA stability, as they lead to the formation of more hydroxyl groups, which are in fact ROS (Neves et al., 2022). Though not all tardigrades inhabit extreme environments that are characterized by particularly hot temperatures or other conditions that necessitate extensive times in the tun state, other environments in which tardigrades live – bryophytes, for instance – are subject to great variations in temperature, humidity, and ultraviolet exposure, as only a thin layer of protection contributes to shelter them (Miller, 2011).
The extent of tardigrades’ radiotolerance
Exposure to radiation has emerged to be, in the majority of studies, the most convenient means to assess tardigrades’ resistance to accumulating DNA damage. While this resistance itself can be considered as an adaptation relative to environmental challenges and to the constraints posed by the tun state, radiotolerance can hardly be taken as an adaptation to any habitat, since no ecological niche on Earth appears to be exposed to radiation of the same intensity as those that tardigrades can withstand as a viable limit (Beltrán-Pardo et al., 2013). Nonetheless, it is certain that tardigrades’ habitats’ high variability represents a conundrum to be addressed in that it may lead to higher ultraviolet exposure, as mentioned. As a matter of fact, studies have established that all tardigrade species confounded exhibit a significant resistance to UV radiation and show an average LD50[1] after exposure during one to two days equal to 4-6 kGy with X-ray, gamma (γ), and alpha (α) radiation, which entails that tardigrades execute both highly efficient DNA protection and DNA repair mechanisms. For comparison, doses higher than 4 Gy lead to death in half of humans, which is more than a thousand times less (Hashimoto & Kunieda, 2017). Both categories of adaptations will be tackled.
Ionizing radiation can be distinguished into two different types in biological irradiation contexts; it can either be low linear energy transfer (low-LET) irradiation or high linear energy transfer (high-LET) irradiation (Jönsson, 2019). While low-LET irradiation is represented by X-rays and γ-rays and results in the creation of excessive ROS that damage DNA and proteins, high-LET irradiation is notably represented by protons and helium ions (α particles) and results in direct damages induced by the deposition of energy in cells. DNA lesions are notably characterized by double-strand breaks (Hashimoto & Kunieda, 2017) and thymine dimers (Altiero et al., 2011). Further statistical data reveal that most tardigrades remarkably cope with both types of radiation, with average LD50swidely comparable to the one given by Beltrán-Pardo et al. for low-LET irradiation. Some species of tardigrades have average LD50s even more outstanding for high-LET irradiation; for instance, Ramazzotius coronifer’s LD50 for high-LET irradiation has been estimated to 10 kGy, suggesting that it is even more efficient in coping with direct than with indirect DNA and biomolecular damages. As shown in Figure 8 and Figure 9, hydrated tardigrades also tend to exhibit higher survival rates than anhydrobiotic ones in irradiation conditions. As for tardigrades’ resistance to ultraviolet radiation, the inverse tendency has been observed in most studies (Altiero et al., 2011; Horikawa et al., 2013; Jönsson, 2019), but only under higher temperatures (Jönsson, 2019). Considering that tardigrades rely on both DNA repair mechanisms and the avoidance of DNA damage and that the anhydrobiotic state is marked by an interruption of the metabolism, it is possible to hypothesize that hydrated tardigrades possess the advantage of being able to count on such repair mechanisms as part of their metabolic activity over desiccated tardigrades in addition to avoiding DNA damage.
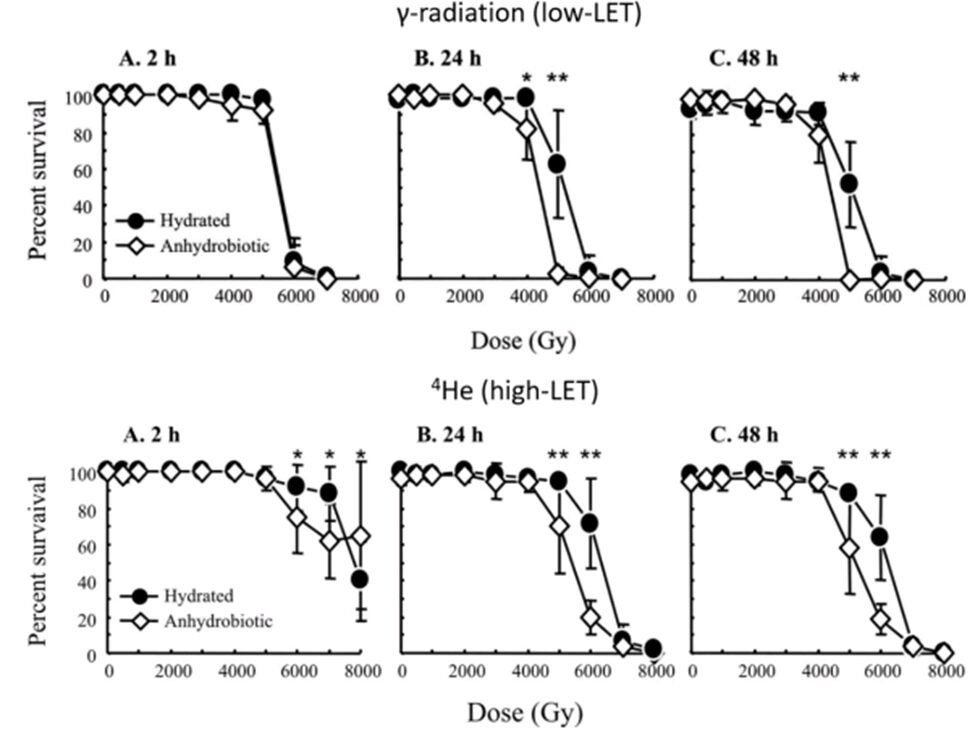
Figure 8: Survival percentages of Milnesium tardigradum to both low-LET and high-LET irradiation for different durations and doses. As depicted by the six graphs, M. tardigradum in its hydrated state generally tends to be better able to cope with both types of irradiations than it does in its anhydrobiotic state (Jönsson, 2019).

Figure 9: Tolerance to ionizing radiation in different species of tardigrades. As in Figure 8, it is shown that hydrated tardigrades generally cope better with irradiation than anhydrobiotic ones. N.B: Hypsibius dujardini is nowadays called Hypsibius exemplaris (Hashimoto & Kunieda, 2017).
Difference in impact of radiation on early developing tardigrades
Over their early development, tardigrades are subject to a high mitotic activity, and therefore to a high frequency of DNA replication, and are devoid of cellular protection mechanisms until more advanced stages of growth (Jönsson, 2019). Thus, as it is reasonably expected, tardigrade embryos are much more sensitive to radiation in a way inversely proportional to their development advancement (see Figure 10), as it is provided for by the Law of Bergonié and Tribondeau in biology across many organisms’ embryos, and by the fact that irradiation before the mid-blastula stage does not trigger the checkpoint cell cycle responses, which lead to unrepaired and later amplified genetic damage (Beltrán-Pardo et al., 2013). On the other hand, adult tardigrades are less vulnerable to radiation, especially since they grow not by mitosis but by enlargement of their already existing somatic cells (Ingemar Jönsson et al., 2005). Hydrated embryos have also been found to be less likely to hatch when exposed to radiation compared to anhydrobiotic embryos; for instance, LD50s related to high-LET radiation have been established respectively to 509 Gy and 1690 Gy (Jönsson, 2019). It is noteworthy that eggs exposed to more elevated doses of radiation also require more time to hatch, which indicates that some mechanism is at work during the supplementary time for mitigating the lesions caused by irradiation (Beltrán-Pardo et al., 2013). However, no change has been noticed regarding differently irradiated tardigrades’ behavior after hatching, which points to the effectiveness of the latter mechanism that is nonetheless acquired only later during their development.

Figure 10: Proportion of hatched eggs of Milnesium tardigradum exposed to different doses of gamma radiation during different developmental stages. Earlier developmental stages are more sensitive to radiation (Beltrán-Pardo et al., 2013).
Avoiding DNA damage as one strategy
The most known mechanism ensuring the prevention of DNA damage involves the tardigrade damage suppressor protein (Dsup), a protein unique to the tardigrade (Hashimoto & Kunieda, 2017). In Hashimoto’s study, the expression of Dsup in a human-cultured engineered cell line contributed to significantly minimizing DNA fragmentation and conserving cellular morphology and proliferative ability upon and after exposure to X-rays and hydrogen peroxide, a ROS; in fact, such engineered cells were found to have undergone much less DNA breaks, as they expressed an unexpectedly low quantity of γ-H2AX, a marker associated with DNA breaks.
Dsup binds non-specifically (regardless of any specific DNA sequence) to nucleosomes (DNA-histone complexes), which constitute chromatin, the natural packaged structure of the genetic material inside the nucleus, by its C-terminal region (see Figure 11), which is strikingly similar in sequence to the nucleosome-binding domain of high mobility group N (HMGN) proteins found in vertebrates, as shown in Figure 12 (Chavez et al., 2019). Although the association of proteins with DNA normally leads to heterochromatinization and interferences in transcription and replication, its N-terminal region along with its α-helical region allow for the mitigation of those detrimental effects (Hashimoto & Kunieda, 2017). It is noteworthy that more than one molecule of Dsup can bind to one nucleosome for enhanced protection and that Dsup can also simultaneously bind with histone H1, a major nucleosome-binding protein in all animals that is generally bound to every nucleosome (Chavez et al., 2019). Dsup was originally found in Ramazzotius varieornatus and it is known that its structure differs across tardigrade species; the Dsup tackled herein also refers to that of R. varieornatus. However, it was found, for instance, that Hypsibius exemplaris[2] possesses an ortholog protein, meaning that both share a very similar structure and the same function after evolution from a common ancestor.

Figure 11: Theoretical structure of the Dsup protein (Hashimoto & Kunieda, 2017).
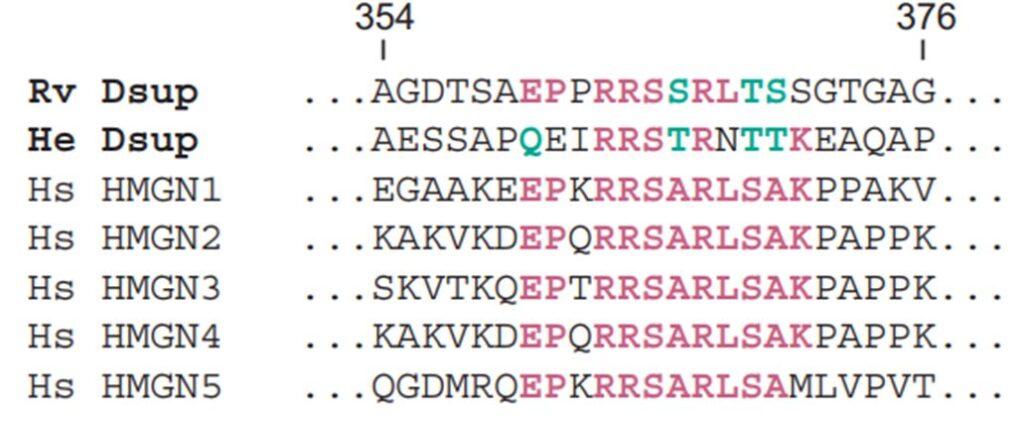
Figure 12: Similarities in sequence of Dsup proteins and the five human HMGN proteins. Rv stands for Ramazzotius varieornatus and He stands for Hypsibius exemplaris (adapted from Chavez et al., 2019).
For what regards the mechanism of action through which Dsup binds to nucleosomes, it was found above all that there exists a significant electrostatic complementarity between them and that, being an intrinsically disordered protein (IDP), Dsup flexibly adapts its structure to DNA when they interact by notably fitting the bent structure adopted by the DNA around histones in the chromatin (Mínguez-Toral et al., 2020). The electrostatic complementarity arises from the fact that Dsup features clusters of positive charges due to lysine and arginine residues that interact strongly with the negative DNA surface. Indeed, the electric field generated by the corresponding Poisson-Boltzmann molecular electrostatic potential (EP) falls into the range of about 0.08 to 0.15 V/Å[3], which is on par with what is observed in photosynthetic reactions and at active enzyme sites; thus, the electric field generated thereby is significantly strong. Figure 13 and Figure 14 represent Dsup relatively to this.
Moreover, Dsup exhibits a decreasing radius of gyration upon interacting with DNA, indicating that it becomes more compact and tighter in the presence of nucleic acids and that it thus abandons its intrinsically disordered nature.
By doing so, Dsup acts as a physical shield onto DNA against the direct effects of radiation, primarily those of high-LET irradiation, by superior absorption of energy, and against the damage induced by ROS, primarily because of low-LET irradiation, as already explained (Jönsson, 2019; Mínguez-Toral et al., 2020).

Figure 13: Three-dimensional representation of Dsup and Poisson-Boltzmann electrostatic potentials of Dsup-DNA complexes (Mínguez-Toral et al., 2020). On the right side of each image, a top-view is provided. (a) and (b): Dsup is shown as green and blue ribbons, DNA is shown as ball-and-stick structures with P atoms in orange, O atoms in red, N atoms in blue, and C atoms in cyan. (c) and (d): Same complexes shown with their Poisson-Boltzmann electrostatic potentials according to the legend. (e) and (f): Dsup and DNA considered with their global Poisson-Boltzmann electrostatic potentials.
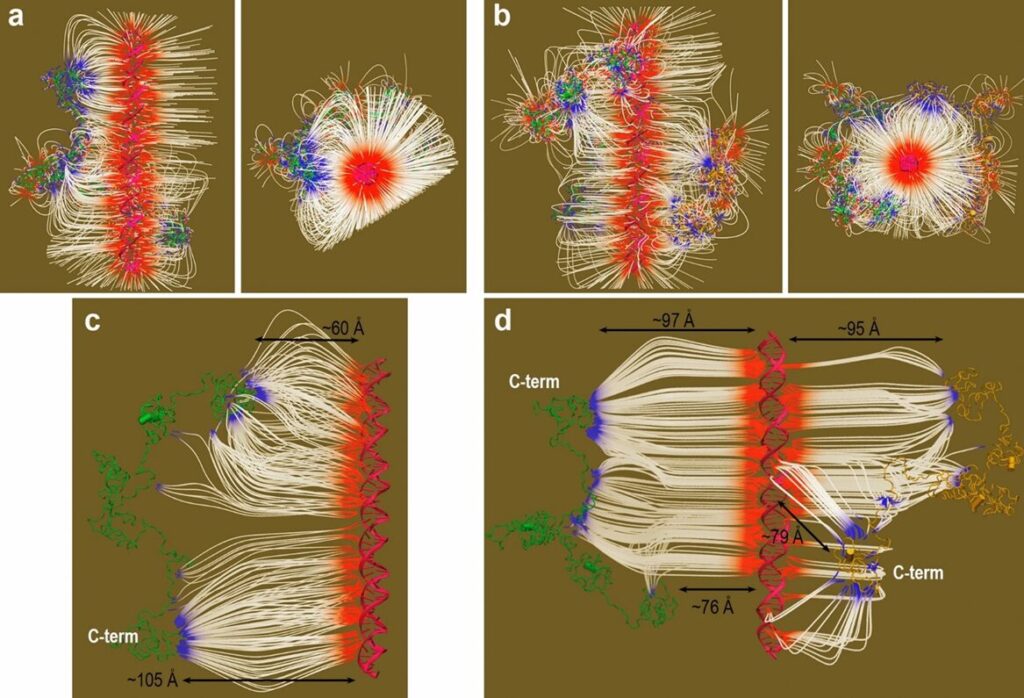
Figure 14: Electric field E existing in Dsup-DNA complexes (Mínguez-Toral et al., 2020). (a) and (b): E= 6 kT, according to the proximity of the Dsup proteins relative to DNA. (c) and (d): E= 3kT (Dsup proteins farther apart from the DNA). On the left, are complexes with one Dsup molecule. On the right, are complexes with two Dsup molecules.
Investigation of DNA repair mechanisms
Omics studies outline the upregulation of different DNA repair pathways upon radiation-induced damage (that is upon DNA damage), among which are the upregulation of the Rad51 protein in the hydrated M. tardigradum, Mre11, DNA repair endonuclease XPF, ubiquitin, DnaJ family, Rhp57, proteasome maturation factor, mutS and POLE (Förster et al., 2012; Jönsson, 2019). DNA and RNA helicase are also found in the transcriptome analysis of tardigrade species (Förster et al., 2012). Thus, this suggests that tardigrades possess all if not most DNA repair pathways that exist in biology and that entail non-homologous end joining, homologous recombination, mismatch repair, nucleotide excision repair, and base excision repair. As an exception, Echisnicoides sigismundi shows a relatively much weaker ability to withstand DNA damage (see Figure 9) since it does not possess mechanisms related to non-homologous end joining for repairing DNA double-strand breaks, and it is also less tolerant to long-term anhydrobiosis (Jönsson, 2019). As a matter of fact, this species lives in marine ecosystems and since it is reasonably exposed to less environmental fluctuations, including exposure to UV radiation, this adaptation may be of no use for it. What is more, not all species of tardigrades are equally equipped with such mechanisms. For instance, M. tardigradum disposes of a more extended set of pathways for these purposes than Hypsibius exemplaris, and its evolution has also been more directed towards developing repair strategies complementarily to direct protective strategies. Again, this is very likely due to the fact that one inhabits a more fluctuating environment than the other and that one is thus more subject to DNA lesions (Förster et al., 2012).
In summary, although resistance to the effects of radiation does not pose a major conundrum for tardigrades’ survival, notwithstanding that many limnoterrestrial species of tardigrades face high variations in ultraviolet exposures in their habitats where thin layers of water can rapidly evaporate, the main interest in assessing their radiotolerance lies in the fact that it is a simple way to assess their resistance to DNA damages, whether it be related to their ability to repair their genetic material when impaired or to their ability to avoid such damages. Such DNA damage can notably occur during the tun state or in the face of harsh environmental conditions. Most tardigrades’ radiotolerance capacities are outstanding and point towards the existence of mechanisms associated with both of these abilities as solutions adopted by the tardigrades throughout their evolution. Dsup displays a strong electrostatic affinity with DNA and also acts as a shield against agents likely to compromise tardigrades’ genomic integrity, while a wide range of biochemical pathways can be put into action in tardigrades in order to repair any damage inflicted on the DNA. Dsup could be of interest in oncology and in the context of spaceflights, which entail high levels of exposure to radiation, knowing that human embryonic kidney cells (HEK293) exhibited enhanced radiotolerance when transfected with the Dsup gene. On the other hand, tardigrades’ repair mechanisms, such as Rhp57, are also found in humans and are therefore being targeted to improve DNA damage tolerance in man, which is an important objective for cancer prevention as well.
Role of antioxidants in the survival of tardigrades
The effects of global climate change are a contributing factor to the decline or even extinction of numerous wild species. In such challenging circumstances, the ability to withstand desiccation became a crucial mechanism for these species to escape the adverse consequences of increasing environmental dryness. Certain animals have developed an impressive adaptive mechanism known as anhydrobiosis, one of the more researched types of cryptobiosis. Anhydrobiosis is the reversible capacity of an organism to endure a significant loss of its bodily water due to evaporation, which occurs as its surrounding habitat progressively dries out (Rebecchi, 2013). However, abiotic factors like high temperatures, high oxygen partial pressure, high relative humidity, and the time spent in a desiccated state can cause cellular damage, like protein and nucleic acid denaturation, as covered in the previous section, membrane destabilization, and oxidative stress with the eventual death of organisms (França, 2007). It is then crucial to understand how tardigrades manage to survive after long periods of desiccation.
Oxidative stress
Oxidative stress refers to the phenomenon caused by an imbalance between the increased production of reactive oxygen species and the limited action of antioxidant defenses and is one of the most harmful consequences of desiccation (Pizzino, 2017). ROS are not always harmful to the organism: they are generated in most cell compartments, serve as messengers, and regulate some physiological phenomena (Weidinger, 2015). However, when water is depleted from anhydrobiotic organisms, such as tardigrades, there is a change in the concentration of ions in the cells. This change in ionic concentration can lead to the formation of ROS, which include both free radicals such as superoxide anion radical, hydroxyl radical, and perhydroxyl radical, and non-radical forms like hydrogen peroxide (França, 2007). Therefore, the increase in the production of ROS and the limited action of antioxidants (because of a lack of an ongoing metabolism) leads to oxidative stress.
Production of reactive oxygen species (ROS) during anhydrobiosis
As previously stated, desiccation has been shown to lead to the increased production of ROS; this was speculated to apply to tardigrades. In order to verify the link between water loss and the production of ROS in these microscopic animals, the production of ROS was studied in the coelomocytes (circular mobile cells found in the body cavity of tardigrades that serve as storage units and carriers for lipids and glycogen). To measure the ROS quantity, a fluorescent probe called 2,7-dichlorodihydrofluorescein diacetate (DCFH2-DA) was used. This probe becomes fluorescent when it reacts with ROS. The storage cells were exposed to desiccation by keeping them in a dried-out state for different durations, ranging from 1 day to 20 days. After rehydrating the storage cells, the intensity of the fluorescent signal emitted by these cells was measured, which provided information about the level of ROS produced in response to desiccation and rehydration (see Figure 15).

Figure 15: Signal emitted by the coelomocytes of the tardigrade Paramacrobiotus spatialis, using the fluorescent probe 2,7-dichlorodihydrofluorescein diacetate (DCFH2-DA). (a) represents hydrated animals (control), (b) represents the desiccated tardigrades for 1 day (3 h after rehydration process), (c) represents the desiccated tardigrades for 1 day (12 h after rehydration process), (d) represents the desiccated tardigrades for 20 days (3 h after rehydration process), (e) represents the desiccated tardigrades for 20 days (12 h after rehydration process) (Giovannini, 2022).
The intensity of the fluorescence signal emitted by the storage cells of desiccated animals is significantly higher than the fluorescence intensity detected in the cells of control animals. Moreover, the intensity of the fluorescence signal was at its highest level in the coelomocytes of animals kept desiccated for 20 days. This proves that the amount of ROS increases with desiccation in tardigrades, as seen in Figure 15. (Giovannini, 2022) This leads to oxidative stress which is very damaging and dangerous for the tardigrade’s survival in their desiccated state.
Use of RNA interference (RNAi) to understand the role of antioxidants
The tardigrade’s ability to tolerate desiccation with the increase of ROS is ripe for scientific exploration. Tardigrades possess protective substances that serve to safeguard the organism’s cellular components during the desiccation process. There exist different types of protective substances: aquaporin proteins (aquaporins 3 and 10), sugar trehalose (trehalose-6-phosphate synthase), and antioxidants (catalase, superoxide dismutase, glutathione peroxidase, glutathione transferase, and glutathione reductase), the latter of which will be explored in depth. To learn about the roles of each protectant, RNA interference (RNAi) was used. RNAi is a method allowing scientists to disrupt the functioning of specific genes within the organism. After targeting each protectant, they were studied to see if these animals could successfully recover after desiccation to determine if the targeted gene for each protectant has an important role in the tardigrade’s ability to survive anhydrobiosis (Giovannini, 2022).
Results of the RNAi
To determine the impact of RNAi on these genes, researchers monitored the motility of P. spatialis specimens that had undergone the RNAi treatment. Three groups were considered for comparison: uninjected control animals (control 1), animals injected with RNase-free water (control 2), and animals injected with double-stranded RNA (dsRNA) targeting the specific gene of interest (experimental group) (Tenlen, 2013). The motility was evaluated at various time points after rehydration following a period of anhydrobiosis (desiccation tolerance): immediately after rehydration (t0), 1 hour (t1), 24 hours (t24), and 48 hours (t48) later. The motility assessment of uninjected and water-injected control animals consistently resulted in 100% motility, demonstrating that the desiccation protocol and the injection process alone did not affect the survival of the tardigrades during anhydrobiosis. However, significant differences were observed in the motility percentages of experimental groups. Specifically, animals injected with dsRNA targeting the antioxidant enzyme genes (gpx, gr, gst, cat, and sod), encoding for the enzymes glutathione peroxidase, glutathione reductase, glutathione transferase, catalase, and superoxide dismutase respectively, exhibited significantly lower motility at various time points after rehydration. (Giovannini, 2022)
Solutions to counter the consequences of anhydrobiosis
As previously shown, ROS production continues even when tardigrades are in a completely dried-out state. This is unusual because many organisms, when desiccated, reduce their metabolic activities to a minimum or halt them entirely. However, tardigrades seem to keep producing ROS during this state. This has consequences on the tardigrade’s body; oxidative stress leads to accumulative molecular damage, but also to a longer time to recover to their normal active state after desiccation because their metabolism is stopped during desiccation, and because their repair systems are not functioning. However, research on tardigrade species revealed that these species have specialized antioxidant strategies to cope with the challenges posed by anhydrobiosis in their unique environments (Rebecchi, 2009). In fact, tardigrades increase the efficiency of antioxidant defenses, including enzymes and antioxidants, which increases the tardigrades’ survival by avoiding the production of ROS (França, 2007). For example, the antioxidant enzyme system becomes more active in desiccated P. richtersi specimens (Rizzo, 2010). The difference in strategies might be due to genomic variations, different habitats and geographical regions, dietary preferences, etc. (Rebecchi, 2009)
In conclusion, the process of anhydrobiosis is crucial for the survival of many species, including tardigrades. Nonetheless, anhydrobiosis is very complex and takes a heavy toll on an organism. As a result, natural solutions have been created by tardigrades to survive during the process of anhydrobiosis and return to their normal behavior after desiccation. The most important solution protecting tardigrades from harmful consequences after anhydrobiosis is the availability of antioxidants that play a massive role in protecting tardigrades from an excess of ROS.
Resistance to toxic substances
At the intersection of biology and chemistry, little is currently known about tardigrades’ tolerance towards environmental toxicants, and a lot of mechanisms are still to be studied (Hygum et al., 2017). In fact, water bears have various mechanisms of resistance, going from DNA repair mechanisms to antioxidants.
As a result of anthropogenic disturbances related to the disruption of the ecosystem due to human-induced activities, there is a huge discharge of contaminants like pesticides, detergents, and heavy metals into their environments (Hygum et al., 2017). Heavy metals are non-biodegradable elements, making their toxicity highly persistent. Cadmium and copper, respectively Cd with atomic number 48 and Cu with atomic number 29 on the periodic table, are two of the more commonly used heavy metals. It is important to effectively monitor and track down the presence of heavy metals, especially in aquatic ecosystems because they can lead to ecological imbalances even in trace concentrations. This could also be a threat and pose risks to human health: “High concentrations of metals such as cadmium, lead, and mercury present a threat to human health through drinking water supplies. Also, consuming seafood contaminated with metals can cause these toxic metals to accumulate in human tissue” (Jaishankar et al., 2014). The World Health Organization has set a maximum limit amount allowable for Cd and Cu in freshwater: 3.0 ppb and 2.0 ppm respectively (Opeyemi Oluwadamilola Ojekunle, 2022). Heavy metals are toxic substances for most organisms; however, tardigrades have demonstrated a limited but remarkable way of coping with these metals using their defense mechanisms; antioxidants. Studies mostly focus on copper and cadmium, which are potent toxicants found in aquatic environments. Nonetheless, it is important to monitor and be aware of the production of ROS to understand tardigrades’ stress responses and resilience. When the amount of ROS produced exceeds the body’s ability to eliminate them, cell damage induced by oxidative stress is possible (Olatoregun, 2021). In the study, the produced reactive oxygen species were confirmed with fluorescence microscopy (Opeyemi Oluwadamilola Ojekunle, 2022).
In this study, it was hypothesized that Hypsibius exemplaris (H. exemplaris) has a low tolerance to a high concentration of toxicants (Opeyemi Oluwadamilola Ojekunle, 2022). Copper and cadmium tolerance have been linked to sodium turnover rates, which in turn are strongly related to body size (Hygum et al., 2017). The morphology changes of the tardigrades are examined as they are being exposed to different concentrations of these metals and at different exposure times.
In the assays, only highly active tardigrades were used, chosen based on whether they exhibited clear movement or responded to tactile stimuli. On the other hand, if they did not respond to any of these signs, then they were considered to have entered cryptobiosis, characterized by a metabolic activity shutdown (Hygum et al., 2017). Lastly, the average size of the species used in this research was about 528 µm = 0.528 mm.
In the latter study, two solutions were prepared, one with copper and another with cadmium in which several dilutions were held, aiming to create various concentrations for keeping track of how tardigrades respond to the different concentrations of Cd and Cu. In the experiment, tardigrades were first collected from the stock solution, which is a solution diluted from a known concentration. Once collected, they were then subdivided into small groups of 50 and exposed to a solution of Cd and Cu at various concentrations; ranging between 0.025 ppb to 500 ppb for cadmium and between 0.05 ppm to 2 ppm for copper. Exposure times were 2, 4, 6, and 24 hours (Opeyemi Oluwadamilola Ojekunle, 2022).

Figure 16: Fluorescence at 0.4 ppm of Cu after 6 hours (left) and after 24 hours (right). For tardigrades exposed to copper concentrations, the images were produced using a microscope with a 4x objective lens and DS-Qi1Mc camera). The orange arrow indicates the length of the tardigrades at various exposure concentrations while the blue one shows the presence of water bears. Here, the metabolic activity of tardigrades when exposed to concentrations of 0.4 and 0.6 ppm at different exposure times is investigated. It is clearly depicted that the size of the tardigrades kept on decreasing when time and concentration increased. Fluorescence increased and started appearing after 6 hours (Figure 16) and was at its highest point after 24 hours when the metabolic activity was down to about 20%. Using a concentration of 0.4 ppm, the size decreased from 350 µm to 220 µm. Using a concentration of 0.6 ppm, the size decreased to 332 µm. At this time, the metabolic activity was down to 20% (Opeyemi Oluwadamilola Ojekunle, 2022).

Figure 17a: Fluorescence at 0.5 ppb of Cd after 6 hours (left) after 24 hours (right).
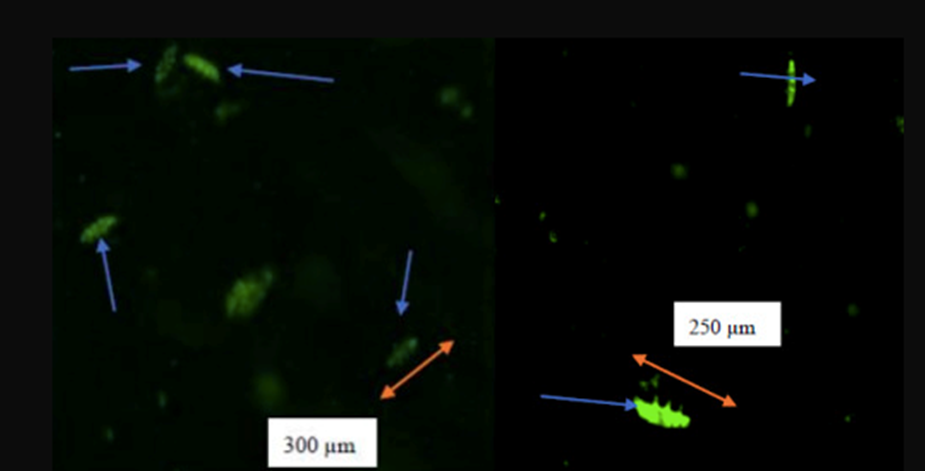
Figure 17b: Fluorescence at 1.0 ppb of Cd after 6 hours (left) and after 24 hours (right).
Figure 17: Fluorescence at different concentrations of cadmium at different times. As mentioned in Figure 16, the size of tardigrades kept on decreasing with the increasing exposure time and concentration of cadmium. Fluorescence was observed after 6 hours of exposure to 0.5 ppb and the highest intensity was at 1.0 ppb as shown in Figure 17b. For both concentrations, the size of the tardigrade decreased; that is from 328 to 245 μm for a concentration of 0.5 ppb of Cd and from 300 to 250 μm for a concentration of 1.0 ppb. This clearly shows that Cd exerts an influence on this species (Opeyemi Oluwadamilola Ojekunle, 2022).
Based on this experiment, the appearance of fluorescence at 6 hours proves the start of emission of the toxic effects exerted by the metals, which leads to choosing the 6 hours exposure time as the sufficient length of time to allow harmful and toxic effects of Cu and Cd to occur (Opeyemi Oluwadamilola Ojekunle, 2022). The intensity of the fluorescence appears to increase with increasing time exposure and concentrations as shown in Figures 16 and 17, proving the significant production of ROS.
Interestingly, the size of H. exemplaris was reduced. This is clearly depicted in the fluorescent imaging, where the dimensions of the tardigrade kept on reducing, indicated by the orange-colored arrows in the images above, and reducing their metabolic activity with the increase of period exposure to cadmium and copper. The metabolic activity of water bears is clearly impacted by their exposure to concentrations of the two heavy metals; copper and cadmium. It decreases as the concentration of heavy metals increases.
As was observed, cadmium and copper solutions increased the tardigrade’s sensitivity to oxidative stress, which is the main toxicity mechanism of heavy metals. To cure the potentially hazardous and detrimental effects of heavy metals, tardigrades have the ability to produce antioxidants which play a role in cell protection, as mentioned in the previous section (Olatoregun, 2021). They are involved in the prevention of cellular damage by removing oxidative stress and are crucial for reducing the damage caused by ROS to the cell. To heal the exposed tardigrade from induced ROS, an antioxidant, such as selenium, could be used to abate the effects of toxicity and avoid the alternation of enzymatic pathways (Opeyemi Oluwadamilola Ojekunle, 2022).
Thus, findings suggest that exposure of tardigrades to cadmium and copper concentrations results in the release of antioxidative enzymes. The defense system of antioxidants helps neutralize ROS and gives tardigrades the resilience of withstanding the effect of toxicity given rise to by heavy metals. However, this resilience is limited: as tardigrades are continuously exposed to higher concentrations of heavy metals and at a higher rate, the production of antioxidative enzymes is reduced, leading to harmful consequences (Olatoregun, 2021). Antioxidants are just a piece of the puzzle in tardigrades’ strategy for coping with these substances. Many other ones are yet to be studied.
Conclusion
The tardigrade has evolved remarkable chemical adaptations to be able to survive environmental conditions that are highly non-conducive to life, as summarized in Figure 18. They are able to survive low humidity levels, high osmotic pressures, low temperatures, low oxygen levels, high toxin concentrations, and more. In particular, it is the evolution of cryptobiosis, as triggered by ROS (and in turn leading to reversible cysteine oxidation), that enables their survival during long periods of deleterious conditions by enabling a reduction of the metabolism. Essentially, fundamental functions do not need to operate properly if they are not needed to the same extent. Moreover, it is the production of chemical bioprotectants (such as TDPs, Hsps, and Dsup) that allow the tardigrade to withstand the immense damage on the body caused by tun formation, often occurring in conjunction with cryptobiosis. Finally, additional protective mechanisms, such as those protecting the body from heavy metals and high ROS levels, are also critical to enabling the tardigrade to navigate their extreme environments with a higher rate of survival.
In fact, these design solutions can all be classified as “protective mechanisms” that have evolved in response to the incredibly adverse conditions tardigrades are subjected to in their environments. Further research into such mechanisms could reveal pertinent applications in a wide range of fields, particularly in medicine. For instance, it is believed that, with the ability to induce cryptobiosis in human cells and drastically reduce their metabolism, progressive diseases could be halted in their tracks and cellular death could be prevented (Westover et al., 2022). However, it is clear that there is a large informational gap surrounding the biochemical processes underlying these protective functions that needs to be bridged before anything close to these types of engineering innovations can be achieved.
Ultimately, nature has had many millennia to develop the design solutions we see around us today. Despite the exponential advancement of human technology, it is likely that we will need a lot more time before we are able to come close to replicating the complexity and ingenuity of the design solutions harbored in nature.

Fig. 18.. Diagram representing the conundrums (left) faced by the tardigrade and the solutions (right) that have been evolved to mitigate them.
References
Altiero, T., Guidetti, R., Caselli, V., Cesari, M., & Rebecchi, L. (2011). Ultraviolet radiation tolerance in hydrated and desiccated eutardigrades. Journal of Zoological Systematics and Evolutionary Research, 49, 104-110. https://doi.org/https://doi.org/10.1111/j.1439-0469.2010.00607.x
Beltrán-Pardo, E., Jönsson, K. I., Wojcik, A., Haghdoost, S., Harms-Ringdahl, M., Bermúdez-Cruz, R. M., & Bernal Villegas, J. E. (2013). Effects of ionizing radiation on embryos of the tardigrade Milnesium cf. tardigradum at different stages of development. Plos one, 8(9), e72098.
Bertolani R., Guidetti R., Jönsson K.I., Altiero T., Boschini D., Rebecchi L. (2004). Experience with dormancy in tardigrades. J. Limnol. 63. 16-25. https://doi.org/10.4081/jlimnol.2004.s1.16.
Boothby T.C., Tapia H., Brozena A.H., Piszkiewicz S., Smith A.E., Giovannini I., Rebecchi L., Pielak G.J., Koshland D., Goldstein B. Tardigrades Use Intrinsically Disordered Proteins to Survive Desiccation. Mol Cell. 2017 Mar 16;65(6):975-984.e5. https://doi.org/10.1016/j.molcel.2017.02.018. PMID: 28306513; PMCID: PMC5987194.
Britannica, T. Editors of Encyclopaedia (2023, October 17). tardigrade. Encyclopedia Britannica. https://www.britannica.com/animal/tardigrade
Chavez, C., Cruz-Becerra, G., Fei, J., Kassavetis, G. A., & Kadonaga, J. T. (2019). The tardigrade damage suppressor protein binds to nucleosomes and protects DNA from hydroxyl radicals. eLife, 8, e47682.
Crowe, J. H. (1972). Evaporative Water Loss by Tardigrades under Controlled Relative Humidities. Biological Bulletin, 142(3), 407–416. https://doi.org/10.2307/1540318
Förster, F., Beisser, D., Grohme, M. A., Liang, C., Mali, B., Matthias Siegl, A., Engelmann, J. C., Shkumatov, A. V., Schokraie, E., & Müller, T. (2012). Transcriptome analysis in tardigrade species reveals specific molecular pathways for stress adaptations. Bioinformatics and biology insights, 6, BBI. S9150.
França, M. B., Panek, A. D., & Eleutherio, E. C. (2007). Oxidative stress and its effects during dehydration. Comparative biochemistry and physiology. Part A, Molecular & integrative physiology, 146(4), 621–631. https://doi.org/10.1016/j.cbpa.2006.02.030
Giovannini, I., Corsetto, P. A., Altiero, T., Montorfano, G., Guidetti, R., Rizzo, A. M., & Rebecchi, L. (2022). Antioxidant Response during the Kinetics of Anhydrobiosis in Two Eutardigrade Species. Life (Basel, Switzerland), 12(6), 817. https://doi.org/10.3390/life12060817
Guidetti R., Altiero T., Rebecchi L. On dormancy strategies in tardigrades. J Insect Physiol. 2011 May;57(5):567-76. https://doi.org/10.1016/j.jinsphys.2011.03.003. Epub 2011 Mar 21. PMID: 21402076.
Hashimoto, T., & Kunieda, T. (2017). DNA protection protein, a novel mechanism of radiation tolerance: lessons from tardigrades. Life, 7(2), 26. https://doi.org/https://doi.org/10.3390/life7020026
Hengherr S., Worland M.R., Reuner A., Brümmer F., Schill R.O.. High-temperature tolerance in anhydrobiotic tardigrades is limited by glass transition. Physiol Biochem Zool. 2009 Nov-Dec;82(6):749-55. https://doi.org/10.1086/605954. PMID: 19732016.
Horikawa, D. D., Cumbers, J., Sakakibara, I., Rogoff, D., Leuko, S., Harnoto, R., Arakawa, K., Katayama, T., Kunieda, T., & Toyoda, A. (2013). Analysis of DNA repair and protection in the Tardigrade Ramazzottius varieornatus and Hypsibius dujardini after exposure to UVC radiation. Plos one, 8(6), e64793.
Hygum, T. L., Fobian, D., Kamilari, M., Jørgensen, A., Schiøtt, M., Grosell, M., & Møbjerg, N. (2017). Comparative Investigation of Copper Tolerance and Identification of Putative Tolerance Related Genes in Tardigrades [Original Research]. Frontiers in Physiology, 8. https://doi.org/10.3389/fphys.2017.00095
Ingemar Jönsson, K., Harms-Ringdahl, M., & Torudd, J. (2005). Radiation tolerance in the eutardigrade Richtersius coronifer. International journal of radiation biology, 81(9), 649-656.
Jaishankar, M., Tseten, T., Anbalagan, N., Mathew, B. B., & Beeregowda, K. N. (2014). Toxicity, mechanism and health effects of some heavy metals. Interdiscip Toxicol, 7(2), 60-72. https://doi.org/10.2478/intox-2014-0009
Jönsson, K. Ingemar, et al. Tardigrades Survive Exposure to Space in Low Earth Orbit, current biology, 9 Sept. 2008, doi.org/10.1016/j.cub.2008.06.048.
Jönsson, K. (2019). Radiation Tolerance in Tardigrades: Current Knowledge and Potential Applications in Medicine. Cancers, 11 (9), 1333. In.
Kinchin, I. M. (1994). The Biology of Tardigrades [Review of The Biology of Tardigrades]. Portland Press, London.
Kondo K., Kubo T, Kunieda T. Suggested Involvement of PP1/PP2A Activity and De Novo Gene Expression in Anhydrobiotic Survival in a Tardigrade, Hypsibius dujardini, by Chemical Genetic Approach. PLoS One. 2015 Dec 21;10(12):e0144803. https://doi.org/10.1371/journal.pone.0144803. PMID: 26690982; PMCID: PMC4686906.
Kondo, K., Mori, M., Tomita, M., Arakawa, K. AMPK activity is required for the induction of anhydrobiosis in a tardigrade Hypsibius exemplaris, and its potential up-regulator is PP2A. Genes Cells. 2019; 24: 768–780. https://doi.org/10.1111/gtc.12726
Kristensen, R.M. and Hallas, T.E. (1980), The Tidal Genus Echiniscoides and Its Variability, with Erection of Echiniscoididae fam.n. (Tardigrada)1. Zoologica Scripta, 9: 113-127. https://doi.org/10.1111/j.1463-6409.1980.tb00657.x
Macdonald, H. (2004) Geologic Puzzles: Morrison Formation, Starting Point. Retrieved Sept 9, 2004, from http://serc.carleton.edu/introgeo/interactive/examples/morrisonpuzzle.html
Mihaylova M.M. and Shaw R.J. The AMPK signalling pathway coordinates cell growth, autophagy and metabolism. Nat Cell Biol. 2011 Sep 2;13(9):1016-23. https://doi.org/10.1038/ncb2329. PMID: 21892142; PMCID: PMC3249400.
Miller, W. R. R. (2011). Tardigrades: These ambling, eight-legged microscopic” bears of the moss” are cute, ubiquitous, all but indestructible and a model organism for education. American Scientist, 99(5), 384-391.
Mínguez-Toral, M., Cuevas-Zuviría, B., Garrido-Arandia, M., & Pacios, L. F. (2020). A computational structural study on the DNA-protecting role of the tardigrade-unique Dsup protein. Scientific Reports, 10(1), 13424.
Møbjerg N., Neves R.C. New insights into survival strategies of tardigrades. Comp Biochem Physiol A Mol Integr Physiol. 2021 Apr;254:110890. https://doi.org/10.1016/j.cbpa.2020.110890. Epub 2020 Dec 26. PMID: 33373690.
Neumann, S., Reuner, A., Brümmer, F., & Schill, R. O. (2009). DNA damage in storage cells of anhydrobiotic tardigrades. Comparative Biochemistry and Physiology Part A: Molecular & Integrative Physiology, 153(4), 425-429.
Neves, R. C., Møbjerg, A., Kodama, M., Ramos-Madrigal, J., Gilbert, M. T. P., & Møbjerg, N. (2022). Differential expression profiling of heat stressed tardigrades reveals major shift in the transcriptome. Comparative Biochemistry and Physiology Part A: Molecular & Integrative Physiology, 267, 111169.
Northcote-Smith, E. (2012). “The ecology of tardigrades,” The Plymouth Student Scientist, 5(2), p. 569-580. http://hdl.handle.net/10026.1/14001
Olatoregun, A. (2021). Investigating the Detoxification of Cadmium by the Tardigrade Hypsibius Exemplaris. [Dissertation]. https://digitalscholarship.tsu.edu/dissertations/34/?utm_source=digitalscholarship.tsu.edu%2Fdissertations%2F34&utm_medium=PDF&utm_campaign=PDFCoverPages
Opeyemi Oluwadamilola Ojekunle, D. O., Sonya Good, Ayodotun Sodipe. (2022). Toxicological Impact of Cadmium and Copper Exposure in H. Exemplaris.11, Article 2. https://doi.org/https://www.macrothink.org/journal/index.php/emsd/article/view/19434
Pizzino, G., Irrera, N., Cucinotta, M., Pallio, G., Mannino, F., Arcoraci, V., Squadrito, F., Altavilla, D., & Bitto, A. (2017). Oxidative Stress: Harms and Benefits for Human Health. Oxidative medicine and cellular longevity, 2017, 8416763. https://doi.org/10.1155/2017/8416763
Ramløv, H., Westh, P. (2001). Cryptobiosis in the Eutardigrade Adorybiotus (Richtersius) coronifer: Tolerance to Alcohols, Temperature and de novo Protein Synthesis. Zoologischer Anzeiger. 240. 517-523. 10.1078/0044-5231-00062.
Rebecchi, L., Cesari, M., Altiero, T., Frigieri, A., & Guidetti, R. (2009). Survival and DNA degradation in anhydrobiotic tardigrades. The Journal of experimental biology, 212(Pt 24), 4033–4039. https://doi.org/10.1242/jeb
Rizzo, A. M., Negroni, M., Altiero, T., Montorfano, G., Corsetto, P., Berselli, P., Berra, B., Guidetti, R., & Rebecchi, L. (2010). Antioxidant defences in hydrated and desiccated states of the tardigrade Paramacrobiotus richtersi. Comparative biochemistry and physiology. Part B, Biochemistry & molecular biology, 156(2), 115–121. https://doi.org/10.1016/j.cbpb.2010.02.009
Smythers, A. L., Joseph, K. M., O’Dell, H. M., Clark, T. A., Crislip, J. R., Flinn, B. B., Daughtridge, M. H., Stair, E. R., Mubarek, S. N., Lewis, H. C., Kolling, D. R. J., & Hicks, L. M. (2023). Chemobiosis reveals tardigrade tun formation is dependent on reversible cysteine oxidation. bioRxiv, 2023.2005.2015.540801. https://doi.org/10.1101/2023.05.15.540801
Sømme, L., Meier, T. Cold tolerance in Tardigrada from Dronning Maud Land, Antarctica. Polar Biol 15, 221–224 (1995). https://doi.org/10.1007/BF00239062
Tenlen, J. R., McCaskill, S., & Goldstein, B. (2013). RNA interference can be used to disrupt gene function in tardigrades. Development genes and evolution, 223(3), 171–181. https://doi.org/10.1007/s00427-012-0432-6
Wang, C., Grohme M., Mali, B., Schill, R., Frohme, M. “Towards decrypting cryptobiosis—analyzing anhydrobiosis in the Tardigrade Milnesium tardigradum using transcriptome sequencing.” PLoS ONE, vol. 9, no. 3, 2014, https://doi.org/10.1371/journal.pone.0092663.
Weidinger, A., & Kozlov, A. V. (2015). Biological Activities of Reactive Oxygen and Nitrogen Species: Oxidative Stress versus Signal Transduction. Biomolecules, 5(2), 472–484. https://doi.org/10.3390/biom5020472
Weronika, E., Łukasz, K. Tardigrades in Space Research – Past and Future. Orig Life Evol Biosph 47, 545–553 (2017). https://doi.org/10.1007/s11084-016-9522-1
C. Westover, D. Najjar, C. Meydan, K. Grigorev, M.T. Veling, R.L. Chang, C. Chin, D. Butler, E.E. Afshin, P.A. Silver, C.E. Mason, Multi-omics analysis of Dsup expressing human cells reveals open chromatin architectural dynamics underyling radioprotection, BioRxiv. (2020) 2020.11.10.373571. https://doi.org/10.1101/2020.11.10.373571.
[1] LD50 is a measure of the median lethal dose. LD50 = 4 – 6 kGy means that 50% of analyzed tardigrades die upon exposure to 4 – 6 kGy.
[2] Updated name for Hypsibius dujardini.
[3] About 8 × 108 V/m to 1.5 × 109 V/m.