Master of Camouflage: Chemical Deception in Insect and Plant Species
Christopher Coluni, John Eric Hamilton, Olivia Lopardo, Olivier Morin
Abstract
When the topic of camouflage and mimicry is discussed, it is often associated with the idea of colors and complex patterning. The visual aspect of camouflage is something that many are familiar with. Plenty of organisms express an array of vibrant colours and deceiving visuals for both protection and methods of attraction. This is all common knowledge when discussing camouflage but one area that is overlooked is the role of chemistry. For this research essay, the main objective is to introduce a variety of camouflaging examples with an acute focus on the chemical mechanisms found within them. To accomplish this, attention was directed towards the lower trophic levels of producers and primary consumers as they possess some of the most elaborate biochemical mechanisms in biology. Visual camouflage is often an effective countermeasure to predators who take advantage of sight in order to hunt prey. However, a different type of camouflage also coexists and relates to the field of chemical ecology. Chemical mimicry bases itself on the concept of chemical signals and the physiological responses they create when interpreted by a receiving organism. This is a method used by both primary consumers and producers as they tend to communicate through chemically-mediated interactions. While this will be discussed in detail with the help of both pollination and host mimicry examples, the paper will also highlight some biochemical mechanisms found in insect bioluminescence and simple plant mimicry.
Introduction
Living organisms perform mimicry by taking advantage of chemical signals and information from their surrounding environment. It occurs in order to take advantage of certain resources, like in the case of pollination mimicry where plants take advantage of surrounding insects to reproduce. Another example is host mimicry where insects use the nests of other organisms to take care of their offspring. However, they can also resort to mimicry in order to protect or defend themselves from their surrounding environment. In the case of protective mimicry, a plant can use physical cues and turn them into electrical signals to mimic the nature of a deceased plant. Finally, Batesian mimicry is a defense mechanism where a harmless organism mimics the signature of a poisonous one to repel predators. In all these cases, the organisms take advantage of certain chemical mechanisms that their species have developed over many years of evolution in order to dupe the beings that are in its ecosystem in order for them to survive.
Pollination Mimicry
Flowers are only able to reproduce if there is an intermediary which brings pollen from another flower and deposits it into its stigma. From there, the pollen will make its way down the style to fertilize an ovule (Pereira & Coimbra, 2019). These intermediaries can be the wind, herbivorous animals, but most commonly this work is done by insects. However, these insects require an incentive to complete their natural tasks. Many species of flowers developed mechanisms to help them survive with the help of insects by providing them with some sort of motivation.
As such, the flowers and intermediary insects coexist by the “trading of floral resources and pollination services” (Lunau & Wester, 2017). This means that the flowers get fertilized, and the insects receive some reward like nectar. In some cases, flowers have evolved to take advantage of the insects that help them, and this is what is called deceptive mimicry. This is where the flower has nothing to offer but it makes the insect think it does. It uses different tricks to achieve this, one of them being sexual deception.
Sexual deception is a subclass of pollination mimicry. The flowers make use of this mimicry, by imitating a member of a certain species, usually the female, with the goal of attracting the male so that it comes and fertilizes it (Schiestl et al., 1999).
A good example of this kind of mimicry are the flowers of the genus Ophrys with the solitary bees, Andrena nigroaenea (Figure 1). First, the orchids resemble the shape and colour of the female bees (Schiestl et al., 1999) which grabs the attention of the bees. There are also some chemicals that are present in the surroundings of the flower that mimic the female bees sex pheromones (Schiestl et al., 1999). Pheromones are used by living beings to “identify mating partners of the opposite gender” (Gomez-Diaz & Benton, 2013). Usually, once the female is ready to reproduce, it emits the pheromones in the air to gain the attention of the males in the surrounding areas. The orchid flowers can mimic this signal type to dupe the male individuals into thinking they are also female bees. According to studies conducted by Schiestl et al. (1999), the hydrocarbon molecules contained in the plant's surface wax have another function other than water loss control. It acts as a mimic of the sex pheromone of the female bees. There was not a specific hydrocarbon that was found to exactly mimic the pheromone but rather a mixture of different saturated and unsaturated alkenes (Mant et al., 2005). Once they receive the signals from the plants, the male individuals become attracted to it as they are attracted to females. They attempt to reproduce with the so-called female but then realize that it isn't really a female bee but only a flower. After they have tried to reproduce with the flower that they landed on, this flower receives the pollen from another flower of the species that the bee had been carrying (Monteiro et al., 2012) and attaches some pollen from itself to be able to pollinate other flowers.
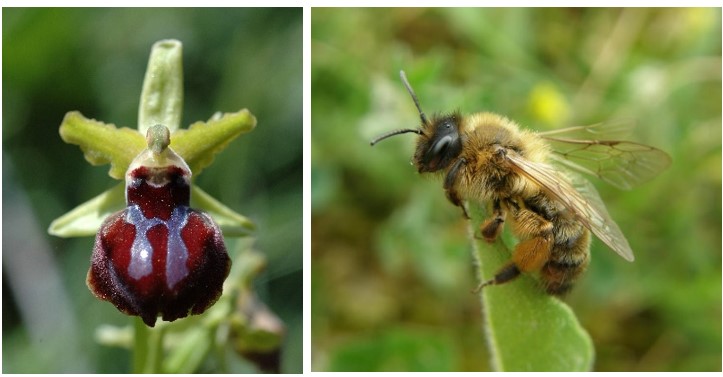
In some other studies, it was found that there is more than one type of chemical that attracts them. There is the previously talked about one and another one called linalool that acts more as a longer-range attractant (Mant et al., 2005) (Figure 2). However, the latter is only used to attract whereas the hydrocarbon chain pheromones are used to “induce copulatory behaviour” (Mant et al., 2005). In other words, the linalool is used to enhance the effects of the mimic pheromones.
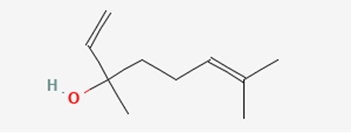
The production of these hydrocarbon chains is said to be related to a protein called stearoyl-acyl carrier protein desaturase or SAD2 (Monteiro & Sebastiana, 2012). This protein is present in the mechanism of synthesis of alkenes only and its role is the desaturation of the alkane chain (Schlüter et al., 2011). The protein is also responsible for the placement of the double bond on the hydrocarbon chain (Figure 3). It was observed in Ophrys sphegodes, a type of orchid, that SAD2 was expressed at a higher rate, and it resulted in greater amounts of 9 and 12 alkenes hydrocarbon chains being produced compared to other species (Monteiro et al., 2012). This ratio of compounds was shown to attract specifically the Andrena nigroaenea bee species. This leads to the belief that the genes that are responsible for coding for SAD2 can be classified as a “barrier gene” (Schlüter et al., 2011) and separate different orchid species' pollinators and therefore the pollen that is pollinating them, which decreases the possibility of cross-species reproduction. In other words, each orchid species has its preferred pollinator species, and it can attract it by creating a mixture of hydrocarbon chains that it knows mimics well the sex pheromone of that species.
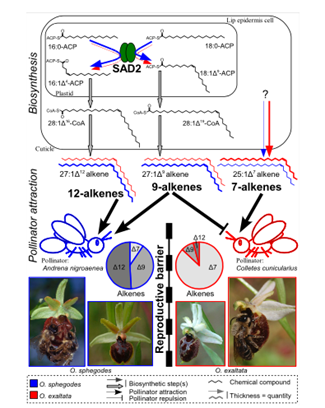
Some bees can learn from their mistakes after being tricked by these sexually deceptive plants. They are said to “become aversive to the location of the deception” and “rarely visit multiple neighbouring orchids” (Gaskett, 2011). This means that these pollinators do have some memory retention and are able to avoid the orchids in the surrounding areas of where they were deceived.
Another way that orchids use chemicals to their advantage and manipulate the bees is by using “farnesyl hexanoate, an antiaphrodisiac compound” (Mant et al., 2005). Usually, this substance is used by female bees to let the surrounding males know that she has already found a mate (Mant et al., 2005). The orchid flowers use this but again to their advantage. They produce this substance but use it so that the male bees do not come and try to copulate with them. This corresponds to the opposite effect of their usual behaviour but they have a purpose behind it. They try to direct the bees to help other orchid flowers that have not been “mated with” yet so that more of the individuals in the species can also perform well (Mant et al., 2005).
Orchids have become intelligent in the way they perform this sexual deception because they are able to time their flowering cycle at a precise time. This time coincides with the time the male pollinating bees start to look for mates. Also, during this time, the female bees have not yet started looking (Lunau & Wester, 2017). This allows them to have the upper hand when deceiving the male bees because there is no competition.
To summarize, pollination mimicry is an evolutionary skill that orchids have developed to mimic the sex pheromone of solitary bees, and this allows them to get pollinated without needing to reward the bees with anything in return.
Host Mimicry
Much like terrestrial and aquatic species, insects must also face the reality of predation pressure. Visual camouflage is one form of defense allowing for the survival of certain species but not all organisms locate prey through optical mechanisms (Akino, 2005). At the lower trophic levels, organisms communicate through chemically-mediated interactions and require different defensive mechanisms to survive (d'Ettorre, 2016). Cryptic colouring and dynamic skin texturing fail at bypassing non-visual predators and this is where the concept of chemical deception comes into play (Akino, 2005). An important display of this form of chemical mimicry arises in the relationship between inquiline insects and ants (Dettner & Liepert, 1994). As a result of their size, many underestimate the predatory nature of the ant species, who are notoriously aggressive towards non-nestmates (Bos & d'Ettorre, 2012). The following pages will first discuss the mechanisms behind insect recognition, followed by a discussion of the biochemical mechanisms allowing for camouflage in insects.
Cuticular Hydrocarbons in Chemical Signaling and Recognition
Ants are often referred to as social insects, as their highly advanced societies are based on the act of communication (d'Ettorre, 2016). Being primarily odor guided, ants recognise other organisms based on their cuticular hydrocarbon label (Bos & d'Ettorre, 2012). Hydrocarbon recognition is paramount within ant colonies as it provides them with a method of eliminating potentially harmful non-nestmates (Bos & d'Ettorre, 2012). When an intruder is intercepted, ant species will compare the foreign hydrocarbon label to their own respective template. If the oncoming chemical signal falls short of the template-label acceptance range, the organism will simply be rejected (Bos & d'Ettorre, 2012). Therefore, in order for an organism to be accepted by the ant colony, they would have to chemically camouflage themselves by mimicking a familiar signal. These signals are referred to as “colony odors” and if an intruder can recreate this familiar scent, they can avoid attacks (d'Ettorre, 2016).
As the name suggests, cuticular hydrocarbons are composed of carbon and hydrogen atoms and reside on the outer surfaces of insects (Ginzel & Blomquist, 2016). Initially designed to prevent water loss, these hydrocarbons, composed mainly of straight chain, unsaturated and methyl branches, have become a key component in chemically-mediated interactions (Figure 4) (Ginzel & Blomquist, 2016). Hundreds of these hydrocarbons exist, varying not only from the number of carbons but from the position and geometry of bonds and functional groups (Millar, 2010). Furthermore, methyl-branched chains contain stereoisomeric potential which is yet another distinguishing factor in cuticular hydrocarbons. For example, 3-methylheptacosane can exist as two stereoisomers whereas 9,13,17-trimethylheptacosane can form 8 molecular geometries (Figure 4) (Millar, 2010). In short, all of these structural variations within the molecules allow for a very diverse array of chemical signals and physiological responses (Ginzel & Blomquist, 2016).
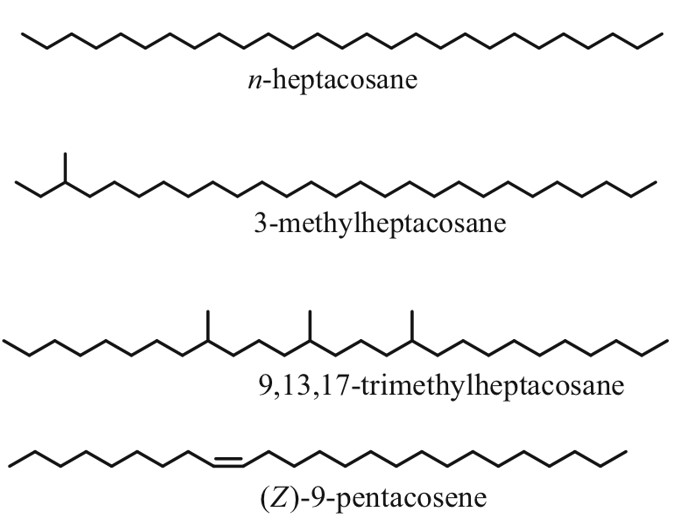
Insect Recognition in Ant species: The Olfactory System
Although much is left to be discovered on how ants process these chemical signals within their brain, their olfactory system is well understood (d'Ettore, 2016). Chemical signals emitted from ants and intruders are intercepted by the ants' antennas (Bos & d'Ettorre, 2012). Olfactory receptors, which are sophisticated sensory organs, detect the hydrocarbon pattern first and relay a message to the main antennal lobe (Bagnères, 2020). This input is then transferred to more complex regions of the nervous system (i.e., mushroom bodies) for further analysis (Figure 5) (d'Ettore, 2016). Impressively, the sensory organs on ant antennas are so powerful that they are capable of distinguishing between enantiomers of certain molecules and between relative concentrations of the same hydrocarbon (Bagnères, 2020; d'Ettore, 2016). The latter implies that even though a mimic is capable of recreating the same hydrocarbon signal, if it is not found in similar quantities, the ant can uncover the true identity of the intruder and attack. In brief, this trajectory map of the ant's olfactory system (shown in Figure 5 & 6) is complex, but not without reason. Not only do these organisms need to detect and recognize hundreds of unique chemical structures, they also need a means of remembering them (Bos & d'Ettorre, 2012).
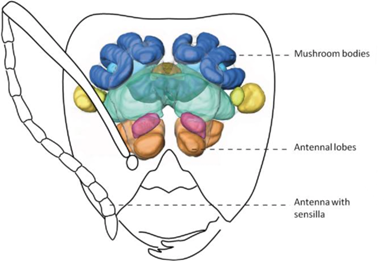
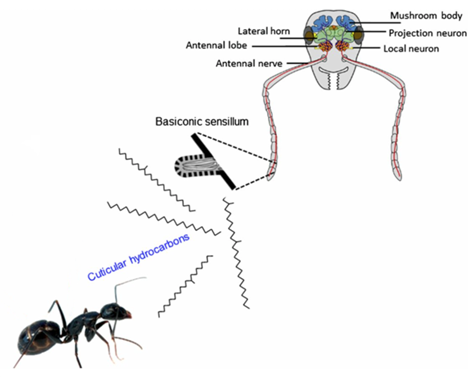
Biochemical mechanisms in Inquiline Chemical Mimicry
Branching away from the concepts of hydrocarbon signaling and insect recognition, it is now time to introduce the impersonators. As a result of there being many examples of mimics within the insect world, the following discussion will focus on inquiline insects and how they take advantage of ant colonies (Dettner & Liepert, 1994). The term inquiline is given to organisms who exploit the living space of others. This may sound familiar to parasitism, but these insects are not causing any harm.
Inquilines infiltrate the ant colonies by mimicking the chemical label of young ant pupae. A wonderful example of this is displayed within the Syrphid fly, a member of the genus Microdon (Dettner & Liepert, 1994). After mating, adult flies lay their eggs inside of the ant colony. Instead of being attacked, these fly larvae are accepted by the ant host and treated as their own offspring (Figure 7) (Dettner & Liepert, 1994). Interestingly enough, these young fly larvaes are actually primary predators towards the ant species taking care of them and as a result of similar hydrocarbon labels, the host species is incapable of distinguishing a difference.
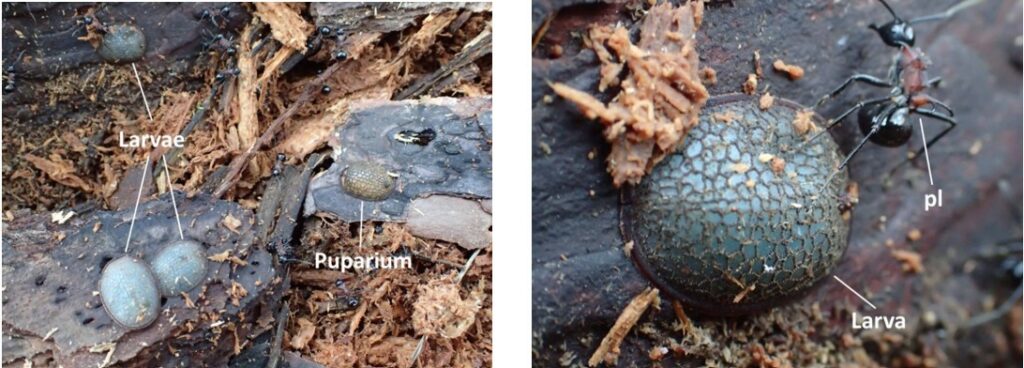
These flies mimic the ant's hydrocarbon signals through biosynthesis. This biochemical mechanism takes place internally in regions called oenocytes. Once the carbon hydrogen chains are formed, they are transported in full length towards the cuticle where they can be interpreted by the host (Howard et al., 1990).
Biosynthesis in insects
Biosynthesis is essentially the process where simple substrates are converted into large macromolecules. In insects, this is the process of creating large, branched molecules containing upwards of 40+ carbons (Ginzel & Blomquist, 2016). The formation of these chains is a result of a multi-step chemical reaction requiring energy, catalytic components and intermediates. An example of a biosynthetic reaction, taken directly from the book Extracellular Composite Matrices in Arthropods, has been greatly simplified in order to provide a general sense of the reactions occurring within the oenocytes (Ginzel & Blomquist, 2016).
Step 1: Formation of the precursor; a carbon chain containing methyl groups and fatty-acids

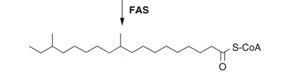
Step 2: Fatty acid elongation
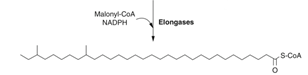
Step 3: Fatty acid to aldehyde conversion
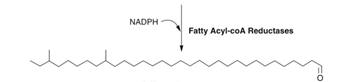
Step 4: Decarbonylation of aldehyde functional groups
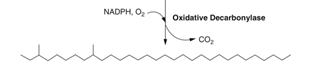
After this set of chemical pathways, the result is a saturated carbon chain containing two methyl groups, situated on the Carbons 3 and 9. The function of this chain in particular is unknown, but field research concludes that some contact pheromones have been observed to have similar structures to the final product in Step 4 (Millar, 2010).
To summarize, host mimicry in insect species is a multifaceted mechanism. For it to function correctly, three elements are required: A chemical signal composed of hydrocarbons, a receiving organism (the host organism) and a mimic capable of biosynthesis. Although one example was presented, further readings can be done on beetles, spiders and bees who employ similar chemical deception techniques to take advantage of their hosts (Dettner & Liepert, 1994).
Protective Mimicry
The Mimosa pudica plant has developed a unique type of protection mimicry which tricks preying organism that physically stimulates it to believe that it is dead. Through a series of electrical and biochemical signals, the plant's motor organ, known as pulvinus, will rapidly close its leaves onto the stem and will lower its branches to deter the predator (Figure 8) (Volkov et al., 2010). There are three major steps required in the process of nastic movements: the recognition of a mechanical stimulus, the propagation of the stimulus-induced signal, and the chemical exchanges which alter the pulvini shape (Hagihara & Toyota, 2020). The chemical properties and reactions present in the last two steps of the process will be emphasized throughout the analytical breakdown of the M. pudica mimicry technique.
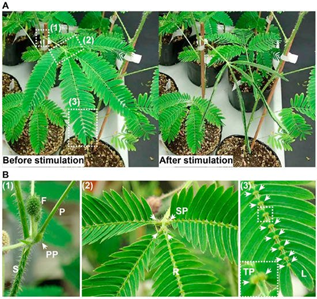
Propagation of the mechanically-induced stimulus:
The process of M. pudica sensing an initial movement and converting the mechanical sensation into an electrical or chemical signal is still being explored, yet the diffusion processes of such signals have mostly been elucidated. Post-contact, the M. pudica releases two types of signals which will affect the motor organ. Based on the intensity of the contact, M. pudica either propagates a rapid signal to the pulvini through electrical stimulation or long-distance chemical stimulation through exchanges with the xylem and phloem (Hagihara & Toyota, 2020; Snow, 1924).
Rapid signaling occurs when a contact is made with the pulvini of the plant, yet the plant's structure is not harmed (Hagihara & Toyota, 2020). These types of pulvini stimulations result in the cell generating an action potential (AP) which, in the case of M. pudica propagatesthrough electrical interaction through apoplastic pathways connecting the cell walls (Figure 9) (Hagihara & Toyota, 2020; Sukhov, et al., 2011). The effects of an AP and the propagation of the response requires further clarification. AP is the reaction where a sensory cell sends an electrical signal to its neighbouring cells, thus altering the cell membrane potential and stimulating specific channel proteins (Sukhov et al., 2011). Using electrophysiological measurements techniques and galvanometers, the process of inducing electrical signals throughout the petiole to recreate the effect of the action potential was proven to have a change in ion concentrations within the cells (Hagihara & Toyota, 2020). This electrical stimulation was proven to activate the various ion pumps, such as H+-ATPase, potassium ion pump, and chlorine ion pump, which actively transport ions from the cytosol to the apoplast, thus creating a potential difference between the cytosol and the extracellular matrix (Hagihara & Toyota, 2020; Sukhov et al., 2011). The biochemical importance lies in the charges and movement of the ions.
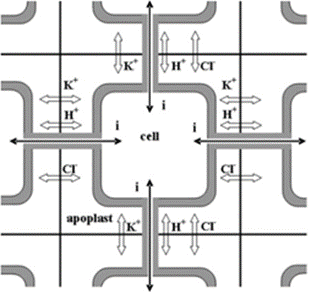
Long-distance signaling through the xylem and phloem occurs when the plant is significantly wounded, thus requiring the whole plant to receive the signal to act dead. Through applying various concentrated extract solutions to cut sections of the M. pudica stem, it was discovered that a chemical signal travels through the xylem vessels, transfers to the phloem, and signals the pulvini of its wound (Snow, 1924). The chemical in question is likely to be a form of Glutamate (Glu), an amino acid known for its signaling capabilities in plants and animals that have glutamine receptor-like proteins (GLRs) which trigger calcium ion (Ca2+) exchanges (Figure 10 & 11) (Qui et al., 2020). The production process of Glu takes place within the cytosol and the mitochondrion of the cell and is believed to be secreted throughout the conducting streams of the xylem and phloem (Figure 10) (Hagihara & Toyota, 2020; Snow, 1924). The role of Glu is to bind to the glutamate-gated Ca2+ channels present in the pulvini cellular membranes and stimulate the excretion of Ca2+ ions within the cell's cytosol (Forde & Lea, 2007). This change in ion concentration will be the main chemical exchange which results in the movement of the plant.
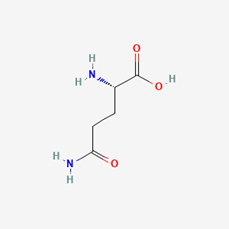
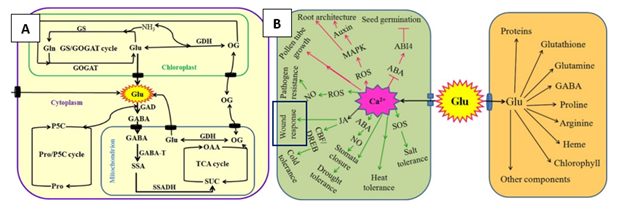
Therefore, both the rapid and the long-distance signalization techniques of the M. pudica utilize electrical and biochemical techniques, respectively, to control the movement of its leaves and stems to mimic a dead plant. Additionally, once the pulvini motor cells receive the threatening signals, they must go through a series of intercellular exchanges which ultimately physically alter the shape of the cell, thus moving the specific parts of the plant (Hagihara & Toyota, 2020).
Chemical Exchanges of Pulvini
Previously discussed in signal propagation, the motor cells receive different signals depending on the severity of the stimulus. In rapid signaling an electric current is felt and activates voltage-dependent ion channels, while in the long-distance signaling the Glu concentration in the xylem and phloem are increased to active Ca+-channels (Forde & Lea, 2007; Sukhov et al., 2011). While both signalizations are initially different, their effects are very similar.
In the case of rapid signalization, the AP activates the voltage-dependent potassium ion (K+) and chlorine ion (Cl-) channels (Hagihara & Toyota, 2020). The efflux of K+ from the cytosol results in a loss of positive charge, therefore, Ca2+ is brought into the cell from the extracellular matrix and from a tannin vacuole (TV) to balance the charge potential of the cell membrane (Hagihara & Toyota, 2020). Moreover, the influx of Ca2+ ions disrupt the cell membrane potential and trigger the activated Ca2+-dependent Cl—channels which aim to depolarize the cell membrane through efflux of Cl- ions (Hagihara & Toyota, 2020). Consequently, the efflux of K+ and Cl- ions and influx of Ca2+ in the cytosol results in the ion concentration of the cell becoming much lower than the apoplast, thus the cell becomes hypotonic compared to its environment (Hagihara & Toyota, 2020). Water molecules will travel through aquaporin channels and try to restore the tonicity of solutions, consequently, the pulvini cells will shrivel and lose their shape, hence the loss of volume will cause the motor cell to change shape and close on itself (Hagihara & Toyota, 2020). Therefore, the process of dehydrating the pulvini is controlled by the intracellular and extracellular exchanges caused by the AP (Figure 12).
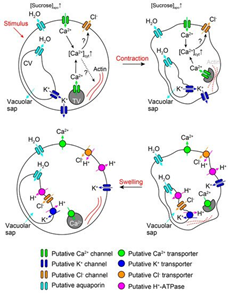
In long-distance signal recognition, the initiation of the ion transport is due to Glu activating Ca2+ channels (Forde & Lea, 2007). Like rapid signalization, the influx of Ca2+ creates a positive potential within the cell while also activating the Ca2+-dependent Cl—channels (Hagihara & Toyota, 2020). The efflux of Cl- and the influx of Ca2+ will repel the K+ ions from the cytosol, hence creating the same hypertonic solution for the cell (Hagihara & Toyota, 2020). Therefore, the result is the same as in rapid signaling and the exchange of molecules are extremely similar.
Conclusively, in both cases of signal recognition, the change in ion concentration forces plasmolysis due to the hypertonicity of the apoplast compared to the cell, thus changing the pulvini shape enough to create movement of the leaves (Hagihara & Toyota, 2020). Reactivating the ion channels and rectifying the proper solute concentration and composition of the cytosol works through the energy created by the efflux of H+ ions post plasmolysis (Hagihara & Toyota, 2020). When H+ ions are pumped out of the cell by H+-ATPase which adds positive charge in the apoplast and stimulates influx of K+ in the cell, moreover, ATP is partially used by the channel proteins to pump Ca+ back into the TV and the apoplast, hence reactivating the Cl- channels (Hagihara & Toyota, 2020). Furthermore, the readjustment of ion abundance will require water to obtain isotonicity, thus regaining turgor pressure and its original shape (Hagihara & Toyota, 2020).
Batesian Mimicry
A specific form of defensive mimicry is a phenomenon where a non-harmful species evolves to mimic the appearance of a species that is harmful to potential predators, known as Batesian mimicry. In other words, by appearing as the harmful species, the Batesian mimic benefits from the predator's learned avoidance behaviour. An extraordinary example of this Batesian mimicry in terrestrial animals is the glowing cockroach mimicking the bioluminescent toxic click beetle (Vršanský et al., 2012).
The Chemistry of Bioluminescence
First, it is important to understand the basics of bioluminescence and how it is propagated in the luminescent toxic click beetle of the genus Pyrophorus, as pictured in Figure 13below.
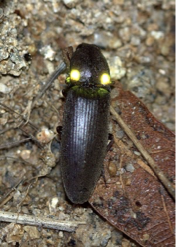
Bioluminescence is the production and emission of light in living organisms due to internal chemical reactions (Navizet et al., 2011). Light emission in this organism is a two-step process involving the chemical compound luciferin and the enzyme catalyst luciferase. In Figure 14 below, the scheme of light emission in the click beetle is displayed. The enzyme luciferase catalyzes the light production reaction by oxidizing the luciferin substrate, in the presence of ATP and Mg2+, to obtain an intermediate state called luciferyl adenylate. This is then oxidized with molecular oxygen to yield adenosine monophosphate (AMP), carbon dioxide and oxyluciferin, in its excited state (Wood et al., 1989). The subsequent relaxation of the excited oxyluciferin molecule to its ground state results in the emission of a photon, and thus the production of light. It is evident that bioluminescent organisms achieve the emission of light through purely chemical means, without requiring incident light to get an excited electron (Adams & Miller, 2020).

Bioluminescence Spectra of Click Beetles
Unlike other terrestrial bioluminescent organisms such as the firefly, luminescent beetles are able to change the emission spectrum of the light they produce. These different colours are not due to alterations in the substrates used in the bioluminescence chemical reactions, but rather a product of variation in the interaction of the substrates with the luciferase enzymes (Wood et al., 1989).
Shown in Figure 15 is the bioluminescence emission spectra of E. coli expressing the luciferase enzyme. The wavelengths of maximum intensity are 546 nm for green, 560 nm for yellow-green, 578 nm for yellow, and 594 nm for orange light. However, when the same bacteria were tested in different acidic conditions, the spectra showed a shift towards longer wavelengths at pH above 9, most notably for green light, as shown in Figure 16 (Wood et al., 1989). This demonstrates that at different acidic levels, the enzymes being proteins are able to change function. For instance, at higher pH levels the green-emitting luciferase is able to shift to emitting higher wavelengths of light, thus appearing as more of a reddish colour than blue. This allows the click beetle to emit different colours in varying acidic conditions that may be favourable to them in protection from predators; however, this is an idea experts have yet to explore (Hastings, 1966).
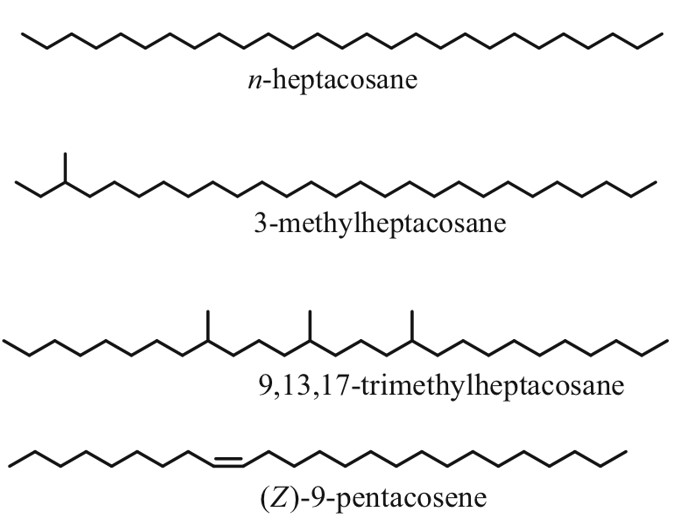
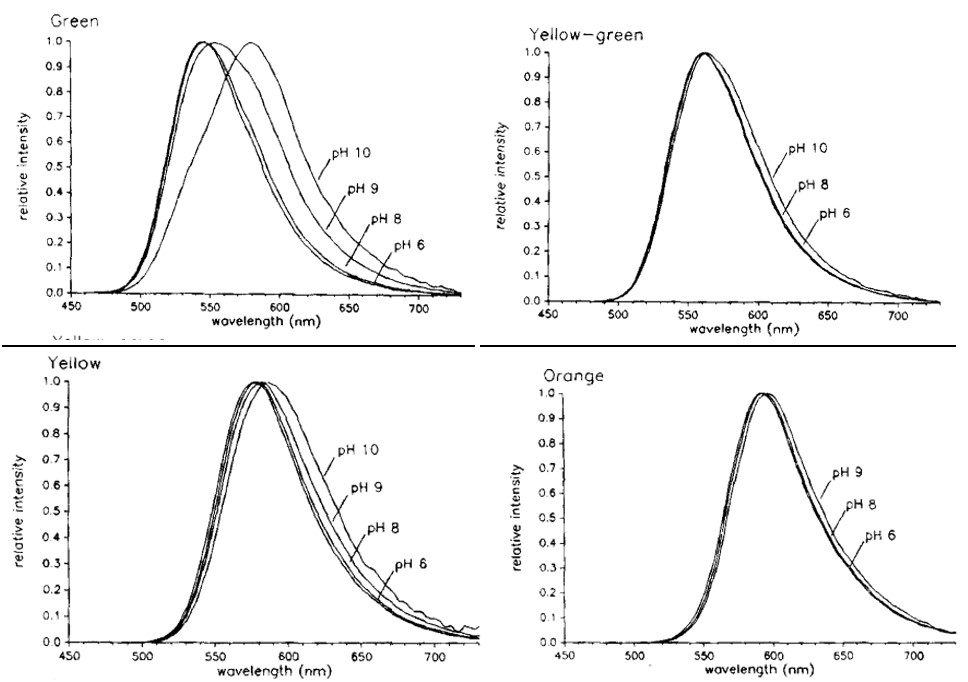
How does Bioluminescence serve the Click Beetle?
Now that the mechanism allowing for the click beetle's glow is understood, the question that remains is when and how do they utilise it? Bioluminescent toxic click beetles have two sets of light organs, one pair of lanterns located on the dorsal surface of the head, and a single lantern located in a cleft on the ventral abdomen. The light organs on the dorsal surface emit long pulses of green light when the beetle is not in flight, as a form of an aposematic signal to deter predators. During flight, the light organ set on the ventral surface emits a lime-green to bright yellow and orange light, and is involved in sexual communication between the species (Wood et al., 1989).
The dorsal lantern also lights up when the insect is disturbed, which is an attribute of the warning signal that click beetles present to potential predators. The click beetle produces toxic compounds that are fatal to predators so, as a way to deter predators and protect themselves, these beetles emit this bioluminescent green light. The toxic click beetle is most likely adapted to showing green as the aposematic, or repelling signal because most vertebrates and invertebrates have green receptors (Lall et al., 2010). Accordingly, potential predators can easily detect the green bioluminescence emissions and avoid preying on the toxic beetle.
The Glowing Cockroach- A Batesian Mimic
In 1999, luminescent cockroaches, Lucihormetica luckae, were discovered for the first time in the canopy forests of South America. Since bioluminescence in terrestrial organisms is so rare, these cockroaches were of great interest and are viewed as Batesian mimics of the toxic click beetle (Vršanský et al., 2012). Although cockroaches are more robust than beetles, they have a nearly identical structure of their luciferin protein and luciferase enzyme, an identical colour of light emission, and the same distance and locations of their lanterns.
As previously stated, the click beetle has lanterns located on the dorsal side of their head which flashes green to ward off predators. Similarly, these cockroaches have lanterns of identical size and location which emits the same green colour, as shown in Figure 17 (Vršanský et al., 2012).
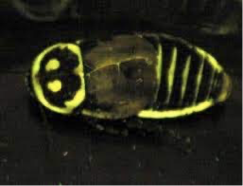
It is important to recall that the ability for the toxic click beetle to glow does not directly provide them with protection, but rather serves as a warning signal to deter unwanted predators. Therefore, by mimicking this green fluorescence, the cockroach tricks its predators into thinking that it is in fact the toxic click beetle as a means for survival.
Conclusion
Overall, chemical mimicry can take on many different forms and each organism uses this function to their own advantage. It may be in the form releasing pheromones to mimic another species' odor like orchid plants do for pollination purposes, copying chemical makeups like the Syrphid fly, or even by emulating physical appearances for survival purposes, a mechanism the Mimosa pudica plant and the Lucihormetica luckae beetle use. Through various signals and processes, these chemical mimics use this resemblance to their surroundings to successfully thrive in their environments.
References
Adams, S. T., Jr., & Miller, S. C. (2020). Enzymatic promiscuity and the evolution of bioluminescence. Febs j, 287(7), 1369-1380. https://doi.org/10.1111/febs.15176
Akino, T. (2005). Chemical and behavioral study on the phytomimetic giant geometer Biston robustum Butler (Lepidoptera: Geometridae). Applied Entomology and Zoology, 40(3), 497-505. https://doi.org/10.1303/aez.2005.497
Bagnères, A.-G. (2020). Cuticular Hydrocarbons. In C. K. Starr (Ed.), Encyclopedia of Social Insects (pp. 1-4). Springer International Publishing. https://doi.org/10.1007/978-3-319-90306-4_160-2
Bos, N., & d'Ettorre, P. (2012). Recognition of Social Identity in Ants [Mini Review]. Frontiers in Psychology, 3. https://doi.org/10.3389/fpsyg.2012.00083
d'Ettorre, P. (2016). Genomic and brain expansion provide ants with refined sense of smell. Proceedings of the National Academy of Sciences, 113(49), 13947-13949. https://doi.org/doi:10.1073/pnas.1617405113
Dettner, K., & Liepert, C. (1994). Chemical Mimicry and Camouflage. Annual Review of Entomology, 39(1), 129-154. https://doi.org/10.1146/annurev.en.39.010194.001021
Edwards, M. (2016). Andrena nigroaenea (Kirby,1802). Bees, Wasps & Ants Recording Society. Retrieved November 6, 2021, from https://www.bwars.com/bee/andrenidae/andrena-nigroaenea
Forde, B. G., & Lea, P. J. (2007). Glutamate in plants: metabolism, regulation, and signalling. Journal of Experimental Botany, 58(9), 2339-2358. https://doi.org/10.1093/jxb/erm121
Gaskett, A. C. (2011). Orchid pollination by sexual deception: pollinator perspectives. Biological Reviews, 86(1), 33-75. https://doi.org/https://doi.org/10.1111/j.1469-185X.2010.00134.x
Ginzel, M. D., & Blomquist, G. J. (2016). Insect Hydrocarbons: Biochemistry and Chemical Ecology. In E. Cohen & B. Moussian (Eds.), Extracellular Composite Matrices in Arthropods (pp. 221-252). Springer International Publishing. https://doi.org/10.1007/978-3-319-40740-1_7
Gomez-Diaz, C., & Benton, R. (2013). The joy of sex pheromones. EMBO reports, 14(10), 874-883. https://doi.org/https://doi.org/10.1038/embor.2013.140
Hagihara, T., & Toyota, M. (2020). Mechanical Signaling in the Sensitive Plant Mimosa pudica L. Plants, 9(5), 587. https://doi.org/10.3390/plants9050587
Hastings, J. W. (1966). The Chemistry of Bioluminescence. In D. R. Sanadi (Ed.), Current Topics in Bioenergetics (Vol. 1, pp. 113-152). Elsevier. https://doi.org/https://doi.org/10.1016/B978-1-4831-9969-6.50010-X
Howard, R. W., Stanley-Samuelson, D. W., & Akre, R. D. (1990). Biosynthesis and chemical mimicry of cuticular hydrocarbons from the obligate predator, Microdon albicomatus Novak (Diptera: Syrphidae) and its ant prey, Myrmica incompleta Provancher (Hymenoptera: Formicidae). Journal of the Kansas Entomological Society, 437-443.
Iwai, H., Horikawa, D. D., Arakawa, K., Tomita, M., Komatsu, T., & Maruyama, M. (2016). Rearing and observation of immature stages of the hoverfly Microdon katsurai (Diptera, Syrphidae). Biodiversity Data Journal(4).
Lall, A. B., Cronin, T. W., Carvalho, A. A., De Souza, J. M., Barros, M. P., Stevani, C. V., Bechara, E. J. H., Ventura, D. F., Viviani, V. R., & Hill, A. A. (2010). Vision in click beetles (Coleoptera: Elateridae): pigments and spectral correspondence between visual sensitivity and species bioluminescence emission. Journal of Comparative Physiology A, 196(9), 629-638. https://doi.org/10.1007/s00359-010-0549-x
Lunau, K., & Wester, P. (2017). Chapter Ten – Mimicry and Deception in Pollination. In G. Becard (Ed.), Advances in Botanical Research (Vol. 82, pp. 259-279). Academic Press. https://doi.org/https://doi.org/10.1016/bs.abr.2016.10.005
Mant, J., Brändli, C., Vereecken, N. J., Schulz, C. M., Francke, W., & Schiestl, F. P. (2005). Cuticular Hydrocarbons as Sex Pheromone of the Bee Colletes cunicularius and the Key to its Mimicry by the Sexually Deceptive Orchid, Ophrys exaltata. Journal of Chemical Ecology, 31(8), 1765-1787. https://doi.org/10.1007/s10886-005-5926-5
Millar, J. G. (2010). Chemical synthesis of insect cuticular hydrocarbons. In A.-G. Bagnères & G. J. Blomquist (Eds.), Insect Hydrocarbons: Biology, Biochemistry, and Chemical Ecology (pp. 163-186). Cambridge University Press. https://doi.org/DOI: 10.1017/CBO9780511711909.009
Monteiro, F., Sebastiana, M., Figueiredo, A., Sousa, L., Cotrim, H. C., & Pais, M. S. (2012). Labellum transcriptome reveals alkene biosynthetic genes involved in orchid sexual deception and pollination-induced senescence. Functional & Integrative Genomics, 12(4), 693-703. https://doi.org/10.1007/s10142-012-0288-x
National Center for Biotechnology Information. (2021). PubChem Compound Summary for CID 4525487, Glutamate. Retrieved November 6, 2021, from https://pubchem.ncbi.nlm.nih.gov/compound/Glutamate
Navizet, I., Liu, Y.-J., Ferré, N., Roca-Sanjuán, D., & Lindh, R. (2011). The Chemistry of Bioluminescence: An Analysis of Chemical Functionalities. ChemPhysChem, 12(17), 3064-3076. https://doi.org/https://doi.org/10.1002/cphc.201100504
Pereira, A. M., & Coimbra, S. (2019). Advances in plant reproduction: from gametes to seeds. Journal of Experimental Botany, 70(11), 2933-2936. https://doi.org/10.1093/jxb/erz227
Qiu, X.-M., Sun, Y.-Y., Ye, X.-Y., & Li, Z.-G. (2020). Signaling Role of Glutamate in Plants [Review]. Frontiers in Plant Science, 10. https://doi.org/10.3389/fpls.2019.01743
Schiestl, F. P., Ayasse, M., Paulus, H. F., Löfstedt, C., Hansson, B. S., Ibarra, F., & Francke, W. (1999). Orchid pollination by sexual swindle. Nature, 399(6735), 421-421. https://doi.org/10.1038/20829
Schlüter, P. M., Xu, S., Gagliardini, V., Whittle, E., Shanklin, J., Grossniklaus, U., & Schiestl, F. P. (2011). Stearoyl-acyl carrier protein desaturases are associated with floral isolation in sexually deceptive orchids. Proceedings of the National Academy of Sciences, 108(14), 5696-5701. https://doi.org/10.1073/pnas.1013313108
Snow, R. (1924). Conduction of excitation in stem and leaf of Mimosa pudica. Proceedings of the Royal Society of London. Series B, Containing Papers of a Biological Character, 96(678), 349-374.
Sukhov, V., Nerush, V., Orlova, L., & Vodeneev, V. (2011). Simulation of action potential propagation in plants. Journal of Theoretical Biology, 291, 47-55. https://doi.org/https://doi.org/10.1016/j.jtbi.2011.09.019
The Biology Project. (2003, August 25). Glutamine Q (Gln). University of Arizona. Retrieved November 6, 2021, from http://www.biology.arizona.edu/biochemistry/problem_sets/aa/Glutamine.html
Volkov, A. G., Foster, J. C., Baker, K. D., & Markin, V. S. (2010). Mechanical and electrical anisotropy in Mimosa pudica pulvini. Plant signaling & behavior, 5(10), 1211-1221.
Vršanský, P., Chorvát, D., Fritzsche, I., Hain, M., & Ševčík, R. (2012). Light-mimicking cockroaches indicate Tertiary origin of recent terrestrial luminescence. Naturwissenschaften, 99(9), 739-749. https://doi.org/10.1007/s00114-012-0956-7
Wood, K. V., Lam, Y. A., & McElroy, W. D. (1989). Introduction to beetle luciferases and their applications. Journal of Bioluminescence and Chemiluminescence, 4(1), 289-301. https://doi.org/https://doi.org/10.1002/bio.1170040141
Zschunke, P. (2007, April 23). Ophrys garganica. Encyclopedia of LifeThe https://eol.org/media/13713304