Superpowers in the Animal Kingdom
Yaman Al Janaideh, David Bettan, Kaitlyn Cribb, Vanessa Piché
Abstract
Navigating an unfamiliar environment can prove difficult for organisms that lack the necessary adaptations. Nevertheless, some animals possess unique senses that help them with the perception of their specific surroundings. Over many years of evolution, they have adopted ‘superpowers,' ranging beyond our five senses. These abilities aid their species in performing necessary tasks for their survival. In this paper, four different superpowers of four different animals are analyzed in detail, including the evolutionary advantages that are gained through the possession of these abilities. The four sections are: echolocation in bats, ultraviolet vision in fish, magnetism in migrating birds, and electroreception in elasmobranchs. First, the paper will explore the intricate process of echolocation in bats, focusing on its application in the hunt of prey. This active sensory system enables bats to ‘see' and avoid obstacles in the darkness, using their advanced auditory system to analyze echos. Then, how a species of reef fish, called the ambon damselfish, has been shown to utilize UV vision to their advantage. The extra electromagnetic spectrum perception allows them to see facial pattern markings on other fish, only perceivable through UV light. After that, the paper will cover how birds sense magnetic fields through the use of radical pairs. These pairs are formed by the cryptochrome molecule located in their retinas to determine direction when migrating. Finally, the paper will discuss how sharks and related species have a unique sensory organ, called the ampullae of Lorenzini, that allows them to detect very minute electric fields.
Introduction
The abilities of humans to perceive, understand, and respond to stimuli from their environments are limited by the five senses: sight, smell, hearing, taste, and touch. Over thousands of years, these five senses have evolved to perfectly suit the needs of humans, but there still exist many things in this world that are outside the realm of what humans can perceive. Since animals face different environmental pressures, for instance, having to hunt for food and hide from predators, they have, through evolution, developed special abilities or ‘superpowers' that are advantageous to their specific niches. Some general examples of animal superpowers include echolocation, magnetic sense, shapeshifting, UV vision, heat sensing, electroreception, and superstrength. In this essay, a few of these powers are discussed in more detail.
Echolocation in Bats
While most mammals can navigate through their environment, search for their prey, and identify different species through the use of their eyes, chiropterans (bats) must depend on their ears to ‘see.' As bats are nocturnal species that live in dark caves, they have poor exposure to light, thereby inhibiting their vision. Consequently, chiropterans adapted to their somber environments and lifestyle by using the process of echolocation to perform fundamental tasks.
How Does Echolocation Work?
There are three main steps in the process of echolocation, namely, emission, reflection, and reception. First, bats produce and emit high-frequency sound pulses through their mouth (larynx) or nose (nostrils) in a given direction. These emissions are typically ultrasonic sound waves at frequencies larger than 20 kHz, which surpasses the human audible frequency range of 20 Hz to 20 kHz (Kim, 2015). Hence, humans cannot hear the sound pulses emitted by bats. In terms of pitch, bats produce echolocation calls of constant frequencies (C.F.) and varying frequencies, referred to as frequency modulated (F.M.) sweeps. Chiropterans tend to use higher frequency C.F. and F.M. calls to gather more detailed data about their prey's range, direction, motion, size, and shape (Kim, 2015). Specifically, bats produce S.O.N.A.R. (Sound, Navigation, And Ranging) signals during echolocation, which helps them accurately examine their surroundings. Second, the emitted sound waves reflect off walls, obstacles, objects, or other creatures in the environment, which creates an echo that returns to the bat. Finally, the echoes are received by the bat's advanced auditory system and subsequently processed by the brain to generate an image of the analyzed surroundings (Kim, 2015).
Indeed, a bat's ears are finely tuned to the frequencies of the sound pulses it emits to recognize its own calls and thus perceive its environment. Multidisciplinary research suggests that bat ears were subject to auditory adaptations during evolution to improve their perception of their surroundings (Figure 1). Their advanced auditory system consists of a stapedius, outer hair cells surrounding the ear cartilage, three ossicles (malleus, incus, and stapes) in the ear canal, an eardrum, an elongated basilar membrane in the cochlea, and a high concentration of receptor cells in the inner ear which makes bat ears extremely sensitive to frequency changes (Davies et al., 2013). It follows that a bat's auditory system goes through a very intricate process when echolocating. First, the stapedius must contract to separate the three ossicles and reduce the bat's hearing sensitivity to prevent them from deafening due to their intense high-frequency calls. Then, the cartilage receives the echoed sound waves and funnels them into the ear canal. Afterwards, the sound pulses reach the eardrum, causing it to vibrate. These vibrations are then transferred to the basilar membrane in the cochlea. Each area of the membrane then creates unique vibrations in response to a particular frequency, which are converted into nerve impulses by the cochlea. Finally, the brain interprets the numerous nerve impulses and creates a mental map of its surrounding physical space (Davies et al., 2013). This active sensory system simultaneously occurs in both ears, allowing chiropterans to analyze the direction of specific objects or species. Therefore, echolocation enables bats to examine their surroundings and avoid obstacles in the darkness merely through their advanced auditory system.
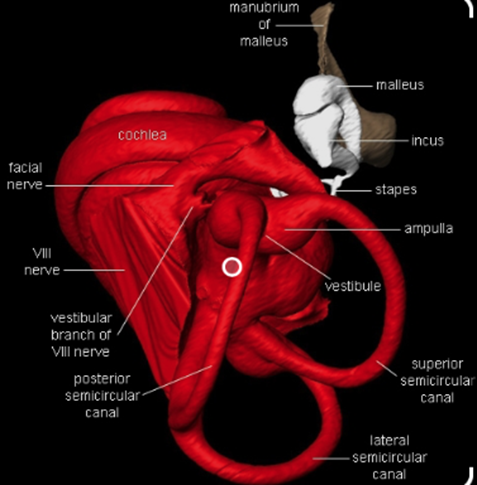
The Pursuit of Prey
Furthermore, bats use echolocation to search for and hunt their prey. Chiropterans are insectivores, and they primarily target small flying bugs, such as moths and mosquitos. In fact, certain bats can eat up to 1200 insects in an hour, which is significant in controlling insect populations (Simmons et al., 1979). To do so, bats rely on several factors during echolocation, namely, the range, direction, motion, size, and shape of their prey, to ensure that they successfully capture the correct insects (Figure 2).
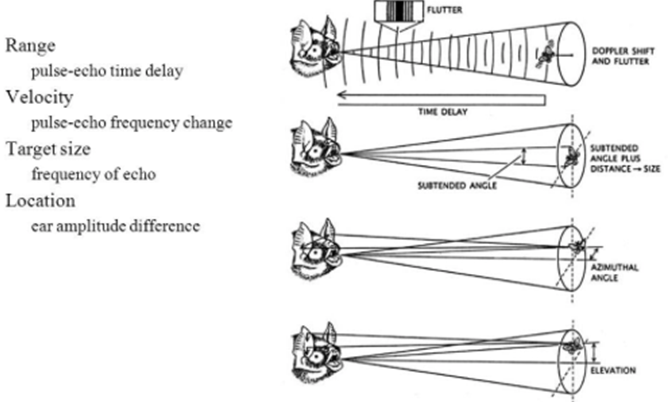
While the ability to perceive obstacles and creatures without using their eyes is impressive, studies show that bats can do as much as accurately pinpoint different species in their environment through a process called ranging. More specifically, as bats emit several ultrasonic calls, they can estimate how far their prey is located depending on how long it takes for the echoed sound waves to reach the bat's ears (Bohn, 1988). If an echo takes a long time to reach the source, the insect is far away. However, if the echoed sound waves quickly return to the bat's ears, the insect is near, and the bat should prepare to attack. This range-determining procedure can be generalized using the following formula: d = (Vₛ)·(𝚫t)/2, where d is the distance to the bat's prey, 𝚫t is the time it takes for the echo to return to the source, and Vₛ is the speed of sound. Clearly, as the time interval 𝚫t increases, the distance d increases proportionally. To improve the mental image of their physical environment, bats must also pay attention to which ear the echoed sound waves enter. Whichever ear receives the reverberation indicates the direction of the insect (Kim, 2015). Thus, the analysis of range and direction enables bats to precisely track down their prey.
Moreover, chiropterans can determine whether an insect is moving towards or away from them using the phenomenon known as The Doppler Effect (Kim, 2015). This theory refers to the change in wave frequency during the relative motion between a wave source and its observer. It follows that waves emitted by an observer moving towards the source get compressed, prompting a higher frequency. In the case of echolocation, if the insect moves towards or away from the bat, the resulting echoed sound waves will increase or decrease in frequency respectively, allowing the bat to adjust its attack plan.
In addition to determining the range, direction, and motion of their prey, bats can examine a creature's size based on the intensity of the reverberated sound wave. Chiropterans emit calls at intensities ranging from 50 to 120 dB; therefore, any change in intensity (loudness) between the bat's calls and the returned echoes enables them to infer the size of a species. Precisely, if the unidentified creature is smaller than the bat, the echoed sound waves will have a lower intensity than the sound pulses emitted by the bat and vis-versa (Kim, 2015). Using this information, bats associate lower intensity waves with their prey (moths or mosquitos) and higher intensity waves with their predators (hawks, owls, snakes, or raccoons) or other creatures. Once chiropterans analyze the creature's size, they determine its shape to create a better picture of the species. Myological studies show that bats have particular laryngeal muscles that can contract up to 190 times per second, allowing them to emit 160 to 190 chirps per second (Davies et al., 2013). The numerous sound waves emitted by the bat surround the creature in question, and each echoed wave returns data about the shape of the species. Bats can then differentiate between prey and predators based on the perceived shape of the creature (Schnitzler & Kalko, 2001). Thus, the various methods used to determine the range, direction, motion, size, and shape of a species allow bats to generate an accurate image of the creature they encounter and adjust their attack plan accordingly.
Now that bats have gathered all this information about their prey, they are prepared to systematically approach and attack them. First, bats emit low-frequency calls to search the area for local prey. Bats then navigate towards their prey using the previously explained echolocation factors and apply these notions to avoid obstacles and objects in their path. Once chiropterans are close to their destination, they emit up to 190 chirps per second to create a precise mental image of their prey and its surroundings. Next, bats slowly approach the insects at an angle that allows them to scoop their prey with their claws. Lastly, chiropterans fly away with the insects and consume them until more prey are located (Simmons et al., 1979).
The Effects of Environmental Conditions on Echolocation
While bats must already consider several factors when echolocating, changes in the environmental conditions of their habitat further complicate this self-locating process. For instance, since air pressure decreases when travelling at higher altitudes, temperature also decreases, which profoundly impacts a bat's echolocation. As molecules at lower temperatures experience less kinetic energy and vibrate slower, sound waves travel slower (Figure 3). Therefore, to adjust to the changing climates, chiropterans alter the frequency and intensity of their chirps. More specifically, to combat the decreased speed of sound at lower temperatures, bats increase the frequency and intensity of their ultrasonic signals (Goerlitz, 2018). Similarly, the atmospheric attenuation of sound in the air is affected by relative humidity (Figure 3). Since dry air is denser than humid air, and larger molecules require more energy to vibrate than smaller ones, the speed of sound decreases in dry air (Goerlitz, 2018). Another environmental condition that bats consider during echolocation is the direction of the wind, as it can affect the speed at which their signals travel. Precisely, if the wind is blowing in the opposite direction of the emitted sound waves, the wind will decrease the speed of sound. Thus, bats must increase the frequency and intensity of their calls to oppose unfavourable weather conditions (Goerlitz, 2018). Clearly, various environmental factors impact a bat's echolocating process.
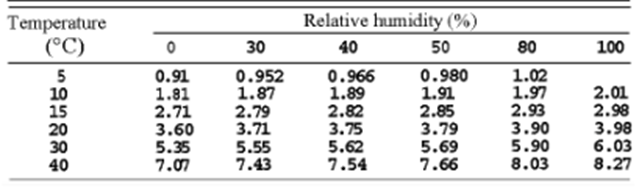
Ultraviolet Vision in Fish
The perception of the environment by different species, specifically, their visual perception, is dependent on the range of wavelengths included in the visible light spectrum of that species. For example, humans have a relatively narrow visible light spectrum, ranging from 400 to 700 nm; therefore, the human eye cannot absorb anything else, such as infrared and ultraviolet light, which are outside that range. Ultraviolet (UV) light refers to wavelengths lower than 400 nm, and the UV region of the electromagnetic spectrum ranges down to 10 nm wavelengths. This region consists of 3 bands or types of ultraviolet light: UVA (320-400 nm), UVB (280-320 nm), and UVC (10-280 nm). The solar radiation that reaches the Earth's surface is primarily UVA and UVB light, in addition to the human-visible light spectrum (Zamzov et al., 2008)
Ultraviolet Light in the Ocean
The ocean is perceived as being deep-blue in colour since water absorbs most of the longer wavelengths of the visible light spectrum, meaning reds and yellows, while reflecting the shorter ones, like blues and violets. However, water transmits shorter, ultraviolet wavelengths as well. Photons of ultraviolet light can travel up to 200 metres deep beneath the ocean's surface if the water is clear enough, which means that enough UV light can be provided for the vision of the animals that can perceive it, especially those that live in the more shallow parts of the ocean (Losey et al., 1999).
The retina inside the eye can perceive light signals from the environment because they contain two types of photoreceptors: rods, responsible for vision at low light levels, and cones, responsible for colour vision. The rods and cones of vertebrates, like fish, contain a group of proteins called opsins that are sensitive to light, so the absorption of light causes them to change conformations from their resting state to their signaling state. The ability to perceive UV light is dependent on the presence of cones that contain specific UV opsins with a of 360-380 nm, meaning that the strongest photon absorption occurs in that range of wavelengths. Fish can have up to 4 different types of cones, one of which can detect UV light, and the unique opsin molecule within this cone is highly conserved across different species of fish (Losey et al., 1999).
The Function of UV Perception in Fish
It has been shown through multiple studies that whether or not fish are sensitive to ultraviolet light, as well as their degree of sensitivity, tend to vary by species, as not all species possess this opsin. An indication that led researchers to believe that fish may perceive UV light is that many fish have UV pattern markings on their bodies. Furthermore, many of these markings that reflect ultraviolet light are located on their faces and fins, regions of the body that are mainly on display in behaviours where visual appearance is important, such as courtship, which suggests that the patterns play a role in social communication (Siebeck et al., 2001). This result would indicate that many fish, such as coral reef fish, which tend to have elaborate UV patterns, should be able to use this physiological advantage and therefore possess a sensitivity to ultraviolet light. (Siebeck et al., 2001).
UV Perception in Ambon Damselfish
A study that focused on ambon damselfish (Pomacentrus amboinensis), a species of reef fish found mainly in the Western Pacific, was done to determine whether these fish can use their UV pattern markings for species discrimination. These ambon damselfish are known to share their primary habitat with closely related species, namely, the lemon damselfish (P. moluccensis), and tend to react strongly to the intrusion of other fish because of the threat to their territory and resources. This resource-defending behaviour is especially shown towards conspecifics, those of the same species, rather than heterospecifics, those of other species, like the lemon damselfish, because conspecifics represent the most significant competition for food, space, and mates (Siebeck et al., 2001). Although these two species seem to be practically identical to the human eye, they each possess unique UV pattern markings in the face area (Figure 4).
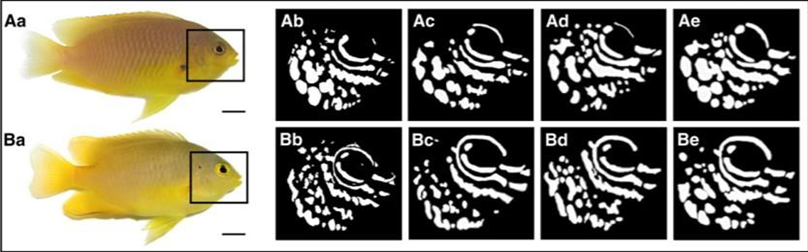
By measuring the preference of ambon damselfish to attack conspecifics versus heterospecifics under conditions of UV illumination, as well as conditions where a filter blocked UV light, they were able to show that the ambon damselfish can identify the species to which their intruders belong by analyzing the ultraviolet reflecting patterns on their faces. Their tests showed that the territory owners, the ambon damselfish, attacked their preferred intruder, their conspecifics, more frequently than the non-preferred intruder, the lemon damselfish, if the UV patterning was visible, but not if the UV filter blocked it (Figure 5). Thus, it appears that the presence of UV patterns is necessary for species discrimination (Siebeck et al., 2010).
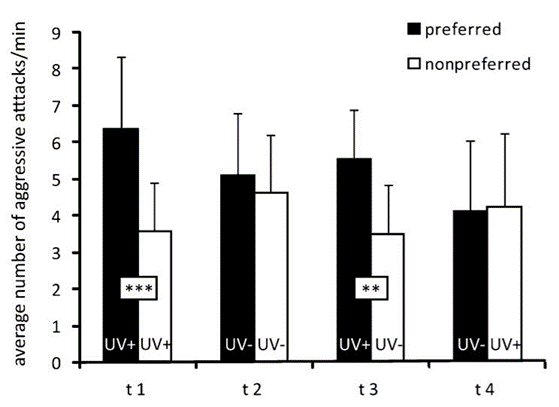
Further, to test whether the attacks were a response to the overall intensity of UV-reflecting facial patterns or the specific shapes of the patterns, another control condition was introduced in which the ambon damselfish were shown a non-preferred intruder presenting UV patterns and the preferred intruder not presenting such patterns, due to a UV blocking filter. Even in these conditions, the territory owner did not switch to attacking the non-preferred intruder simply because they were shown in the presence of UV light (Siebeck et al., 2010). As a whole, the experiments show that the ambon damselfish can use complex facial patterns to distinguish between conspecific and heterospecific fish, and they can do this while only using their UV-specific cones.
Another reason for which UV-based pattern recognition would be beneficial for the ambon damselfish is as a means of camouflage and communication while staying hidden. This fish species has a dominantly yellow body colouration (Figure 4), which allows it to be well-camouflaged in its predominantly yellow-brown reef habitat. Their facial UV patterns allow these fish to recognize and communicate with their own species without compromising their camouflage abilities (Siebeck et al., 2010). Additionally, the common predators of ambon damselfish, which include wrasses, coral trout, and rock cods, all possess lenses in their eyes that block UV transmission, similar to humans. For these predators, it is more beneficial to filter out UV light, and there are two main reasons for this. Firstly, exposure to UV light reduces contrast in their visual perception, similar to when humans develop cataracts. Thus, blocking UV light would enhance contrast, which is vital for prey detection, especially over long distances (Siebeck et al., 2010). Secondly, since UV exposure is damaging to the eyes, species with longer lives, such as these predators, would benefit from protecting their retinas. However, short-lived species, such as the damselfish, would not benefit as much since the costs of UV damage prove to be relatively small compared to all that they gain through UV light perception (Siebeck et al., 2010). Overall, this enhanced colour spectrum of vision improves their ability to protect their resources and to camouflage. Further research is being done regarding whether their advanced vision could also play a role in courtship and mating.
Magnetism in Migrating Birds
Every year, migratory birds, fueled by the desire for food or warmer weather, migrate across the globe. However, the question remains as to what natural “superpower” these animals possess that allows them to access this internal GPS. This phenomenon has been highly researched among the scientific community, often resulting in contrasting evidence and differing conclusions. The overall consensus, however, is that birds' natural sense of direction (partly) derives from their ability to sense magnetism and convert that to signals that inform them of their current position.
Magnetic Field
It is also important to specify that birds' magnetic dependence stems from the Earth's magnetic field rather than the magnetic dipole created by Earth's opposing poles. An experiment performed on European robins concluded that birds determine their direction by the axial inclination of the geomagnetic field (Wiltschko, 1972). They use the north-south axis as their north, much like how you set bearings on a compass, where the field lines and the gravitational vector form the smallest angle (Wiltschko, 1972). This phenomenon was proved by precisely altering the magnetic field in a testing area.
A study conducted on Northern Wheatears (a bird native to Europe) demonstrated the influence of the geomagnetic fields on birds' migratory patterns (Heyers et al., 2017). The population was placed in a magnetic field that was gradually changed to simulate the species' natural migratory pattern. By doing so, they noticed that the birds started to display less “migratory restlessness.” The term migratory restlessness is used to describe the natural urge for migratory birds to progress during their cycle. Another test was performed in which they kept the geomagnetic field resembling that of Northern Germany (their departure point) constant. The results showed that their migratory restlessness increased. This increase in restlessness was described as their reaction to the perceived lack of progress in their journey.
Although these case studies demonstrate their natural use of magnetism to determine flight paths, it does not necessarily indicate the specific ways they process and receive this magnetic information. The two phenomena often discussed and disputed in scientific literature are the concepts of the internal magnetic compass and magnetic mapping.
Internal Compass
Over the years, numerous theories about how and why birds can sense magnetism on Earth have been developed. The method that has been primarily researched is the use of an “internal compass.”
One theory explaining how birds operate this internal compass is through a radical pair mechanism (Worster et al., 2017). The molecule cryptochrome, a blue-light photoreceptor, is responsible for the formation of two radicals (flavin and tryptophan) (Worster et al., 2017). This molecule is believed to be responsible for magnetic sensation. These magnetoreceptors are believed to be located in the bird's retinas, where various cells are known to contain the cryptochrome molecule (Worster et al., 2017). The current theory suggests that the magnetic characteristics are detected through the change in the spin dynamics of the two photo-induced radicals caused by the field (a visual depiction of how birds see magnetic fields can be seen in Figure 6) (Worster et al., 2017).
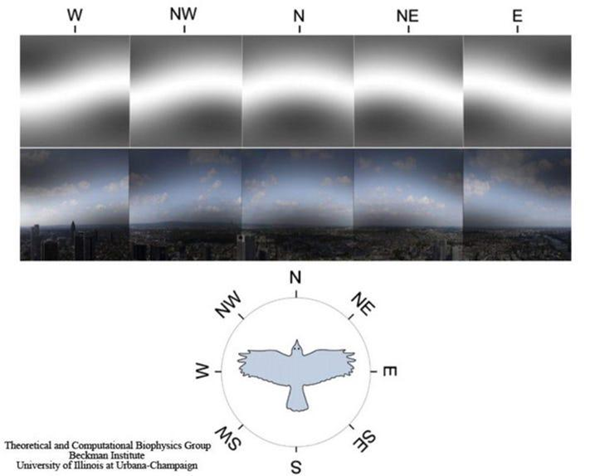
An experiment performed testing birds' responses to light gave significant evidence supporting the use of cryptochrome for magnetic signalling (Wiltschko & Wiltschko, 2001). In the experiment, they exposed birds to different forms of light (variating wavelengths). This study showed that blue-green light was required for correct orientation and found that the birds were disoriented under yellow light (Wiltschko & Wiltschko, 2001). In another study, they also found that birds were disoriented under the influence of red light (Pakhomov et al., 2017). Since cryptochrome is a blue-light receptor, if the birds were not exposed to blue light, they would not be able to photo-chemically produce their magnetic sensing radicals, therefore making the bird disoriented (as demonstrated in the results).
It was also found that the magnetic information was processed in the area of the brain named “Cluster N,” which is connected to the visual system (Pakhomov et al., 2017). They found that this area of the brain was highly active when nocturnal birds experienced migratory restlessness, unlike non-migratory birds (Pakhomov et al., 2017). When this area of the brain was lesioned, the birds would lose their ability to navigate using magnetic field cues (Pakhomov et al., 2017), indicating that their magnetic sensing abilities rely on their visual perception, further supporting the photo-radical theory.
Magnetic Mapping
Although birds can sense a general direction using this internal compass, it is also essential to consider how birds can tell this is the correct direction. The concept of “magnetic mapping” explains this succeeding concern. It is believed that their mapping abilities rely on the magnetic parameters (inclination, intensity and declination) of “grid lines” in Earth's magnetic field (Heyers et al., 2017).
Birds are believed to sample and store magnetic values along their migratory path. Initially, the use of magnetite (cluster of iron, depicted in Figure 7) was suggested as the way that birds can sense magnetic fields since they are strongly magnetic (Holland & Helm, 2013). The concentrations of iron found in the upper beak (both) of pigeons are connected to the central nervous system (Holland & Helm, 2013). Recent studies disprove its use as a magnetic compass; nevertheless, some researchers still believe it may play a role in magnetic mapping. A study done with juvenile and adult birds support this magnetic mapping sense. In their experiment, juvenile birds were not affected by the pulse; however, adult birds were (Holland & Helm, 2013), indicating that juvenile birds lack this experienced flight map. The idea of migratory restlessness and fuel deposition (the act of consuming more food in preparation for a nutrient-deficient environment) is indicative of this “mapping” concept (Heyers et al., 2017). Both of these concepts indicate process or position, implying the existence of a pre-mapped flight plan.
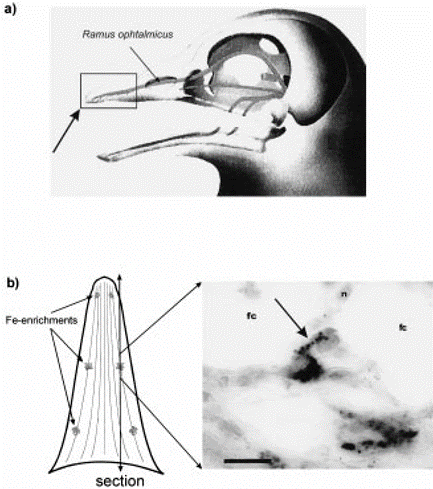
A magnitude of factors come into effect when analyzing how birds determine direction. For example, the use of landmarks and olfactory receptors are standard tools used to find their way. Birds have also been shown to use the sun and stars as directional guides. However, through the use of perception, they also employ both the use of a magnetic compass and a magnetic mapping system to fine-tune their migration program.
Electroreception in Elasmobranchs
One of the core drivers of evolution and natural selection is the idea of predator and prey. As such, a species' ability to navigate and sense its environment is quintessential for its survival. Many aquatic vertebrates have various sensory modes available to them as a tool for hunting and maneuvering. As a special case, elasmobranchs – in addition to the “usual” senses – have the ability to sense weak electric fields generated by other oceanic animals (Fields et al., 2007). They have a specialized sensory organ called the ampullae of Lorenzini (Fields et al., 2007), which allows them to sense electric field changes, such as those produced by their preys' muscle contractions (Zhang et al., 2018). Near and around their heads, sharks, skates, and related species have skin pores (Figure 8) that lead through insulated tubes to the ampullae (Fields et al., 2007). Serving as semiconductors, these tubes are filled with a gel that has a similar ionic makeup as the seawater the sensor is in (Bellono et al., 2018). Consequently, when a shark is close enough to a changing electric field, electricity is conducted through the tubes to the ampullae (Zhang et al., 2018), which is where the electric field is met with a thin layer of electrosensory cells, thereby triggering nerves that extend into the central nervous system (Bellono et al., 2017). Lastly, at the end of this pathway, the brain aligns the body with the field and processes the sensation of the field's producer.
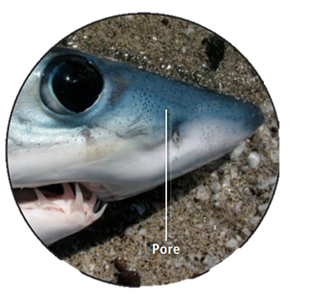
Voltage-gated Calcium ion channels
Research has shown that the electrosensory cells have a low threshold voltage-gated calcium channel, facilitating the cell's activity (Fields et al., 2007). When an electric field reaches the ampullae, it produces an electric potential across the receptor cell's apical membrane. There, calcium/potassium ion channels are activated (Fields et al., 2007), resulting in a calcium inflow and an outward counteractive potassium ion current (Figure 9) (Fields et al., 2007). Interestingly, it only takes 5nVcm-1 (Bellono et al., 2017) to activate the calcium channels in little skates and <.05 µV/cm-1 (Bellono et al., 2018) in sharks. Just a single nerve can detect electric fields almost eight times better than needed for orientation (Collin et al., 2000). This finding is believed to be due to the channels amplifying (Figure 10) the input voltage in a positive feedback loop (Collin et al., 2000). As the potassium ions flow out of the sensing cell (Fields et al., 2007) from the apical membrane (Collin et al., 2000), a current in the opposite direction of the original electric field is generated (Collin et al., 2000). Combined, the negative current and the original input flow through the basal receptor-cell membrane (Collin et al., 2000), where nerve activity is facilitated. The coupling of calcium/potassium ion channels causes the release of neurotransmitters at synapses with nerves that project to the brain (Modrell et al., 2017). As a note, it is evident that stronger fields lead to a faster firing rate of the stimulated nerves (Fields et al., 2007).
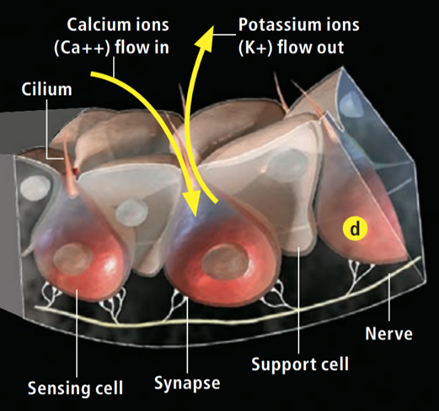
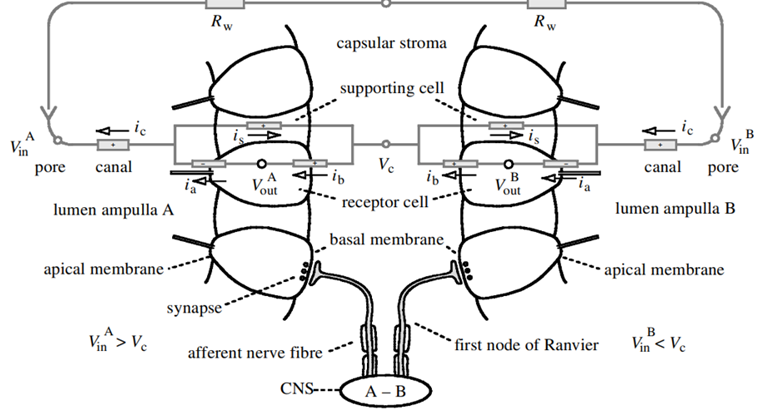
Electroreception Function
In general, the ampullae of Lorenzini allows for the detection of weak electric fields produced by prey, predators, and other living organisms. Due to different environmental and phylogenetic factors, some structural (Kempster et al., 2012) and behavioural differences arise. Thus, the function and use of electrosensory are tailored uniquely for each species and its environment. For instance, a shark's electroreception becomes its best asset when less than a meter (Fields et al., 2007) from its prey. The exact spatial information is revealed by which pores (Fields et al., 2007), and therefore ampullae, are activated. Naturally, with such a high sensitivity to electric fields, a lot of noise will be picked up alongside the prey's signal. Therefore, to block the noise, the signal is taken as an average over parallel ion channels and successive periods (Collin et al., 2000), allowing the jaw's precise orientation and alignment with its food.
Discussion
Through evolution and adaptation to their environments, different animals have developed unique abilities that are advantageous to their survival. Indeed, bats can perceive their surroundings and locate their prey despite being nocturnal creatures that live in dark caves. Chiropterans have finely tuned ears, permitting them to examine any alteration in the physical properties of the ultrasonic sound waves they emit compared to the echoes they receive. Using this collected data, bats create a precise mental map of their surrounding physical space. Moreover, the ambon damselfish possesses ultraviolet vision, allowing them to perceive specific UV facial pattern markings on conspecific and heterospecific fish. This means of species discrimination is evolutionarily advantageous as it aids them in defending their territory and resources. It was also shown how migrating birds have fine-tuned their ability to determine the direction they must travel in. This ‘superpower' is a byway of changes caused by the Earth's magnetic field, in the spin direction of the photo-chemically produced radical pairs located in their retinas. Finally, elasmobranchs possess a sensory organ called the ampullae of Lorenzini. This organ is lined with electrosensory cells, which facilitate nerve stimulation with the aid of low threshold voltage-gated calcium ion channels. Therefore, giving sharks and related species the ability to detect their prey's weak electric fields. As such, these findings demonstrate the vast array of perception modes available throughout the animal kingdom.
Conclusion
To conclude, several species have adopted ‘superpowers' through evolution to improve their perception of their unique environments. For instance, bats can ‘see' using echolocation, fish recognize their own species using UV facial pattern recognition, birds can determine direction when migrating using the Earth's magnetic field, and sharks can hunt using electroreception. These supernatural senses range beyond humans' traditional five senses, which is why researching these species is fascinating when discussing possible future technologies. Understanding how these abilities function opens up the opportunity to mimic and manipulate them.
References
Bellono, N. W., Leitch, D. B., & Julius, D. (2017). Molecular basis of ancestral vertebrate electroreception. Nature, 543(7645), 391-396. https://doi.org/10.1038/nature21401
Bellono, N. W., Leitch, D. B., & Julius, D. (2018). Molecular tuning of electroreception in sharks and skates. Nature, 558(7708), 122-126. https://doi.org/10.1038/s41586-018-0160-9
Bohn, D. A. (1988). Environmental Effects on the Speed of Sound. Journal of The Audio Engineering Society, 36, 223-231.
Collin, S. P., Marshall, N. J., & Kalmijn, A. J. (2000). Detection and processing of electromagnetic and near-field acoustic signals in elasmobranch fishes. Philosophical Transactions of the Royal Society of London. Series B: Biological Sciences, 355(1401), 1135-1141. https://doi.org/doi:10.1098/rstb.2000.0654
CSI Computerized Scanning and Imaging Facility. (n.d.). Big Brown Bat Cochlea 0001. Woods Hole Oceanographic Institution. Retrieved October 6, 2021, from https://csi.whoi.edu/landmammals/big-brown-bat-0001jpg-0/index.html
Davies, K. T. J., Maryanto, I., & Rossiter, S. J. (2013). Evolutionary origins of ultrasonic hearing and laryngeal echolocation in bats inferred from morphological analyses of the inner ear. Frontiers in Zoology, 10(1), 2. https://doi.org/10.1186/1742-9994-10-2
Fields, R. D. (2007). The shark's electric sense. Sci Am, 297(2), 74-80. https://doi.org/10.1038/scientificamerican0807-74
Fields, R. D., Fields, K. D., & Fields, M. C. (2007). Semiconductor gel in shark sense organs? Neurosci Lett, 426(3), 166-170. https://doi.org/10.1016/j.neulet.2007.08.064
Goerlitz, H. R. (2018). Weather conditions determine attenuation and speed of sound: Environmental limitations for monitoring and analyzing bat echolocation. Ecology and Evolution, 8(10), 5090-5100. https://doi.org/https://doi.org/10.1002/ece3.4088
Hanzlik, M., Heunemann, C., Holtkamp-Rötzler, E., Winklhofer, M., Petersen, N., & Fleissner, G. (2000). Superparamagnetic magnetite in the upper beak tissue of homing pigeons. Biometals, 13(4), 325-331. https://doi.org/10.1023/a:1009214526685
Hearing and echolocation. (n.d.). SlidePlayer. Retrieved October 6, 2021, from https://slideplayer.com/slide/4902507/
Heyers, D., Elbers, D., Bulte, M., Bairlein, F., & Mouritsen, H. (2017). The magnetic map sense and its use in fine-tuning the migration programme of birds. J Comp Physiol A Neuroethol Sens Neural Behav Physiol, 203(6-7), 491-497. https://doi.org/10.1007/s00359-017-1164-x
Holland, R. A., & Helm, B. (2013). A strong magnetic pulse affects the precision of departure direction of naturally migrating adult but not juvenile birds. Journal of The Royal Society Interface, 10(81), 20121047. https://doi.org/doi:10.1098/rsif.2012.1047
Kempster, R. M., McCarthy, I. D., & Collin, S. P. (2012). Phylogenetic and ecological factors influencing the number and distribution of electroreceptors in elasmobranchs. Journal of Fish Biology, 80(5), 2055-2088. https://doi.org/https://doi.org/10.1111/j.1095-8649.2011.03214.x
Kim, S. (2015). 7 – Bio-inspired engineered sonar systems based on the understanding of bat echolocation. In T. D. Ngo (Ed.), Biomimetic Technologies (pp. 141-160). Woodhead Publishing. https://www.sciencedirect.com/science/article/pii/B9780081002490000070
Losey, G. S., Cronin, T. W., Goldsmith, T. H., Hyde, D., Marshall, N. J., & McFarland, W. N. (1999). The UV visual world of fishes: a review. Journal of Fish Biology, 54(5), 921-943. https://doi.org/https://doi.org/10.1111/j.1095-8649.1999.tb00848.x
Modrell, M. S., Lyne, M., Carr, A. R., Zakon, H. H., Buckley, D., Campbell, A. S., Davis, M. C., Micklem, G., & Baker, C. V. (2017). Insights into electrosensory organ development, physiology and evolution from a lateral line-enriched transcriptome. Elife, 6. https://doi.org/10.7554/eLife.24197
Nace, T. (2018, April 4). We Finally Know How Birds Can See Earth's Magnetic Field. Forbes. https://www.forbes.com/sites/trevornace/2018/04/04/we-finally-know-how-birds-can-see-earths-magnetic-field/?sh=28e1635b20e1
Pakhomov, A., Bojarinova, J., Cherbunin, R., Chetverikova, R., Grigoryev, P. S., Kavokin, K., Kobylkov, D., Lubkovskaja, R., & Chernetsov, N. (2017). Very weak oscillating magnetic field disrupts the magnetic compass of songbird migrants. Journal of The Royal Society Interface, 14(133), 20170364. https://doi.org/doi:10.1098/rsif.2017.0364
Schnitzler, H.-U., & Kalko, E. K. V. (2001). Echolocation by Insect-Eating Bats. BioScience, 51(7), 557. https://doi.org/10.1641/0006-3568(2001)051[0557:ebieb]2.0.co;2
Siebeck, U. E., & Marshall, N. J. (2001). Ocular media transmission of coral reef fish — can coral reef fish see ultraviolet light? Vision Research, 41(2), 133-149. https://doi.org/https://doi.org/10.1016/S0042-6989(00)00240-6
Siebeck, U. E., Parker, A. N., Sprenger, D., Mäthger, L. M., & Wallis, G. (2010). A species of reef fish that uses ultraviolet patterns for covert face recognition. Curr Biol, 20(5), 407-410. https://doi.org/10.1016/j.cub.2009.12.047
Simmons, J. A., Fenton, M. B., & Michael, J. O. F. (1979). Echolocation and Pursuit of Prey by Bats. Science, 203(4375), 16-21. http://www.jstor.org/stable/1747535
Wiltschko, W., & Wiltschko, R. (1972). Magnetic compass of European robins. Science, 176(4030), 62-64. https://doi.org/10.1126/science.176.4030.62
Wiltschko, W., & Wiltschko, R. (2001). Light-dependent magnetoreception in birds: the behaviour of European robins, Erithacus rubecula, under monochromatic light of various wavelengths and intensities. J Exp Biol, 204(Pt 19), 3295-3302. https://doi.org/10.1242/jeb.204.19.3295
Worster, S., Mouritsen, H., & Hore, P. J. (2017). A light-dependent magnetoreception mechanism insensitive to light intensity and polarization. Journal of The Royal Society Interface, 14(134), 20170405. https://doi.org/doi:10.1098/rsif.2017.0405
Zamzow, J. P., Nelson, P. A., & Losey, G. S. (2008). UV Lights Up Marine Fish. American Scientist, 96(6). https://doi.org/10.1511/2008.75.482
Zhang, X., Xia, K., Lin, L., Zhang, F., Yu, Y., St. Ange, K., Han, X., Edsinger, E., Sohn, J., & Linhardt, R. J. (2018). Structural and Functional Components of the Skate Sensory Organ Ampullae of Lorenzini. ACS Chemical Biology, 13(6), 1677-1685. https://doi.org/10.1021/acschembio.8b00335