Abstract
Although tardigrades were discovered in the late 18th century, they have continued to captivate scientists due to their outstanding resistance to environmental conditions that are lethal to most organisms and that is necessary given their ecological distribution across various environments. Explanations as to the mechanisms underlying this superior resistance have been proposed and are all linked to the tardigrades’ ability to enter a so-called tun state in which they shrivel, contract, and undergo a near-complete dehydration (anhydrobiosis) and interruption of their metabolism (cryptobiosis). Such state enables, in short, certain proteins called TDPs (tardigrade disordered proteins) to adopt spatial arrangements that are thought to be substantially involved in tardigrades’ resistance to external as well as internal physical constraints, resulting from that state, which also requires further physical adaptations that are described here). Moreover, tardigrades exhibit an uncommon way of locomoting relatively to organisms of their small size and have adapted to adjust their gait appropriately to the substrates they encounter while migrating.
Introduction
Tardigrades (phylum Tardigrada), also known as “water bears” or “moss piglets,” are small invertebrates, and close relatives of arthropods, ranging from 0.05 mm to 1.2 mm in body length (Macdonald, 2004). They were first discovered in 1773 by Johann August Ephraim Goeze who gave them the name “water bears” during his initial observation due to the fact that their movements resemble those of a bear. Three years later, the name Tardigrada (meaning “slow stepper”) was bestowed upon them by Lazzaro Spallanzani (Macdonald, 2004). These titles reflect the tardigrade’s unique collection of locomotor mechanisms, enabling them to move through a vast array of environments by walking, despite their microscopic and soft-bodied characteristics.
The tardigrade’s body is divided into two main parts: the head region and the body region, composed of fused segments. The organism’s head is equipped with a tubular mouth encircled by stylets, which are employed to puncture plant cells, algae, and other living organisms on which the tardigrades feed. Inside the mouth, there is a triradiate (muscular pharynx that opens into a short tubular esophagus) that continues down to the intestine and that constitutes most of the length of the organism. The tardigrade’s body is a hemocoel filled with fluid that transports oxygen and water to different parts. Aattached to their bodies are four pairs of legs, each ending in four to eight claws. Tardigrades reproduce either sexually or asexually depending on their species (Weronika, 2017).
These microscopic animals are said to be polyextremophilic; indeed, they are ubiquitous since all four classes of tardigrades – Heterotardigrada, Eutardigrada, Mesotardigrada, and Apotardigrada, thrive in a variety of ecosystems, including intertidal zones, freshwater bodies, limnoterrestrial zones, deserts, urban areas and ice niches in Arctic (Reyes et al., 2023). However, tardigrades are perhaps most well-known for their ability to survive environments distinguished by their extreme nature. Many species of tardigrade are forced to reckon with hostile environmental conditions including highly fluctuating conditions, rapidly changing humidities, extreme temperatures, and un-navigable substrates (as compounded by their microscopic scale). This means it has been necessary for them to develop various solutions to such conundrums. Although surviving in extreme environments poses challenges, it is also advantageous for tardigrades in allowing them to fill an ecological niche that is not highly competitive. For instance, tardigrades face less interspecies competition for resources and are also subject to a lower predation rate in these environments. In essence, there is a high initial cost for tardigrades to adapt to this environment, but the advantages conferred later make this cost ultimately worthwhile.
Tardigrades’ astonishing adaptability across diverse ecosystems and resistance to harsh environmental conditions makes them one of nature’s most exceptional creatures. For instance, they can survive when exposed to lack of oxygen, water scarcity, extreme temperatures (-200ºC to 151ºC), andradiation (Macdonald, 2004). A central factor behind these abilities is a process known as the tun state – a survival strategy in which they suspend and shut down their metabolic activity. Anecdotally, the European Space Agency decided to send two tardigrade species (Richtersius coronifer and Milnesium tardigradum) to space at low Earth orbit during the FOTON-M3 mission. The samples were exposed to space vacuum and to UV radiation and survived (Jönsson, 2008). Furthermore, it must be noted that tardigrades’ unusual way of locomoting is also behind many aspects of their high adaptability; it is another design solution developed relatively to challenges arising from their ecosystems’ variability.
Resistance to extreme conditions is not characteristic of all species of tardigrades; indeed, those that are found in highly stable environments, such as stable aquatic environments, had no pressure to evolve that way contrarily to those found in damp flora or moss microenvironments whose conditions are subject to high variability (Miller, 2011). Bryophytes (land plants) offer only a thin layer of protection and are prone to rapid drying as direct ultraviolet exposure varies and to great variations in temperature, humidity and substrates, hence the need for adaptations to extreme fluctuations even in unextreme habitats.
Though the mystery behind the tardigrade’s incredible adaptability stood unresolved for centuries, research of the last fifty years has in fact identified several key elements in their physiology that are critical to their characteristic resilience, enabling the scientific community to better understand the biological mechanisms underlying their behavior.
The Function of Tun Formation in Anhydrobiosis
The ability of terrestrial tardigrade species, as well as several marine species, to undergo cryptobiosis and enter an inactive and nearly lifeless state is undoubtedly one of the most unique features within the animal kingdom. This characteristic feature is an important factor in many tardigrades’ capacity to withstand extreme environmental conditions and survive over extended periods of resource scarcity. In tardigrades, the process of cryptobiosis is accompanied not only by a radical reduction in metabolic rate, but also often by a period of rapid desiccation through which the tardigrade becomes almost entirely dehydrated. In fact, some species of tardigrade have been shown to lose up to 98% of their water content during anhydrobiosis (Crowe, 1972). During the process of anhydrobiosis, it has been observed that most species of tardigrades contort into a position known as “tun formation,” wherein the body undergoes longitudinal contraction, and intersegmental areas are folded inwards (Clegg, 2001). Such positioning is crucial to the mitigation of rapid evaporative water loss. When desiccation occurs at too rapid a rate, however, the tardigrade’s revival rate diminishes greatly. This suggests that, since tun formation is an important factor in slowing the rate of transpiration, it is critical to survival following rehydration.
The Tardigrade Cuticle
The physiological structure of the tardigrade bears numerous similarities to arthropods, which are considered to be their closest living relatives. For instance, like arthropods, much of the tardigrade integument is formed by a cuticular structure that provides a layer of protection from external elements in the environment. This structure varies in thickness throughout the body, according to locomotive, ovipositional, and feeding stresses (Czernekova and Vinopal, 2021). In addition, the thickness of the cuticle is directly correlated with its permeability. In John H. Crowe’s study on the permeability of different regions of the tardigrade cuticle, dyes were used to differentiate between regions of varying cuticular thickness. According to Crowe, “the dye penetrated the thin portions of the cuticle within a few seconds, but it penetrated the thicker portions extremely slowly” (1972). Crowe further theorizes that, since thinner regions of the cuticle are far more permeable, they are particularly vulnerable to water loss.
The tardigrade has the thinnest cuticular layers in the intersegmental regions – i.e., the regions between the head and four leg-bearing segments. The cuticle in these areas is measured at only one-twelfth the thickness of the cuticle over much of the rest of the body (Wright, 1989). As expected, experimental data demonstrates that these intersegmental regions of the tardigrade are some of the most permeable areas of the body and are consequently highly susceptible to water evaporation (Crowe, 1972). As a result of this vulnerability, adaptative functions that allow the tardigrade to reduce the surface area of thinner, more permeable cuticle exposed to the surrounding environment are highly advantageous, allowing the tardigrade to dehydrate at a rate that promotes future chances of revival.
Tun Formation
The major adaptation that aids in reducing rapid evaporative water loss is tun formation, depicted in Fig. 1 and Fig. 2. Entering tun formation is a complex anatomical process for the tardigrade, involving “retraction of the lobopodia, infolding of the intersegmental cuticle, and a degree of longitudinal infolding” (Wright, 1989). Essentially, the tardigrade contracts in the anterior-posterior direction and its limbs invaginate, resulting in an 87% reduction of bodily volume in eutardigrade Richtersius coronifer for instance (Halberg et al., 2013).

Figure 1 (left): Scanning electron micrographs of extended (2A) and partially contracted (B) tun state tardigrades.
(right): Scanning electron micrographs of tardigrade tuns in dorsal aspect (1A) and ventral aspect (B) (Wright, 1989).
This bodily contraction allows the tardigrade to retract the intersegmental areas prone to transpiration into the body, protecting them from the surrounding environment and reducing exposed cuticular surface area. In fact, in the experiments performed by Halberg et al., it was observed that the process of contraction in the tun formation is “initiated when the animal senses a cue associated with change in external water potential” (2013). Rather than a passive byproduct of anhydrobiosis, the tun state is actively initiated in environments ripe for cryptobiosis, suggesting that this formation is an adaptive function aiding in the dual processes of anhydrobiosis and cryptobiosis.
The Relationship Between Surface Area and Desiccation
Experimental data further reveals the relationship between reduced surface area during tun formation and increased revival rates following rehydration. For instance, in Crowe’s “Desiccation Tolerance and Water-Retentive Mechanisms in Tardigrades,” the relationship between surface area and desiccation tolerance is measured (Fig. 3). The tardigrade is estimated as a hemicylinder, with length “measured from the anterior tip to the junction between the posterior lobopodia” and width measured as the “mean of the intrasegmental regions of … the first, second and third pairs of lobopodia” (1989).

Figure 2: Graph of surface area reduction vs desiccation tolerance of tardigrade specimens. The graph demonstrates a strong positive linear relationship between the two variables (Wright, 1989).
Disregarding the outlying E. testudo specimen, whose cuticular thickness and inflexibility is unrepresentative of general tardigrade morphology, the results displayed in Fig. 3 demonstrate an upward trend in desiccation tolerance as surface area reduction increases, i.e., as surface area decreases. The Pearson correlation coefficient of 0.82 suggests a strong linear relationship between desiccation tolerance and SA reduction, indicating that surface area reduction as determined by tun formation is a crucial factor in increasing a specimen’s tolerance to dehydration. Therefore, Wright’s data demonstrates that entering the tun state allows the tardigrade to prevent rapid water loss by reducing the exposed surface area, essentially enabling a “controlled rate” of desiccation that greatly improves chances of revival.
The Effect of Humidity on Tardigrade Revival
Tun formation is especially critical in the lower humidity states of higher altitude environments, which many tardigrade species inhabit, where evaporation occurs at an even higher rate. It has been demonstrated that, at radically low humidity levels, the tardigrade reaches almost complete desiccation within an hour and does not even have adequate time to enter tun formation (Crowe, 1972). At 25-30% relative humidity (RH), for instance, the tardigrades “are unable to form tuns during such rapid dehydration and remain in an extended posture after drying; they do not revive on subsequent rehydration” (Wright, 1989). Though reflecting a more extreme environment, this data suggests that surface area reduction is especially critical in lower humidities, where revival rate plummets due to rapid evaporation.
In fact, at slightly more elevated humidity levels, when tardigrade specimens can form tuns and reduce their surface area, the evaporation rate slows dramatically. In his experiment, Crowe observed that below about 50% RH, tardigrade specimens were reduced to 2-3% of their water content in a very short period of time and generally could not form tuns (1972). From Fig. 4, for instance, tardigrades in 0% RH took about 15 minutes to reach near-desiccation, while tardigrades at 30% RH took 90 minutes. However, tardigrade specimens at 50% RH took nearly forty hours to become desiccated and, above 60%, no tardigrade was dehydrated by more than 93%. At 70% RH, in fact, it took 10 hours before the tardigrades were even reduced to half of their initial water content.

Figure 3: Graph of tardigrades’ water content (%H2O) over time. %H2O is calculated using the formula %H2O = (Wt − Wd) ∕ Wt × 100, where Wt = weight at time t and Wd = dry weight. The slope represents the evaporative rate experienced by the tardigrade specimens (Crowe, 1972).
Moreover, Crowe demonstrated in his study that tun-forming tardigrades lose water at a far slower rate than non-tun forming tardigrades exposed to the same humidity (1972). It was observed that, while unaltered specimens at 60%, 70%, 80%, and 95% RH were able to form tuns and decrease their permeability, anesthetized tardigrade specimens at 80% RH (i.e., tardigrades prevented from forming tuns) saw little to no decrease in their permeability coefficient over time, meaning the evaporative rate was unchanged and the tardigrades lost their water content rapidly (Fig. 5). In fact, the behavior of the anesthetized tardigrade at 80% RH was almost identical to the tardigrade at 30% RH, further supporting the fact that inability to enter tun formation is a critical factor in rapid dehydration at lower humidities.

Figure 5: Graph representing tardigrades’ permeability coefficient over time at varying humidity levels, with anesthetized and non-anesthetized specimens (Crowe, 1972).
Overall, Crowe’s data demonstrates that tun formation is highly effective at reducing the evaporation rate in lower humidities, allowing the tardigrade to dehydrate at a controlled rate. Thus, without tun formation, the tardigrade’s chance of revival following anhydrobiosis is greatly reduced at low humidities.
In summary, tun formation allows the tardigrade to retract the permeable cuticle of its intersegmental regions and slow the rate of evaporative water loss in a wide range of humidities, thus allowing the body to enter cryptobiosis with a high chance of revival following rehydration. It is an immensely advantageous physical adaptation, enabling the tardigrade to safely enter a state where it can “pause” its metabolism during periods of resource scarcity and, as a result, avoid death due to starvation. Ultimately, tun formation is crucial to the tardigrade’s survival strategy, and is a remarkable example of the diverse physical solutions observable throughout nature.
The Tardigrades’ Unsurpassed Mechanical Resistance
Notwithstanding the fact that tun formation proves to be a highly successful adaptation among tardigrades for surviving harsh environmental conditions, which turn out to be fatal for most organisms, the very existence of this mechanism presents other challenges to them. Indeed, desiccation comes not without physical constraints; inter alia,desiccated cells undergo deformative forces that are attributable to their shrinkage during dehydration (Krakowiak et al., 2023). Thus, complementarily to tun formation, tardigrades must develop strategies to survive this state, which is essential for their relative invulnerability to extreme habitats and, consequently, for their population’s wide distribution. Cytoplasmic-abundant heat-soluble (CAHS) proteins varied architecture, for instance, falls within those adaptations (Tanaka, 2022). It has also been demonstrated that tardigrades are remarkably resilient to high hydrostatic pressures (Ono, 2008) as well as to high-speed impacts while being in the cryptobiotic state (Traspas, 2021). Though no direct explanation seems to have been studied or put forward as to these latter observations, it can rationally be speculated that some mechanisms correlated to an improved resistance in the tun state are also involved in tardigrades’ resistance to such environmental pressures.
Trehalose as an Initial Insufficient Hypothesis
Since it is known that the vitrification of trehalose inside the cytoplasm is a mechanism that allows some species – notably some chironomids’ larvae, brine shrimps’ cysts and Selaginella’s vegetative tissues, to cope with desiccation, the same assumption was initially put forward regarding tardigrades (Krakowiak et al., 2023; Yagi-Utsumi et al., 2021). Such convergent evolutive adaptation in which trehalose, at a high concentration, condensates in a glass-like matrix upon anhydrobiosis would contribute to physically hinder biomolecules’ denaturation inside the cell by immobilizing them and by replacing water in forming hydrogen bonds with them (Krakowiak et al., 2023; Sanchez-Martinez et al., 2023; Yagi-Utsumi et al., 2021). However, quantities of trehalose yielded by tardigrades vary greatly across species and have always proven to be inferior to those found in other animals. Moreover, not all tardigrades possess the gene associated with the enzyme responsible for its production (Boothby et al., 2017; Hesgrove & Boothby, 2020; Kasianchuk et al., 2023; Sanchez-Martinez et al., 2023 ; Yagi-Utsumi et al., 2021). Thus, trehalose vitrification surely is not the sole mechanism that underlies tolerance to anhydrobiosis, not mentioning that trehalose vitrification does not permit to explain how tardigrades resist deformative forces caused by their shrinkage (Krakowiak et al., 2023).
Discovery of Unique Tardigrade Disordered Proteins (TDPs)
Tardigrade-specific disordered proteins (TDPs) are currently pointed at for what comes to desiccation tolerance (Malki et al., 2022). These comprise cytoplasmic, secreted, and mitochondrial abundant heat proteins, respectively CAHS, SAHS, and MAHS, which are all highly distinctive from other proteins encountered in other organisms. Though they all contribute to tardigrades’ resistance to desiccation, their mechanisms differ (Kasianchuk et al., 2023). CAHS proteins allow for the protection of the intracellular content, CAHS proteins contribute, for example, to the protection of the intracellular content, forming an encompassing structure reminiscent of a cell wall, which prevents complete collapse upon desiccation, and MAHS participate in the protection of the mitochondria (Hesgrove & Boothby, 2020). Yet, CAHS proteins’ physical role has been studied more extensively and will be discussed more here.
CAHS Proteins Spatial Arrangement as a Design Solution
In the search for cellular components allowing protection against the mechanical stress that tardigrades are subjected to when transitioning to the tun state, one team of scientists isolated three distinct proteins of the CAHS family by precipitation, which are CAHS12, CAHS3 and CAHS8, using trifluoroethanol, a desolvating agent that contributes to the precipitation of solubilized proteins by impeding aqueous solvation (Tanaka, 2022). According to the team’s reasoning, such proteins would also condensate in a dehydration-like state and, therefore, would possibly contribute to tardigrades’ protection in that condition. In fact, it is the conformation of these CAHS proteins that counteracts deformative forces undergone by the cells rather than biochemical transformations occurring inside them (Krakowiak et al., 2023; Malki et al., 2022; Tanaka, 2022).
Configuration of CAHS Proteins in Function of Hyperosmotic Stress
When a cell containing CAHS proteins is exposed to a mechanical stress caused by an important loss in water, these are observed to change conformation. To visualize such structural changes, human cultured HEp-2 cells separately expressing these proteins in a fluorescent form (fused with the green fluorescent protein, GFP) have been examined, by Tanaka’s team, in a concentrated solution of trehalose which revealed to lead to the same effects as any other solute. The hyperosmotic stress that cells in the concentrated solution were subjected to, induced a water efflux – and thus deformative forces – similarly to the dehydration stress occurring in tardigrades in cryptobiosis.
In an isosmotic environment, that is an unstressed condition, all three proteins were found throughout the cytosol, within the nucleus or both, and none showed any arrangement whatsoever. However, in a hyperosmotic solution, the CAHS proteins exhibited different formations, as shown in Fig. 6:
- CAHS3-GFP condensed and formed a filamentous network in the cytosol.
- CAHS8-GFP condensed in granules located in the nucleus but formed less filaments.
- CAHS12-GFP behaved similarly to CAHS3-GFP, though most filaments were found in the nucleus and though it also condensed, to a lesser extent, in granules.
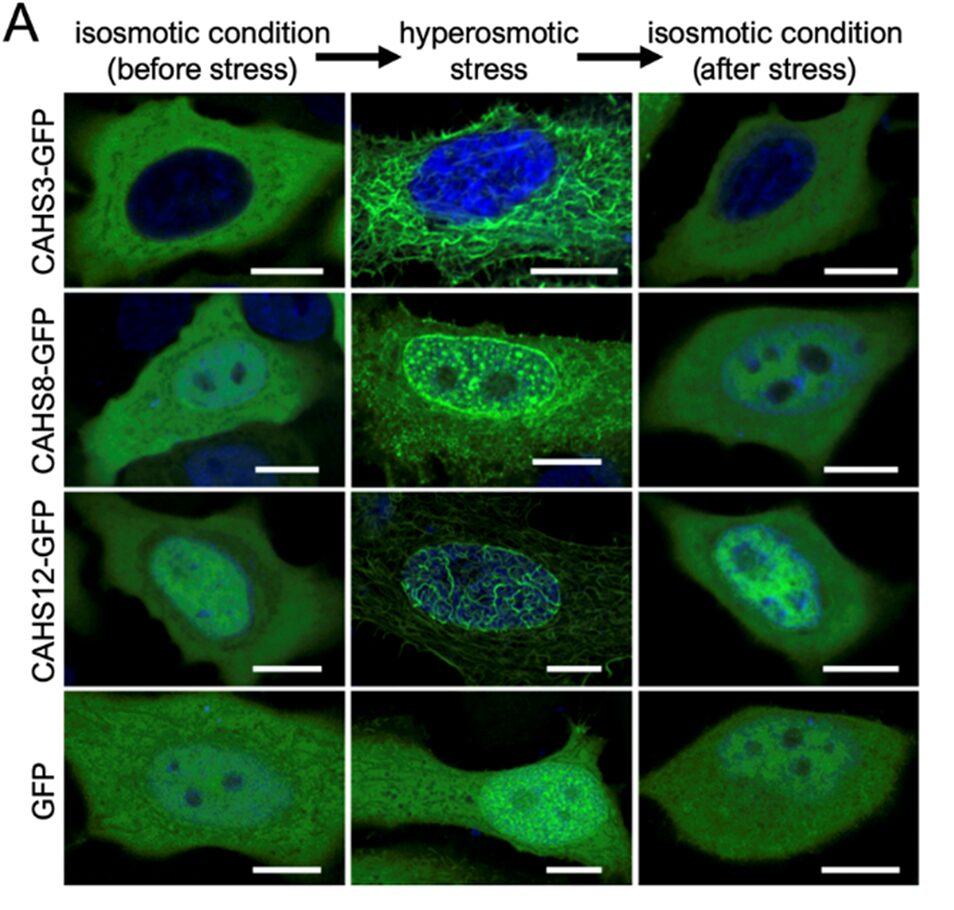
Figure 6: Alteration of CAHS proteins’ structure depending on the osmotic condition (Tanaka et al., 2022). It must be provided that the green fluorescent protein alone did not change arrangement when cells expressing it were transferred to a hyperosmotic solution.
Such behavior was also observed in the absence of the GFP, with different osmolytes of equivalent osmolarity and in non-human cells (pertaining to Drosophila), therefore suggesting that the latter are not involved in CAHS proteins reorganization (Tanaka, 2022). CAHS8 granules have been characterized by Tanaka’s team as partly liquid like, since 1,6-hexanediol, a disruption reagent of liquid-like condensates, dispersed with limited effectivity, whereas, in contrast, CAHS3 and CAHS12 filaments have been characterized as static and solid because the same reagent had no effect on them. Moreover, these filaments, whose structure is closely related to a cell’s cytoskeleton, have been shown to be independent from actin filaments given that actin polymerization inhibitors had no effect on the formation of the filaments despite a shared slight colocalization. As it is also depicted in Fig. 6, CAHS proteins returned to an uncondensed state after the mechanical stress was relieved, which was, in the context of that experiment, caused by a hyperosmotic environment, therefore making this process known to be reversible.
The CAHS proteins adopted the discussed conformation upon any dehydration-associated stress other than the exposition to a hyperosmotic environment; the addition of trifluoroethanol (a desolvating agent) also forced the changes in question, meaning that no biochemical processes are required for them to occur.
Effects of CAHS Proteins’ Conformation on a Cell’s Mechanical Properties
The presence of CAHS3 proteins inside cell-like microdroplets conferred enhanced elasticity when they were led to arrange in filaments, that is when salt was added to the microdroplets by Tanaka’s team, whereas a lesser elasticity was measured when they were uniformly distributed. In fact, as shown in Fig. 7, the microdroplets that did not contain a CAHS3 filamentous network displayed unhindered deformation under a pressure ρ≪0.5 kPa as opposed to no deformation in the case of microdroplets that did. The latter microdroplets’ Young’s modulus Eexhibited an average E = 2.0 kPa while the other ones’ E was zero (E = 0 kPa), as demonstrated by Fig. 8 (Tanaka, 2022). Stiffness was equally assessed by atomic force microscopy performed on Drosophila cells that are, just like tardigrades’ cells, devoid of canonical cytoplasmic intermediate filaments. After exposure to a hyperosmotic environment, such cells expressing CAHS3 proteins shown to be of higher elasticity than other control cells (Tanaka, 2022). Since such cells also present a greater volume in this condition, and hence their cytoskeleton-like conformation, it is reasonably proposed that CAHS proteins’ role is primarily to stiffen stressed cells and protect them from deformative forces induced by desiccation. Considering that the formation of a gel-like state was observed in vitro, after the formation of filamentous networks, with higher concentrations of CAHS proteins and accentuation of stressors associated with dehydration, it is probable that such a gel transition may happen in tardigrades shifting to the tun state, as their cells’ volume then reduces, thus also contributing to cell integrity and mechanical stabilization (Tanaka, 2022). Indeed, a volume reduction of a cell leads to increasing concentrations of proteins and ions inside of it.
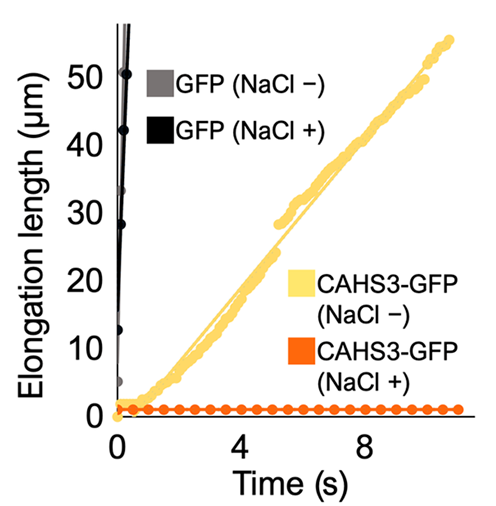
Figure 7: Elongation Length of Microdroplets under Pressure with and without the Addition of Salt with Respect to Time (Tanaka et al., 2022). ρ≪0.5 kPa. Microdroplets without salt are manifestly not elastic and liquid-like as they display very little resistance to the pressure exerted. N.-B: Microdroplets containing GFP alone show virtually no resistance to it.

Figure 8: Young’s Modulus E of Microdroplets with and without the Addition of Salt (Tanaka et al., 2022). Only the microdroplets containing CAHS3 proteins arranged in filamentous networks (thus with the addition of salt) possess a non-zero Young’s modulus.
It has been demonstrated experimentally that CAHS proteins do not only arrange themselves in fibers; in fact, they also form a gel-like matrix whose preponderance is proportional to their initial concentration before undergoing lyophilization, that is desiccation (Eicher et al., 2023). Such fibers are described as α-helices whereas β-sheets refer to the junctions between those fibers, which constitute the gel. Unlike in vitro mediums, a cell entering anhydrobiosis shrinks, and therefore the CAHS proteins’ arrangement inside of it is expected to vary accordingly to Fig. 9.
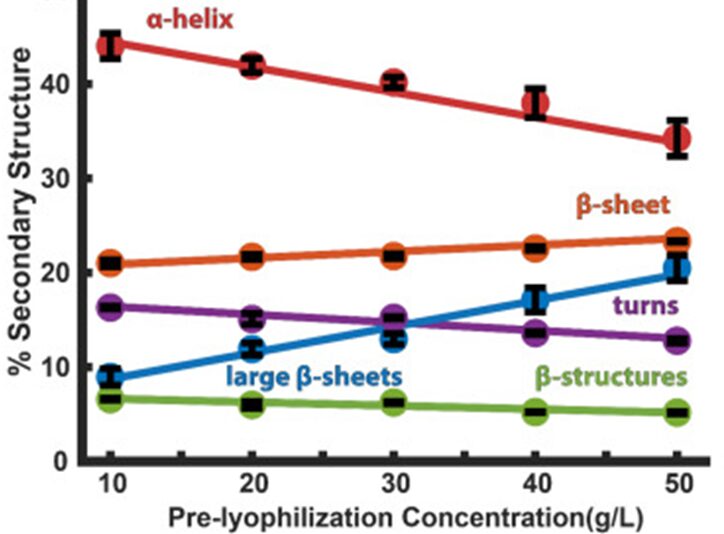
Figure 9: Percentage of CAHS α-Helices and β-Sheets in Function of CAHS Initial Concentration before Lyophilization (Eicher et al., 2023). As the graph suggests, higher concentrations of CAHS favor gel formation.
Late Embryogenesis Abundant Proteins’ (LEA) Roles
Late Embryogenesis Abundant Proteins (LEA) are found in many plant species’ seeds, roots, and pollen grain, which are subject to hydric stress, as well as in other anhydrobiotic organisms such as tardigrades. They are highly hydrophilic polypeptides that, from a physical point of view, are known, analogously to CAHS proteins, to change configuration when dried; the formation of both α-helical and β-sheets structures have been observed (Kasianchuk et al., 2023), the former being linked to fibrillation of CAHS proteins and the latter being recognized as leading to their condensation to a gel state (Eicher et al., 2023). Thus, it is very likely that they contribute to desiccated tardigrade cells’ structural integrity as well. Furthermore, due to polarities induced in their α-helix structures, LEA proteins seem to intercalate between the polar head groups within the phospholipid bilayers, making them space out from each other and thus preventing the gelation of cellular membranes (Hesgrove & Boothby, 2020), which would certainly be detrimental to tardigrade cells’ integrity, as their fluidity, sensitivity to signaling and permeability, for instance, would reasonably be impaired.
While all of these biomolecules – trehalose, tardigrade disordered proteins (TDPs) and LEA proteins have been associated with tardigrades’ resistance to the passage towards the tun state, which essentially implies that they are able to withstand deformative forces due to their shrinkage, it has besides been shown that they can also withstand intense external forces that are directly related to their environment. It is plausible to suggest that it is a consequence of their anhydrobiotic bodies with modified physical properties, as studies grant their outstanding resistance to their tun state (Ono, 2008; Seki & Toyoshima, 1998; Traspas, 2021).
Resistance to High Hydrostatic Pressure
When Macrobiotus occidentalis (order Eutardigrada) and Echiniscus japonicus (order Heterotardigrada) are not in their tun state, they cannot bear pressures over 200 MPa. However, if dehydrated before being exposed to high water pressures, respectively 95 % and 80 % of them can survive high-speed compression under a hydrostatic pressure of 600 MPa (six times the pressure of sea water at a depth of 10,000 meters), and remain under it, as well as high-speed decompression (Seki & Toyoshima, 1998). Macrobiotus occidentalis´ survival rate in relation to the hydrostatic pressures to which it is exposed is depicted in Fig. 10.
Another study puts forward an even more outstanding result: the bodies of Milnesium tardigradum were still intact after 30 minutes under a hydrostatic pressure of 20 GPa, “which corresponds to the pressure just above the lower mantle of the [E]arth,” attained at a rate of 40 GPa/h and thereafter brought down to the atmospheric pressure at a rate of 20 GPa/h (Ono, 2008). After exposition, Milnesium tardigradum (order Tardigrada) “regained metabolic state and no serious injury could be seen,” however, they were no longer alive. This indicates that their death was not attributable to bodily injuries, but rather, possibly, to lack of oxygen, DNA damage or organelle injuries. Nonetheless, the bodies of Milnesium tardigradum can bear a hydrostatic pressure of 7.5 GPa for more than 12 hours, which is still out of the ordinary in the animal kingdom.
Tardigrades’ resistance to pressure either stems from their resistance to deformative force or it is a design solution to extreme pressures in extreme environments, such as the depths of the oceans.
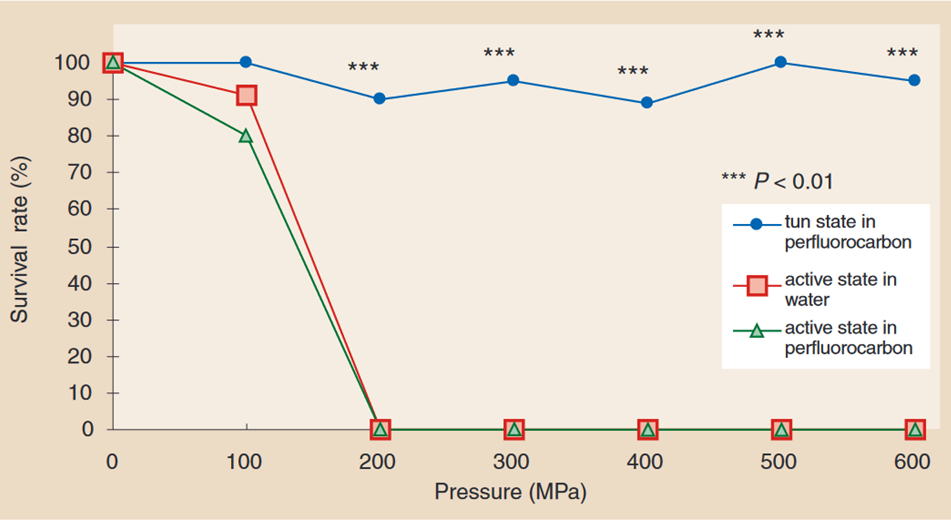
Figure 10: Survival rate of Macrobiotus occidentalis under various hydrostatic pressures, in tun state compared to in the active state (Seki & Toyoshima, 1998). While the survival rate of Macrobiotus occidentalis in its active state drops to 0 % at hydrostatic pressures of 200 MPa and above, it remains close to 100 % at hydrostatic pressures of up to 600 MPa when it is in its tun state.
Resistance to High-Speed Impacts
In addition to resisting high hydrostatic pressures, tardigrades can also resist pressures caused by high-speed impacts. Indeed, it was found that Hypsibius dujardini in its tun state could survive being fired in a gun onto sand targets at a maximal speed of 0.9 km/s, which is equivalent to 1.14 GPa shock pressure (Traspas, 2021). However, Hypsibius dujardini has taken more time to recover its active state when being subjected to a high-speed impact compared to control tardigrades in the tun state. As displayed by Fig. 11, its survival rate drastically collapsed from 100% to 0% between 0.728 km/s and 0.901 km/s.
Tardigrades’ resistance to high-speed impacts likely stems from their resistance to deformative forces as no natural constraint appears to lead to such design solution.

Figure 11: Survival rate of Hypsibius dujardini as a function of its impact speed and the peak shock pressure it undergoes (Traspas & Mark, 2021). Hypsibius dujardini easily survives impact speeds lower than 0.728 km/s; however, its survival rate rapidly drops at speeds higher than 0.728 km/s.
In summary, tardigrades’ tun state entails that they are subject to deformative forces when dehydrating, and moreover studies have demonstrated that they can withstand very intense pressures as well as high-speed impacts while also being in that state. Though trehalose was originally believed to be at the basis of the mechanisms that allow them to survive such physical constraints, it is now proposed that tardigrade disordered proteins (TDPs), principally cytoplasmic abundant heat soluble proteins (CAHS), as well as LEA proteins, also present properties that permit their outstanding physical resistance. Indeed, while CAHS proteins assemble into fibrous networks and a gel that contributes, similarly to a sturdier cytoskeleton, to their higher stiffness, LEA proteins essentially form a comparable gel and prevent the gelation of cellular membranes.
Thermoresistance
Water bears are known to be highly resilient in overcoming difficult conditions, for instance in resisting high radiation levels or extreme impacts. One of the most impressive characteristics of tardigrades, and the one that has attracted perhaps the most scientific curiosity, is their ability to resist extreme temperatures, ranging from -200 °C to 151 °C. It is only in the last few decades that scientists have been able to identify the primary mechanisms underlying tardigrades’ ability to withstand such extreme temperatures, enabling a far deeper understanding of the tardigrade’s physical interactions with their environments.
Tardigrades in Cold Conditions
Over decades of study, researchers have found that tardigrades can withstand temperatures as low as -328 °F (-200 °C). In these conditions, they no longer show any obvious signs of life despite maintaining the ability to revive, raising the question of what happens to the tardigrades’ internal clock and whether they age in this resting state.
Ralph Schill, the author of Water Bears: The Biology of Tardigrades, demonstrated that tardigrades can survive undamaged for many years by shutting down their systems. In this experiment, they froze 500 tardigrades at -30 °C, defrosted them, counted them, fed them, and froze them again, while control groups (groups that were not frozen) were kept at constant room temperature. He pursued this experiment until all the tardigrades died. In his experiment, the frozen tardigrades and the control group had almost identical life spans (quantified as the amount of time in an active state) (Mayer-Grenu A, 2022). Among those that were continually frozen, the longest-living tardigrade survived for a total of 169 days, of which 75 days were spent in cryobiosis while 94 days were spent at a normal temperature (Taub, B. (2022)). Similarly, the oldest tardigrade in the control group lived for 93 days, illustrating how the animals appear to stop aging entirely when frozen. Thus, frozen tardigrades do not change or die, but aging stops when frozen. “So even in ice, tardigrades stop their internal clocks,” concludes Schill. In fact, the tardigrades’ internal clock only resumes once their organism is reactivated, usually when they are surrounded by a film of water to allow them to take in oxygen and expel carbon dioxide.
Despite their indestructibility, not all types of tardigrades survive easily at low temperatures.
Some fare better than others. In Fig. 12, it is observed that Ramazzottius oberhaeuseri has a mean survival rate of 90 % at -80 °C, while Hypsibius dujardini has a mean of survival of approximately 20 % at -80 °C (Glime J. M, 2017). This may be due to the different concentrations of TDPs proteins that differ from one another in the different genus of tardigrades.
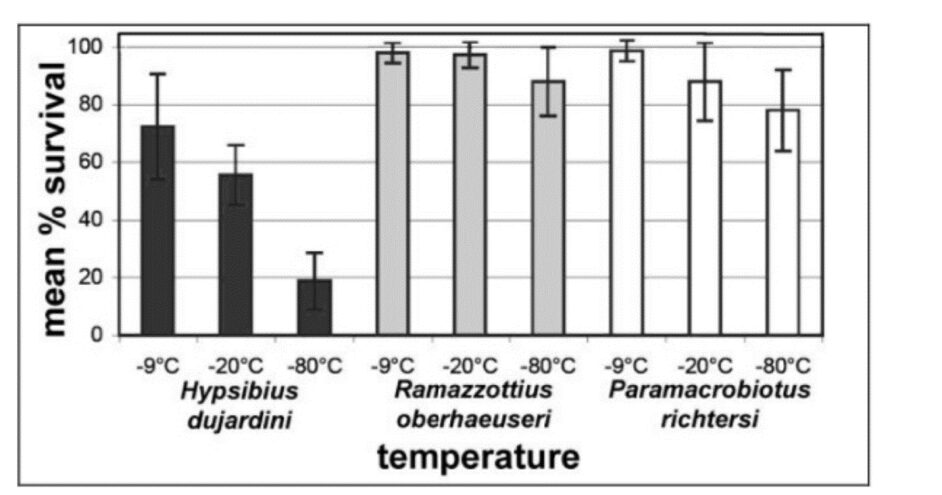
Figure 12: Mean percentage of survival of species of tardigrades as a function of temperature (Glime, 2013).
TDPs: Functional Mediators of the Tardigrade
Scientists described new mechanisms concerning how tardigrades can endure extreme temperatures that involve TDP proteins (Tardigrade-Specific Intrinsically Disordered Proteins). In addition to allowing for greater mechanical resistance, these proteins play a protective role by “guarding” their DNA from damage and by stabilizing their cellular structure, leading to the preservation of the general structure of cellular components. During cellular dehydration, TDPs form a gel that stiffens to protect the cells from this stress that would otherwise kill them, and this glasslike arrangement formed around their cells allows water bears to reanimate in water when the conditions are favorable (Mindy W.& A. B, 2022). It is also thought that such gel contributes to increasing the viscosity of the inside of tardigrades’ cells (Hesgrove C. & T. C. Boothby, 2020). Such enhanced viscosity would prevent protein denaturation, which is necessarily caused by exposure to high temperatures, and shield biomolecules from cold. It is believed that such viscous gel protects biomolecules by trapping them within the pores of an amorphous matrix, which would effectively hinder their aggregation, fusion, and fragmentation (Boothby et al, 2017). In fact, when exposed to high temperatures, the structure of folded proteins is perturbated; disulfide bridges can be disrupted, and subunits can even dissociate. Nonetheless, it has been shown that TDPs do not aggregate nor precipitate the way other well-folded proteins do, when exposed to heat (Hesgrove C. & T. C. Boothby, 2020). Thus, TDPs shield tardigrade cells from the detrimental effects of extreme temperatures.
Nonetheless, TDP proteins might themselves denature at very high temperatures (more than 300 degrees Fahrenheit, or equally 148.9 degrees Celsius) since tardigrades cannot withstand these temperatures in the tun state (Mindy Weisberger & Alina Bradford, 2022). This points to a lesser ability of tardigrades to resist high temperatures compared to low temperatures.
Tardigrades in Hot Conditions
Though tardigrades can survive extreme cold temperatures, they are not as indestructible as believed when it comes to heat. According to a 2020 research study, water bears are fragile when exposed to elevated temperatures and wilt rapidly since their proteins can unfold and their enzymes can denature (Cartier, 2020). When their proteins dry out and unfold due to excessive heat, their cellular system shuts down, leading to irreversible damage (Malki, 2022). When considering that TDPs’ gel is the main mechanism responsible for tardigrades’ thermoresistance, it can readily be hypothesized that since these proteins are also subject to greater agitation due to heat, they are also more subject to denaturation in comparison with the exposure to cold temperatures, which would not hinder TDPs protective mechanism.
In Cartier’s study, researchers exposed water bears in both states to distinct levels of heat over different intervals of time, aiming to find the lethal temperature, described as the temperature at which 50 % of the population dies. The researchers collected samples from the tardigrade species Ramazzottius varieornatus, which is found in freshwater habitats. They tested active and desiccated tardigrades that were given a period of acclimation. Following the experiment, they found that the most heat-sensitive specimens were the active tardigrades. The lethal temperature at one hour was 37.1 °C without acclimation and 37.6 °C with a short acclimation period, while the lethal temperature for desiccated tardigrades was 82.7 °C. This means that the desiccated tardigrades were far less susceptible to heat than the active tardigrades (Cartier, 2020). Thus, it is not excluded that TDPs still contribute to thermoresistance at high temperatures, though to a lesser extent at temperatures above a certain critical threshold range.
Moreover, the lethal temperature for desiccated tardigrades dropped to 63.1 °C over a 24-hour exposure time. Fig. 13 shows tardigrades, intact, after exposure to hot temperatures.

Figure 13: Light micrograph of Ramazzottius varieornatus in its active state following exposure to hot temperatures (Neves et al., 2020). Figure B depicts a tardigrade exposed to heat, but in its desiccated state.
Tardigrades are famous for their ability to survive extreme temperatures that would kill other organisms. In particular, it is the tardigrade-specific proteins – which are present during cryptobiosis and prevent protein denaturation by forming a viscous gel – that allow the tardigrades to withstand many harsh environmental conditions, including extreme temperature fluctuations and UV radiation. Although tardigrades may fight these harsh environmental conditions, their ability to survive high temperatures is surprisingly limited, and they are more vulnerable to heat than to cold. TDPs’ contribution to the intracellular viscosity provides resilience to tardigrades with respect to extreme temperatures, which they encounter either in fluctuating environments or in environments of extreme nature.
The Study of Locomotion as an Adaptation for Survival Across Various Conditions
Tardigrades rely heavily on their ability to move as their gait is the foundation to their survival; it is necessary to help them find food and mates and to elude predators. Moreover, tardigrade locomotion is ripe for research due to two remarkably uncommon features. Firstly, tardigrades are one of the few soft animals (animals with no bones) to possess limbs. Additionally, these animals locomote by walking, which is a completely unique feature as most organisms that exist at the same scale resort to other locomotive abilities. Even though tardigrades move in a unique manner given their physical properties, this does not limit their movement, as they are still able to navigate in marine and freshwater sediment, desert dunes, and soil. Because of their small size, tardigrades also generate inertial forces that would seemingly make it very difficult for them to move around in more viscous substrates; however, they still manage to propel themselves as if they were a hundred thousand times their size. This is because tardigrades have a very similar locomotive strategy to the one of larger arthropods (such as insects or crustaceans), though they exhibit major differences in size and skeleton. This strategy allows them to “scurry” at increasing speeds without changing their gaits, while still being able to modify their stepping patterns depending on the different substrates they might encounter. Studying the tardigrade’s locomotion is also crucial because it influences the tun state: the tun state is very heavily dependent on the tardigrade’s ability to navigate its surroundings. In fact, moving in extremely varying microenvironments can have a strong impact on the process of hydration and dehydration of the tardigrade, which can be fatal as these processes require to be slow and controlled (Nirody, 2021). The locomotion of tardigrades is much more complex than it seems.
The Physiology of Tardigrades and Its Relationship to Locomotion
All tardigrade species have four pairs of legs (eight in total), each ending in four to eight claws. The first three pairs display a similar structure; however, the fourth pair has been observed to possess fewer muscles. As a result of this observation, scientists have hypothesized that the three front pairs of legs have an equivalent role in forward propulsion. In fact, in experimental data, there are no major differences in kinematic parameters or stepping precision between the first three leg pairs. The last pair, on the other hand, mostly uses its claws for gripping or grasping the substrate. This physiological design suggests that tardigrades use a tension-based biomechanical strategy: tardigrades encounter difficulties in locomotion on smooth surfaces because their claw is not in action but are able to move on rougher surfaces. This was visualized in Nirody’s experiment (Fig. 14) where a polyacrylamide gel with a stiffness of 50 kPa was used and where scientists could clearly see the claw engagement in locomotion when studying the deformation of the gel (Nirody, 2021).

Figure 14: Tardigrades’ claw engagement on a polyacrylamide gel observed on video (Nirody et al., 2021). The deformation of the gel caused by the claw engagement can be seen throughout the different walking stages of the tardigrade.
Even though tardigrades are eight-legged species, their posterior legs are only used for gripping. Tardigrades can then be considered as hexapods. The three most recognized hexapod gaits are called “tripod,” “tetrapod,” and “wave gait” (Fig. 15). The tripod gait consists of separating the six legs into two groups of three and moving one group at a time, the tetrapod gait consists of separating the six legs into three groups of two that swing one after the other, whereas the wave gait consists of moving each leg independently. Examining the various stepping patterns helps researchers better understand the interleg coordination patterns (ICP) of tardigrades and other similar species (Nirody, 2021).

Figure 15: Schematic of hexapods’ stepping patterns (Nirody et al., 2021). In a tripod gait, all three limbs swing simultaneously, while in a tetrapod gait, two limbs swing simultaneously. In a wave gait, each limb swings individually. These transitions between configurations correspond to the posterior-to-anterior progression of ipsilateral swing events.
Understanding every potential gait that tardigrades might use can help analyze how these soft, microscopic animals are able to move. After conducting experiments on Hypsibius exemplaris, scientists learned that tardigrades prefer using a similar gait to the tetrapod gait across various walking speeds. Researchers considered factors like stance duration and duty factor (a crucial measure that represents the proportion of a complete gait cycle during which the leg remains in contact with the ground). They found that tardigrades exhibit a smooth, continuous relationship between these parameters and walking speed. This supports the idea that tardigrades do not have distinct gaits but rather smoothly transition between different leg coordination patterns as their speed changes (Nirody, 2021).
Factors Influencing the Tardigrade’s Locomotion Ability
Tardigrades must navigate different and difficult three-dimensional terrains (fresh water, marine, terrestrial) using different walking speeds depending on environmental conditions (walking or running from predators, etc.). This brings into consideration two very important factors in the study of locomotion: speed and substrate. Consequently, scientists use the study of kinematics to better understand how the changes in the frequency and the length of each step can affect the tardigrade’s speed, and how they manage to adapt to the different substrates they might encounter (Nirody, 2021).
To calculate the tardigrade’s different walking speeds, researchers separated the tardigrades’ movement in two phases: the “swing” phase, which is one step taken by the tardigrade, and the “stance” phase, which is the contact of the tardigrade’s leg with the ground. Researchers then employed a new measurement, the step amplitude, which can be best described as the distance between the point measured before the step was taken (posterior extreme position), the point where the leg is lifted, and the point (anterior extreme position) measured after the step as soon as there is contact with the surface. Research showed that the step amplitude increases with the walking speed of the tardigrade. After conducting research, scientists proved that the duty factor changes smoothly as walking speed varies. This further proves that tardigrades do not use different gaits or ICPs but instead make continuous adjustments as they speed up or slow down. Therefore, tardigrades do not exhibit distinct gaits, but their stepping patterns transition gradually as they change their pace (Nirody, 2021).
Tardigrades do not only adjust their gaits depending on their walking speed, but they also change their limb kinematics depending on the different substrates they encounter. The tardigrade’s environment is not homogeneous. They encounter organic substrates such as wood and peat as well as inorganic substrates such as rock and soil. As a result, tardigrades, just like any other legged animal, must adapt to the different environmental inconsistencies such as the changes in substrate’s roughness and stiffness. If the tardigrades were not able to adjust their movement, their performance and walking speed would be strongly affected (Nirody, 2021).
As mentioned above, researchers observed that hard and smooth surfaces are more problematic for the movements of tardigrades than irregular surfaces due to claw engagement. Additionally, it was shown through research on Limnoterrestrial tardigrades, that it is more difficult for these soft-bodied animals to get around on soft substrates because it does not permit adequate claw engagement (Nirody, 2021).
To properly determine the influence that the substrate’s stiffness can have on the speed of tardigrades, two gels representing two different stiffnesses were prepared: a 50 kPa gel and a 10 kPa gel. As hypothesized before, tardigrades walking on the stiffer gel (50 kPa) were almost twice as fast as the ones walking on the softer gel (10 kPa): 160.5 ± 57.8 μm/s compared to 91.0 ± 32.0 μm/s, respectively (Nirody, 2021).
To cope with the diversity of the environments, tardigrades use different methods to move around. When on a stiff substrate, tardigrades move their feet on one of the sides independently to their feet on the other side. However, their feet on a specific side are synchronized following this rule: one limb can only swing forward if the limbs in front and behind it are in contact with the ground. When tardigrades are on softer substrates, where they find it more difficult to walk, they use a different ICP: they coordinate their left and right side of limbs. This is described as “galloping” (Nirody, 2021).
In conclusion, the tardigrade’s locomotion is much more intricate than it seems: they are one of the only species of their size known to have legs and to efficiently use them. They can change their interleg coordination patterns depending on their walking speed and they are able to handle the heterogeneity of the environment that they encounter. Investigating how the locomotive abilities of the tardigrades work can help us get a better understanding of the evolution and mechanisms underlying other legged locomotion by using comparative methods (Nirody, 2021).
Conclusion
Tardigrade physiology provides numerous advantageous adaptations, allowing them to survive in extreme environments and adapt to rapidly changing conditions. Tardigrades resist extreme pressures, velocities, and temperatures, and they also demonstrate a remarkable ability to utilize their limbs to locomote despite their softness and microscopic size, as well as to reach almost complete desiccation such that successful rehydration is possible.
In fact, these remarkable characteristics represent unique design solutions (summarized in Fig. 16 below) that the tardigrade has developed in response to various environmental stresses of their extreme habitats. Firstly, tun formation allows to increase the success rate of anhydrobiosis (and cryptobiosis in turn) by allowing a controlled rate of desiccation, particularly in the lower humidity levels of many tardigrades’ high-altitude environments. Additionally, tardigrade’s CAHS proteins enable them to form a protective pseudo-cytoskeleton, increasing their mechanical resistance, and more specifically increasing their ability to survive the deformative forces that occur as a byproduct of cryptobiosis – in turn due to the oft-fluctuating environments in which they reside. Similarly, TDP proteins increase the viscosity of the cytoplasm in tardigrade cells, enabling them to survive the extreme and ever-changing temperatures of their environments. Finally, the development of varying stepping patterns and speeds has allowed tardigrades to thrive in their ability to maneuver the inconsistent substrates they encounter.
In studying the mechanisms behind these attributes, immense insight can be gleaned into techniques for obtaining resistance to many physical phenomena. This type of research could enable the human population to reap immense benefits by inspiring a diverse range of engineering innovations. For instance, the tardigrade’s approach to metabolism reduction and decelerated desiccation could inspire a method of inducing hypometabolic stasis in humans. Such an innovation would have critical applications in areas such as long-duration interplanetary flight, enabling human exploration of distant, and as yet untraversed, regions of space. Additionally, tardigrade locomotion could provide insight into small-scale technologies and micro-robotics, providing a vast array of applications from the field of health and medicine to that of commercial entertainment.
Ultimately, the tardigrade’s physical hardiness and remarkable adaptations serve as a reminder that, for most of the complex design challenges encountered by humanity in the present and throughout history, a solution has already been propagated in nature via many millennia of natural selection. As humanity looks towards the future of engineering technology, and the exciting developments to come, it is equally important to look around us, towards nature, where inspiration lies ready and in wait.
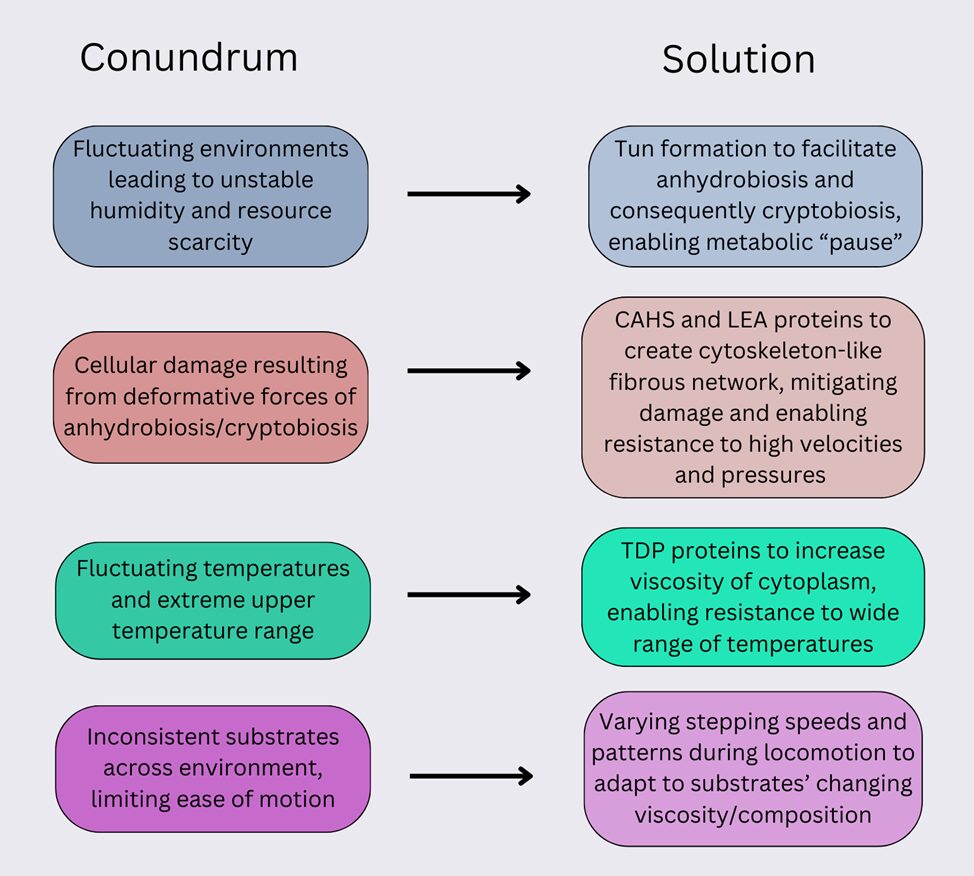
Figure 16: Diagram representing the conundrums (left) faced by the tardigrade and the solutions (right) that have emerged to mitigate them.
References
Boothby, T. C., Tapia, H., Brozena, A. H., Piszkiewicz, S., Smith, A. E., Giovannini, I., Rebecchi, L., Pielak, G. J., Koshland, D., & Goldstein, B. (2017). Tardigrades use intrinsically disordered proteins to survive desiccation. Molecular cell, 65(6), 975-984. e975. https://doi.org/10.1016/j.molcel.2017.02.018
Cartier, K. M. S. (2020, January 21). Even tardigrades will feel the heat of climate change. Eos.org https://eos.org/articles/even-tardigrades-will-feel-the-heat-of-climate-change
Clegg, J. S. (2001). Cryptobiosis–a peculiar state of biological organization. Comparative Biochemistry and Physiology Part B: Biochemistry & Molecular, 128(4), 613-624. https://doi.org/10.1016/S1096-4959(01)00300-1
Crowe, J. H. (1972). Evaporative Water Loss by Tardigrades Under Controlled Relative Humidities. The Biological Bulletin, 142(3), 407-416. https://doi/10.2307/1540318
Czernekova, M., & Vinopal, S. (2021). The tardigrade cuticle. Limnological Review, 21, 127-146. https://doi.org/10.2478/limre-2021-0012
Eicher, J., Hutcheson, B. O., & Pielak, G. J. (2023). Properties of a tardigrade desiccation-tolerance protein aerogel. Biophysical Journal. https://doi.org/10.1016/j.bpj.2023.05.002
Glime, J. M. (2013). Volume 2, Chapter 5-1: Tardigrade Survival.
Halberg, K. A., Jørgensen, A., & Møbjerg, N. (2013). Desiccation Tolerance in the Tardigrade Richtersius coronifer Relies on Muscle Mediated Structural Reorganization. PLOS ONE, 8(12), e85091. https://doi.org/10.1371/journal.pone.0085091
Hesgrove, C., & Boothby, T. C. (2020). The biology of tardigrade disordered proteins in extreme stress tolerance. Cell Communication and Signaling, 18, 1-15. https://doi.org/10.1186/s12964-020-00670-2
Jönsson, K. Ingemar, et al. Tardigrades Survive Exposure to Space in Low Earth Orbit, current biology, 9 Sept. 2008, doi.org/10.1016/j.cub.2008.06.048.
Kasianchuk, N., Rzymski, P., & Kaczmarek, Ł. (2023). The biomedical potential of tardigrade proteins: A review. Biomedicine & Pharmacotherapy, 158, 114063. https://doi.org/10.1016/j.biopha.2022.114063
Krakowiak, M., Bartylak, T., Kmita, H., Kaczmarek, Ł., & Nawrot, R. (2023a). Tardigrade proteins: molecular tools in the phenomenon of anhydrobiosis. Zoological Journal of the Linnean Society. https://doi.org/10.1093/zoolinnean/zlad066
Macdonald, H. (2004) Geologic Puzzles: Morrison Formation, Starting Point. Retrieved Sept 9, 2004, from http://serc.carleton.edu/introgeo/interactive/examples/morrisonpuzzle.html
Malki, A., Teulon, J. M., Camacho‐Zarco, A. R., Chen, S. w. W., Adamski, W., Maurin, D., Salvi, N., Pellequer, J. L., & Blackledge, M. (2022). Intrinsically disordered tardigrade proteins self‐assemble into fibrous gels in response to environmental stress. Angewandte Chemie International Edition, 61(1), e202109961. https://doi.org/10.1002/anie.202109961
Mayer-Grenu, A. (2022). How tardigrades survive freezing temperatures. Journal of Zoology. https://phys.org/news/2022-10-tardigrades-survive-temperatures.html
Miller, W. R. R. (2011). Tardigrades: These ambling, eight-legged microscopic “bears of the moss” are cute, ubiquitous, all but indestructible and a model organism for education. American Scientist, 99(5), 384-391.
Mindy W, A. B. (2022). What are tardigrades and why are they nearly indestructible? Live Science. https://www.livescience.com/57985-tardigrade-facts.html
Møbjerg, A., Kodama, M., Ramos-Madrigal, J., Neves, R. C., Jørgensen, A., Schiøtt, M., Gilbert, M. T. P., & Møbjerg, N. (2022). Extreme freeze-tolerance in cryophilic tardigrades relies on controlled ice formation but does not involve significant change in transcription. Comparative Biochemistry and Physiology Part A: Molecular & Integrative Physiology, 271, 111245. https://doi.org/10.1016/j.cbpa.2022.111245v
Neves, R. C., et al. (2020). Thermotolerance experiments on active and desiccated states of Ramazzotius varieornatus emphasize that tardigrades are sensitive to high temperatures. Nature, 10(94). https://doi.org/https://doi.org/10.1038/s41598-019-56965-z
Nguyen, K., Kc, S., Gonzalez, T., Tapia, H., & Boothby, T. C. (2022). Trehalose and tardigrade CAHS proteins work synergistically to promote desiccation tolerance. Communications Biology, 5(1), 1046. https://doi.org/10.1038/s42003-022-04015-2
Nirody, J. A. e. a. (2021). Tardigrades exhibit robust interlimb coordination across walking speeds and terrains. PNAS, 118(35), e2107289118. https://doi.org/https://doi.org/10.1073/pnas.2107289118
Ono, F. e. a. (2008). Effect of high hydrostatic pressure on to life of the tiny animal tardigrade. Journal of Physics and Chemistry of Solids, 69(9), 2297-2300. https://doi.org/https://doi.org/10.1016/j.jpcs.2008.04.019
Reyes, E., Nuñez, P., & Vázquez, R. (2023). Tardigrades, Thousand Environments to Survive. Revista Mexicana de Astronomia y Astrofisica Conference Series.
Sanchez-Martinez, S., Ramirez, J. F., Meese, E. K., Childs, C. A., & Boothby, T. C. (2023). The tardigrade protein CAHS D interacts with, but does not retain, water in hydrated and desiccated systems. Scientific Reports, 13(1), 10449. https://doi.org/10.1038/s41598-023-37485-3
Seki, K., & Toyoshima, M. (1998). Preserving tardigrades under pressure. Nature, 395(6705), 853-854. https://doi.org/10.1038/27576
Sieger, J., Brümmer, F., Ahn, H., Lee, G., Kim, S., & Schill, R. (2022). Reduced ageing in the frozen state in the tardigrade Milnesium inceptum (Eutardigrada: Apochela). Journal of Zoology, 318(4), 253-259. https://doi.org/10.1111/jzo.13018
Tanaka, A., Nakano, T., Watanabe, K., Masuda, K., Honda, G., Kamata, S., Yasui, R., Kozuka-Hata, H., Watanabe, C., & Chinen, T. (2022). Stress-dependent cell stiffening by tardigrade tolerance proteins that reversibly form a filamentous network and gel. PLOS Biology, 20(9), e3001780. https://doi.org/https://doi.org/10.1371/journal.pbio.3001780
Taub, B. (2022). “Sleeping Beauty” Hypothesis Explains How Tardigrades Survive Being Frozen. Journal of Zoology. https://www.iflscience.com/-sleeping-beauty-hypothesis-explains-how-tardigrades-survive-being-frozen-65678
Traspas, A. B., Mark J. (2021). Tardigrade Survival Limits in High-Speed Impacts – Implications for Panspermia and Collection of Samples from Plumes Emitted by Ice Worlds. Astrobiology, 21(7), 845-852. https://doi.org/https://doi.org/10.1089/ast.2020.2405
Weronika, E., Łukasz, K. Tardigrades in Space Research – Past and Future. Orig Life Evol Biosph 47, 545–553 (2017). https://doi.org/10.1007/s11084-016-9522-1
Wright, J. C. (1989). Desiccation Tolerance and Water-Retentive Mechanisms in Tardigrades. Journal of Experimental Biology, 142(1), 267-292. https://doi.org/10.1242/jeb.142.1.267
Yagi-Utsumi, M., Aoki, K., Watanabe, H., Song, C., Nishimura, S., Satoh, T., Yanaka, S., Ganser, C., Tanaka, S., & Schnapka, V. (2021). Desiccation-induced fibrous condensation of CAHS protein from an anhydrobiotic tardigrade. Scientific Reports, 11(1), 21328. https://doi.org/10.1038/s41598-021-00724-6