Abstract
Antlers are unique architectures. They are shed and rebuilt at yearly intervals, growing from bony pedicles in early spring to full-fledged structures cast in late March. The antler growth cycle—known as antlerogenesis—is a process controlled by the fluctuating interactions of myriad hormones, mainly testosterone. The growth rate is among the fastest of vertebrate bone structures, and hence the bone tissue requires a high amount of minerals for maximal antler growth. Bigger and stronger antlers are essential, as they allow males to gain high social ranking and access mates through physical combat. The chemical composition of antlers thus varies widely among cervids as they have different diets. Moreover, the regenerative nature of antlers makes them a useful model for the proteomic study of bone growth and for applications in regenerative medicine. This essay will explore how the antler growth cycle is controlled by the secretion of hormones and how nutrition and mineral intake play a significant role in it. An overview of the elemental composition of several species’ antlers will be provided. Finally, the innovative potential of antlers will be addressed by illustrating how antler stem cells provide a model for mammalian organ regeneration.
Introduction
Antlers share a similar elemental composition and microstructure with other mammalian bones. However, some distinct variations can be noticed in the functions that each bone and tissue fulfills. For instance, mammalian bones provide structural support as well as protection to their tissues and organs, whereas antlers do not fulfill either of these functions (P.-Y. Chen et al., 2008).
One of the most important characteristics of antlers is their unique regenerative ability. The National Institute of General Medical Sciences (2022) defines regeneration as “the natural process of replacing or restoring damaged or missing cells, tissues, organs, and even entire body parts to full function in plants and animals” (para. 1). Consequently, regeneration is a process that occurs in many species, including those in the Cervidae family. For instance, human hair grows back after falling from the head, and the Mexican Salamander has a backbone capable of regrowing any of its body parts (NIGMS, 2022). What distinguishes the regenerative property of antlers from that of other species is the fact that antlers grow and fall while following a perpetual cycle called the antler cycle. This cycle begins in late winter to early spring, where the antlers arise from the bony pedicles—permanent outgrowths of the frontal bones of deer (Goss, 1983; Gaspar-Lopez et al., 2008).
As an indicator of the status and social rank of deer in the community, antlers have ensured respect and diplomacy between Cervidae for millions of years. One of their main functions involves physical combat. They are also involved in sexual reproduction, as the conditions of antlers indicate how good their carrier’s genes are for survival. Indeed, an individual with the longest, largest, and most well-conditioned antlers is known for having the best genes to survive. Thus, female cervids tend to be attracted to males with the greatest antlers.
Overall, antlers along with their regenerative capability offer an interesting model for applications in environmental studies as well as in health sciences. This essay will examine the innovative potential that the seasonal regenerative cycle of antlers puts forth by first exploring the antler growth cycle, which depends on the interactions between the hormones secreted as well as the Cervidae’s nutrition intake. The antlers’ chemical composition will also be studied along with the different sources of these chemical elements. Finally, antler stem cells will be studied as a model to understand the mechanisms of stem cell regulation and as a potential prototype to the clinical application of regenerative medicine.
Antlerogenesis
The process whereby antlers are regenerated and shed during a certain interval in a Cervid’s life is called antlerogenesis. Antlerogenesis mainly depends on the secretion of hormones such as testosterone in males, and estrogen in females. In fact, the secretion of estrogen in females is responsible for the absence of antlers in female Cervids. Hormone level variation, for its part, is predominantly controlled by the individuals’ exposure to light and darkness. As a result, hormone levels and the antler cycle usually vary according to seasons, which explains why Cervidae populations often grow antlers at the same time and have antler cycles that last about a year. However, Cervidae species that live in regions with low seasonal variation have desynchronized hormone level fluctuations and, therefore, desynchronized antler cycles. In this case, antler cycles can last more than a year (Heckeberg, 2017).
Antler Cycle
In the first few years of a cervid’s life, the initiation of antler formation occurs. As explained above, the antler cycle depends on photoperiodicity, which means that it depends on the length of daylight. As days get longer, antlers are exposed to more daylight, which triggers the cervid’s pineal gland. The pineal gland then makes the pituitary gland to produce more testosterone, thereby increasing antler growth (Goss, 1983). Therefore, the cycle begins in spring, when the days start to elongate (Ledbetter, 2015). Images of a stag’s antlers through the first 6 years of his life are shown in Figure 1. Antler formation requires the formation of pedicles during the fawn’s first year. Pedicles are two platforms of bone tissue located on the frontal region of the skull. The following year, two jagged antlers grow on the pedicles and end up casting at the end of the cycle. In the third year, antlers with multiple branches are formed and then fall at the end of the year. The same cycle continues in the following years, where antler branches multiply every year during the animal’s youth (Hall, 2015).

Fig. 1 Development of a stag’s antlers throughout the first 6 years of its life (Hall, 2015).
When the days begin to shorten, antlers mineralize and harden. The velvet that covers the antlers dries and the stag starts to rub its antlers on trees to get rid of the dried velvet. After the breeding season, the base of the pedicle gets gradually destroyed by osteoclasts, which are cells that degrade undesired bone (Ledbetter, 2015).
The Role of Testosterone in Antlerogenesis
The most important hormone in the antlerogenesis of male cervids is testosterone. Evidence has shown that castrated fawns do not form pedicles. However, when provided with testosterone, castrated fawns end up forming pedicles. Further experiments were performed on female deer who were administered testosterone from external sources. The experiment resulted in the growth of pedicles on a female deer’s head (Hall, 2015).
Testosterone is also involved in the shedding of antler velvet and the detachment of antlers from their carrier’s skull. Toward the end of the antler cycle, the pineal gland perceives the reduction of daylight and sends a signal to the testes, which increases testosterone secretion. High levels of testosterone engender mineralization of antlers, thereby causing the solidification of the bones.
Antler shedding is thus entirely dependent on testosterone and seasonal variation. The antler cycle is also closely correlated to the fertility-sterility cycle of cervids. In spring, spermatogenesis and the growth of antlers both begin, but cervids remain sterile. Signs of fertility only appear at the beginning of summer when the testes start to increase in size. The Leydig cells, which are responsible for the production of testosterone, also start to increase in size. At this point, the antlers are in their velvet state. During summer, as testosterone levels increase, mineralization and shedding of the velvet occur, as explained above. In October or November, during the rutting season, the mass of the testes has quadrupled, and levels of testosterone reach their peak. The differences in testosterone levels in male cervids are illustrated in Figure 2. Finally, during winter, the amount of testosterone flowing in the bloodstream decreases, and antler casting occurs (Goss, 1983).
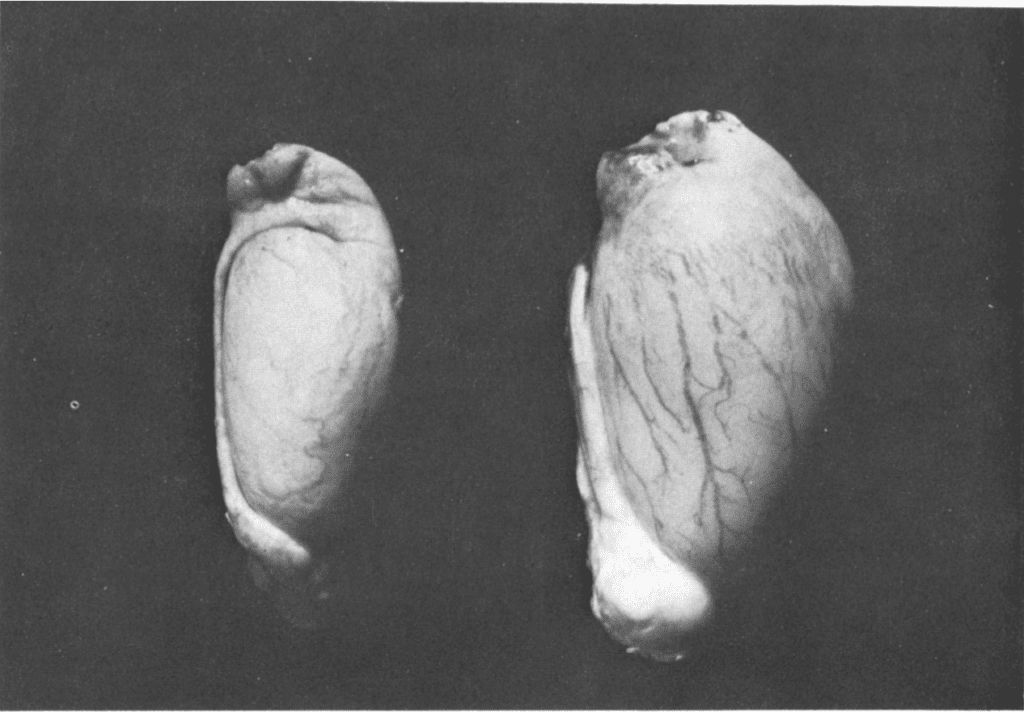
Fig. 2 Size of the testes of a Sika Deer in spring (left) and in fall (right) (Goss, 1983).
Interestingly, if an adult cervid is castrated in winter or in fall, its antlers will never fall, thereby becoming permanent bone structures devoid of velvet. This is because during these two seasons antlers do not grow and are made of bone tissue. On the other hand, if a cervid is castrated during summer, its antlers will also not shed and velvet will remain permanently. The presence of velvet is explained by the antler cycle, which is in the antler growth state during summer (Hall, 2015).
The relationship between testosterone levels and antlerogenesis is summarized in Figure 3. This figure compares the fluctuations in testosterone levels throughout the year to the different phases of the antler cycle. The black regions represent when the antlers are in their bone state, while the cross-hatching represents when the antlers are covered in velvet. The dotted region illustrates the stage of mineralization.

Fig. 3 Levels of testosterone throughout the year and phases of the antler cycle (Goss, 1983).
The Effect of Estrogen on Antler Growth
The absence of antlers in female cervids is explained by the secretion of estrogen. Experiments where male deer were administered exogenous estrogen showed that this hormone inhibits the formation of pedicles (Hall, 2015). However, estrogen does not prevent the formation of antlers once the pedicles have developed. Indeed, in the context of an experiment testing the effects of estrogen on antler growth, estrogen was injected in January into a castrated male red deer. This resulted in the absence of antler growth. However, when estrogen was given in August, the shedding of the velvet occurred prematurely. Overall, various experiments have shown that estrogen prevents antler loss and induces velvet shedding if it is administered during antler growth. Consequently, estrogen and testosterone both have similar effects on an adult cervid. Nevertheless, their effect on fawns is opposite.
The Role of Nutrition on Antler Size
Bucks shed and regrow their antlers every year. After the rutting period, they shed their antlers between January and March (Price and Allen, 2004). Then, in April, they begin producing a new pair that will be ready for the rut in the fall. Antler size is important for the rut as it allows males to compete for females and defend their calves throughout the winter.
A study led by French et al. revealed a close relationship between nutrition and antler size in white-tailed deer by analyzing how various nutritional concentrations affect antler growth. In the experiment, fawns were assigned eleven separate experimental rations created to give both high and low amounts of important nutrients such as protein (sulfur-containing amino acid), calcium, and phosphorus. Table 1 compares the average length of main beam, diameter, and total weight of antlers in deer with complete nutrients to deer with deficient nutrition. Deer fed a complete ration produced fully developed, large, branched antlers with higher points, about 13–15 inches (0.3302-0.381 meters) main beams as well as one-inch (0.0254 meters) diameters about an inch (0.0254 meters) above the antler base. However, deer with a nutrient-deficient ration produced only half the length and diameter of deer fed a complete ration for one year in unlimited amounts (French et al. 1956).

Table 1 Measure of average length of an intact antler’s main beam, diameter, total weight of antlers, density of antlers, and date antler shed in the first year (French et al., 1956).
French et al. also investigated the level of recovery achievable during the second year by shifting one deer from the restricted diet to the control ration. As shown in Table 2, deer maintained low energy, calcium, and phosphorus levels. Low protein rations resulted in stunted growth, low total points ranging in 2-4, and thin spike antlers ranging in length from 7 to 12 inches (0.1778 to 0.3048 meters). Also, they developed main beam diameters of 0.5-0.8 inches (0.0127-0.02032 meters) and average length of main beam of 7-13 inches (0.1778-0.3302 meters). Whereas the control and newly implemented deer developed larger antlers varying in number of points from 6 to 14, with main beam lengths of about 15-18 inches (0.381- 0.4572 meters), main beam diameters of 1.0-1.3 inches (0.0254- 0.03302 meters), and average length of main beam of 13 to 18 inches (0.3302 to 0.4572 meters) (French et al. 1956). Bucks with lower main beam diameters and average main beam length spreads have smaller and thinner antlers than those with higher values.
The adjustment made midway through the second trial year resulted in a quick recovery in antler development, but these animals were not able to achieve the size of the control group. Also, deer on deficient diets began antler maturation later when compared to well-fed bucks of comparable age. This experiment indicated that antler size is heavily dependent on minerals such as calcium and phosphorus, and it is advantageous to have a complete nutrient feed to offer optimum ability of antlers, particularly during the rut.

Table 2 Measure of the average length of an intact antler’s main beam, diameter, total weight of antlers, density of antlers, and date antler shed in the second year after several modification (French et al., 1956).
Effect of Supplementation of Calcium (Ca) and Phosphorus (P) on Antler Mineral Composition
Antler growth rate is one of the fastest rates among vertebrate bone structures (Goss, 1983). For example, the average growth rate of cattle horn is about 1.2 centimeters per month whereas antler growth rate may exceed 1 cm per day, and antlers grow to 1.2 meters or more (Hillman et al., 1973). Because this rapidly growing bone tissue demands a high amount of minerals, partial demineralization of the deer skeleton may occur in antler development, resulting in periodic osteoporosis (i.e., a condition that weakens bones to the point where they break easily) (Goss 1983).
University of Georgia research discovered 11 distinct minerals in deer antlers and revealed that calcium and phosphorus are the two most common elements in antlers. (Miller 1985). However, the partial demineralization of these bodily calcium and phosphorus sources only provides a portion of what is required for maximum antler growth, the remaining must be received directly from their diet when their antlers are actively growing (Tajchman et al., 2020).
Therefore, Tajchman et al. investigated how increasing dietary Ca and P intake for fallow deer increases macroelement concentrations in their bones, which act as a reservoir for subsequent antler development and protect against periodic osteoporosis, as well as antler mineral composition. As indicated in Table 3, the experimental group (I) supplied with larger dosages of Ca and P than the control group had a 14.45 average antler size compared to 10.38 for the control group (C) (Tajchman et al., 2020).
Furthermore, the experimental group had greater macroelement levels in their antlers and bones. Although the dietary supplement used in the experiment did not contain additional K, the experimental group (I) had increased K along with an increase in Ca and P when compared to the control group (C) (Tajchman et al., 2020). As a result, the scientists concluded that higher calcium and phosphorus intake raises K levels in antlers, which is an essential microelement that stimulates antler growth by mobilizing Ca from the skeleton and minimizing Ca loss via urination (Rafferty et al. 2005).

Table 3 Measure of average animal performance depending on the composition of the dietary supplement in control (C) and experimental (I) groups (left), the average content of selected macroelements in fallow deer antler and bones (right) (Tajchman et al., 2020).
Cervids, as previously stated, are reported to be the only mammals capable of utilizing minerals such as calcium and phosphorus from their bones for antler deposition and then supplementing bone calcium from the diet when the antlers harden (Banks, 1968). When being put through different experiments to evaluate the effect of Ca and P supplements on the antler development, the group provided with extra calcium and phosphorus showed an increase in antler and bone mineral compositions, which protect against periodic osteoporosis, having enough minerals to be demineralized (Tajchman et al., 2020). Therefore, French et al. demonstrated that nutrition is required for maximal antler growth and overall health, establishing a strong association between nutrients and antler growth size.
Elemental Composition of Antlers of Different Species Through X-Ray Fluorescence
According to several studies, antler bone is considered to be one of the toughest bones in the cervid’s skeleton (Zioupos, 1994). The presence of an outer cylinder combined with the spongy core of the bone gives it its maximal stiffness (Goss, 1983). During the rut, it is even stronger due to the constant flow of blood (Chapman, 1981). These interesting properties of antlers caused their worldwide export, especially to India, where antler trade was allowed. Antlers are mostly used as trophies, decoration, or to make efficient tools such as cutlery handles, pistol butts, hangers, buttons and even furniture (Menon, 1994). However, the mis-declaration of massive quantities of antlers made the trade illegal as in place of the 100 metric tonnes allowed for exportation, 150 and 200 tonnes were leaving the country (Aziz, 1996).
To strengthen the legal measures of antler trafficking and to protect cervids, the antler identification in any form becomes crucial (Caro, 2003). Therefore, a study was carried out to classify cervid species from their antlers using an elemental analysis so they could be preserved. The antler samples were collected from the laboratory and were analyzed by X-Ray Fluorescence. The antlers of five species were analyzed by passing under the fluorescence spectrometer, namely Axis axis (chital), Rusa unicolor (sambar), Rucervus duvaucelii (swamp deer), Muntiacus muntjak (barking deer) and Axis porcinus (hog deer). X-Ray analysis of the antlers of five deer species revealed high intensities for calcium, phosphorus, strontium, iron, silicon, sodium as well as magnesium. On the other hand, low intensities for sulphur, zinc, chlorine, copper, aluminum, and potassium were observed (Singh, 2022). Figure 4 presents the results of the different fluorescence X-Ray analysis by comparing the intensities of the different elements of the periodic table according to the studied species.


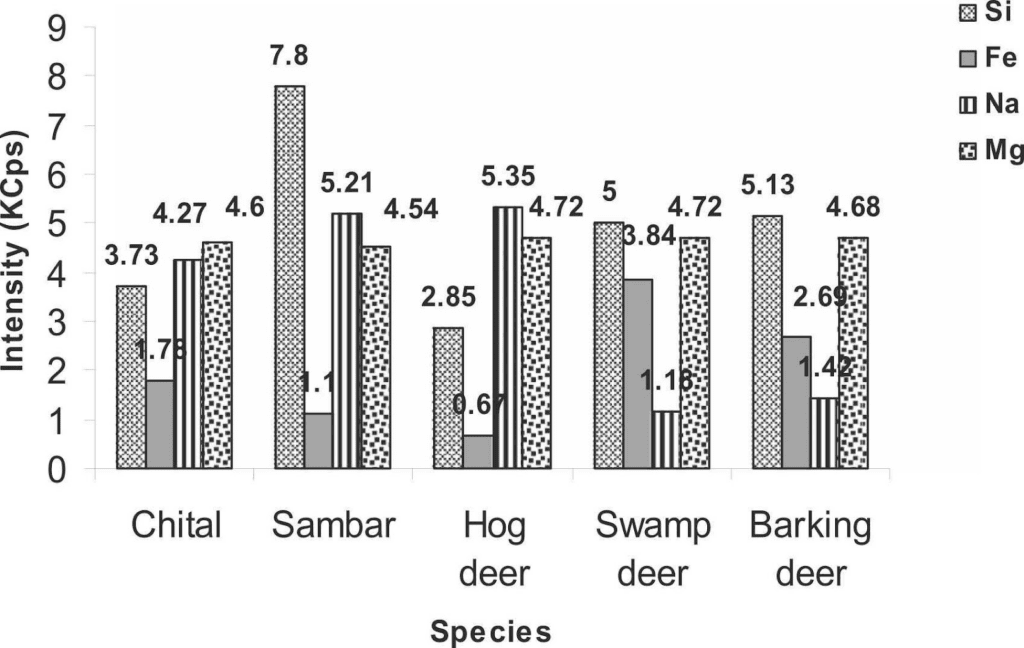
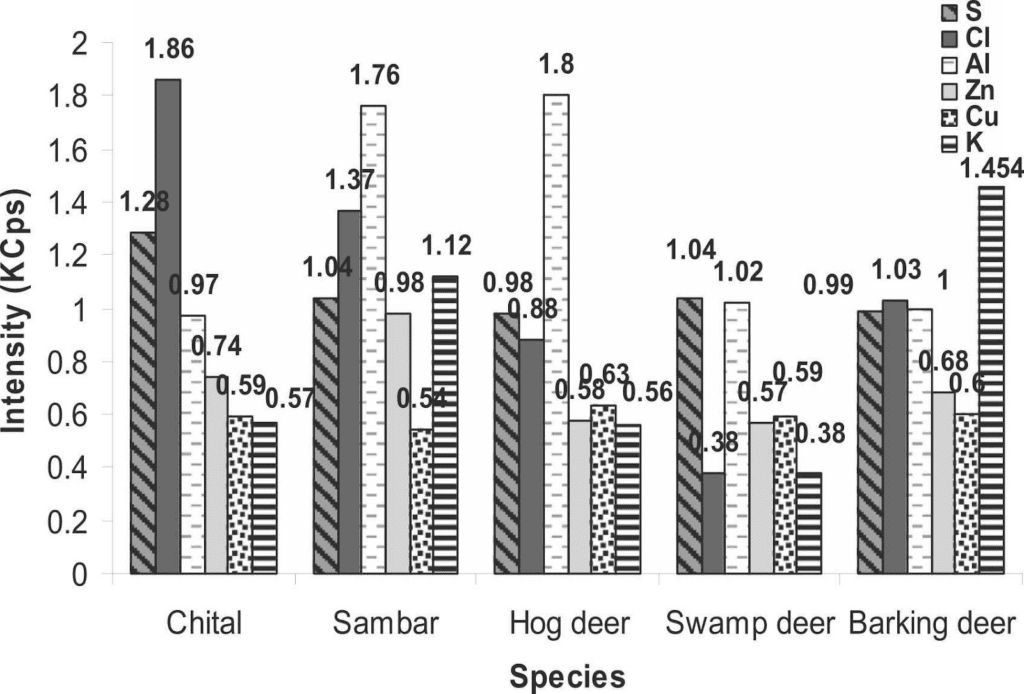
Fig. 4 The intensity of different chemical elements (calcium, phosphorus, strontium, silicon, iron, sodium, magnesium, sulfur, chlorine, aluminum, zinc, copper and potassium) measured in kilo counts per second (KCps) observed in the antlers of five deer species, namely the chital, sambar, hog deer, swamp deer and barking deer (Singh, 2022).
According to Figure 4, calcium was very intense in all species while phosphorus was only half as intense as calcium. The intensity of calcium (286.16 KCps) and phosphorus (137.06 KCps) was highest in swamp deer. Strontium was more strongly observed in hog deer (38.42 KCps) followed by sambar (36.45 KCps) and chital (35.24 KCps) but was not identified in the remaining species. Silicon intensity was highest in sambar (7.8 KCps), iron in swamp deer (3.84 KCps) and sodium was highest in hog deer (5.35 KCps). Magnesium intensities were mostly equal in all studied species. For elements of minor intensity, there were very few differences between them. For sulphur and chlorine, it was in the chital antlers (1.28 KCps and 1.86 KCps respectively) that it was the highest, while in sambar and hog deer aluminum was the most intense (1.76 KCps and 1.8 KCps) in contrast to the other three species. Zinc was the strongest in sambar (0.98 KCps), as was potassium, which was twice the proportion in chital and hog deer. Copper showed a uniform intensity among the five species (Singh, 2022).
Toxic Elements Found in Antlers of Certain Species Considered as Elevated and Chronic Wasting Disease
In antlers, calcium and phosphorus are predominantly found as hydroxyapatite (Ca5(PO4)3OH), accounting for approximately 21% and 10% of antler dry weight, respectively (Chapman 1975). A study conducted by Bubenik (1968) analyzing the composition of velvet antlers revealed that they are composed of about 54% organic matter, 34% ash and 12% moisture (Bubenik, 1968). An even more detailed analysis illustrated the presence of 47% protein, 33% mineral, about 3-4% lipid, and 3% sugar (Bubenik, 1968). The major protein which composes the antlers is collagen, along with amino acids such as glutamic acid, alanine, glycine. Lipids are predominantly present as gangliosides, sphingomyelins, steroids with a thin fraction of prostaglandins and polysaccharides (Bubenik 1968). Moreover, the results obtained in the study support the statement of Moen et al., who predicted that Megaloceros giganteus antlers with a mass of 40 kg could have contained 2.1 kg of nitrogen, 7.6 kg of calcium and 3.8 kg of phosphorus (Moen, 1999). Despite the small number of samples collected, the high intensities of some elements in some species, the lower intensities in others, or even the absence and presence of certain elements can be highly effective in differentiating antler physiology interspecifically. For instance, high levels of silver, barium, and strontium as well as low levels of copper have been measured in antlers, soils, and pastures in areas affected by chronic wasting disease (Purdey, 2004). Chronic wasting disease (CWD) is a transmissible spongiform encephalopathy that affects deer associated with prion proteins, a cellular glycoprotein (PrPC). This disease first affects lymphoid tissue and then spreads to the central nervous system and adjacent tissues (Williams, 2005).
Purdey associated these variations in elemental concentrations measured in areas affected by CWD to artificial and geochemical sources of pollutants. To illustrate the proportions of these materials in the antlers, Purdey gave average values for barium and strontium. Compared to the reference values for these elements in the bone matrix of 5 ppm (Ba) and 52 ppm (Sr), the values from Purdey of 181 ppm (Ba) and 119 ppm (Sr) can be considered as high (Purdey, 2004). In this study, three species came from the same region, while the other two species came from distinct regions, which may have resulted in slight fluctuations in the intensities between these species. In this context, barium and strontium concentrations were determined to find out whether the geographical origin of the samples could have any relationship to the chemical composition of antlers. For chital, an average barium concentration of 33.03 ppm was measured, while a value of 128.57 ppm was obtained for sambar, which is considerably high. Swamp deer reported the highest barium concentration at 207.55 ppm. Hog deer and barking deer reported values of 55.42 ppm and 143.86 ppm, respectively. Strontium was also found to be considerably high in all species: chital (90.18 ppm), sambar (64.79 ppm), swamp deer (71.79 ppm), hog deer (66.34 ppm) and barking deer (289.71 ppm).
The Innovative Potential of Antlers: Applications in Environmental Studies and Regenerative Medicine
Application of the Chemical Composition of Antlers in Environmental Studies
Considering these results, it is then necessary to examine how the origin of the samples and the environment in which they were collected may affect the chemical composition of antlers. In this sense, antlers from several species have been used in environmental studies to establish ambient levels of certain contaminants (Kierdorf, 2005). Their seasonal regeneration makes them an excellent observation and analysis unit for researchers (Kierdorf, 2005). An antler analysis from a certain region over many years can then be useful to reconstruct the temporal evolution of changes in the concentration of certain environmental contaminants such as fluorine or lead (Kierdorf, 2000). A promising field for future research topics is the analysis of isotope signatures in antler bones to determine stable isotope ratios of lead 204, 206, 207 and 208 present in the environment to have a greater understanding of the general distribution of lead sources (Landete-Castillejos, 2019). Moreover, a study was conducted in Austria to analyse the amount of lead present in the environment using the bone material of red deer antlers. As hunters retain the skulls of the deer they hunt down with the precise date and place where they hunted them down, the antlers represent valuable non-altered samples for environmental research. The purpose of this study was to compare lead pollution before and after the addition of lead additives in car gasoline. To do so, 300 samples of reed deer antlers hunted down between 1909 and 1990 from 14 different regions of Austria were collected. A fraction of the antler was then isolated, dried, ashed and dissolved in 5 ml of HNO3 and the concentrated solution was analyzed using the Perkinelmer 5000 (Tataruch 1995). During the annual period of antler growth, the minerals necessary for their formation are mobilized from the skeleton and the diet of the deer. Traces of elements present in plants are stored in antlers, which explains the presence of contaminants such as lead (Tataruch 1995). The presence of lead in an animal’s system can be caused by two main sources: absorption by the respiratory tract and absorption by the digestive tract. Lead is deposited largely on the surface of some plants while a small portion is absorbed by the plant from the soil. When feeding, the deer ingest some soil in addition to the plants, which adds lead to the total amount already present in the deer tissues (Tataruch, 1995). Also, lead in the animal is an indicator of air contamination as it comes from atmospheric deposition. The major results of this research were that they were able to reconstruct a timeline of the variations of lead concentrations associated with evolution of gasoline in Austria. For example, lead levels found in samples before World War II had a high lead concentration, which is strange if one considers the smaller density of traffic. The reason behind those high levels is probably caused by the higher use of coals for power units in manufacturing and heating conditions, which contains metals that are released in the environment as smoke and particles because of a lack of filters (Tataruch, 1995).
What Makes Antlers Special: An Evolutionary Perspective
While generalist species are less specialized species which can utilize a variety of resources from their environment (i.e., having a diverse diet or diverse habitats), specialist species can only thrive within a narrow range of conditions and resources, and are thus highly specialized (Li et al., 2014). Therefore, while a worm may be able to lose its head and grow it back, it is a relatively simple organism, as its body is divided into a series of repetitive segments that are fundamentally similar in structure. On the other hand, Cervidae are complex organisms which evolved about 30-40 million years ago from the Miocene Muntiacinae in central Asia (Gross, 1983). The framework of speciation and regrowth of antlers is highly specialized and limited to Cervidae, as antlers evolved over millions of years, starting from small and simple architectures, and transforming into complex hierarchical structures with elaborate branching (Gross, 1983). Antler regeneration is thus limited to the family of Cervidae and to a certain anatomical niche, as the regeneration does not happen at the level of the entire skeleton.
Stem Cells
During the early embryonic development of all animals, pluripotent stem cells compose the inner cell mass of the embryo and can divide or differentiate to become any cell type in the body (Kolios & Moodley, 2013). This versatility allows embryonic stem cells to self-repair and regenerate. The appeal in regenerative medicine is then to recapitulate the cellular processes responsible for the growth and development of animals at an early stage of cell division. Ideally, stem cells could thus be applied to cell therapy to replace damaged cells or regenerate diseased tissues and organs (Kolios & Moodley, 2013).
The practical question is: if, for instance, salamanders can grow back their tail, why is it that humans cannot grow back limbs? Amphibians evolved from fish near the end of the Cretaceous era, while reptiles evolved about 250 million years ago, and mammals only first appeared about 210 million years ago. Therefore, as pictured in Figure 5, since salamanders are amphibians, they diverged from the evolutionary tree a long time ago and specialized and evolved towards these regenerative abilities, while mammals, including humans, did not. Similarly, Cervidae evolved towards seasonally regrowing deciduous antlers as an adaptive strategy to establish social hierarchy millions of years ago (Gross, 1983).
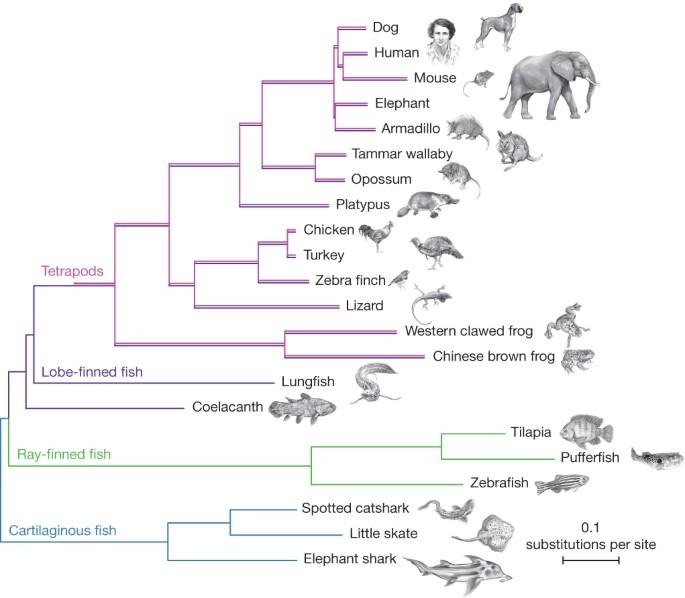
Fig. 5 A phylogenetic tree of vertebrates illustrating how amphibians (e.g., frogs) branched out and evolved a long time ago and have common ancestors to mammals. Humans and rats, being generalist species, are more closely related to each other than they are to amphibians such as salamanders, for instance (Amemiya et al., 2013).
Antler Stem Cells (ASCs) and Regenerative Medicine
Stem-cell-based regeneration has significant potential in biotechnology and the clinical application of regenerative medicine (Ilic & Polak, 2011). However, prior to medical application, scientists must deepen their understanding of the activation and control of stem cells. As deer antlers are the only known mammalian organ to undergo physiological stem-cell-based regeneration of bone, nerves, blood vessels, cartilage, and skin, they provide an ideal regenerative model to grasp the underlying mechanisms of stem cell regulation (Zao et al., 2016; Dong et al., 2020). The main inspiration is that if organ regeneration is possible in one organismal niche, perhaps there is a way to use it in another skeleton element or organism.
The Proteomic Profiling of Antler Stem Cells: A Model for Regenerative Medicine
Proteomics, or the large-scale study of proteins, is one of the most promising models to investigate the biological processes involved in antler bone regeneration (Franco et al., 2013). The goal is to identify protein networks and molecular mechanisms involved in the activation and maintenance of the stem cell niche of antlers to better understand the regenerative nature of antlers (Dong et al., 2020).
Histological and morphological studies of antlers have shown that the distal pedicle periosteum, a thick membrane located on the surface of the bone, contains the main cell source responsible for the initiation of antler renewal (Wang et al., 2018). The pedicle periosteum cells (PPCs) possess stem cell characteristics, such as the mesenchymal stem cell (MSC) markers CD90, CD105, Stro-1, Oct4, Nanog, and SOX2. MSCs are multipotent cells which can differentiate into a variety of cells, for instance those that compose adipose, cartilage or bone tissue (Dong et al., 2020; Feleke et al., 2020).
Proteomic Study of Pedicle Periosteum Cell Membranes
In a study conducted by Wang et al. (2018), the plasma membrane proteins of pedicle periosteum cells and facial periosteal cells—two cell types found in antlers—were isolated and enriched using gradient centrifugation. Their goal was to identify the proteins associated with the membrane of PPCs, as membrane proteins encompass one-third of the total number of proteins encoded by the human genome. Moreover, due to their interfacial position in cells, membrane proteins are involved in cellular processes such as signal transduction, cell adhesion, and transport of molecules. For instance, PI3K/AKT and VEGF are signaling pathways which play central roles in signal transmission (Wang et al., 2018).
Interestingly, the scientists found that a myriad of regulatory proteins such as TGFBR, VEGF, and ITGA/B were upregulated in the PPCs, illustrating that PI3K/AKT and VEGF signaling pathways participate in angiogenesis and cell proliferation of antler regeneration. Thus, this proteomic study of pedicle periosteum cell membranes helped better understand the mechanisms involved in antler regeneration (Wang et al., 2018).
Identification of Unique Proteomic Markers of Antler Stem Cells
In another study conducted by Dong et al. (2020), Ingenuity Pathway Analysis (IPA) and label-free quantification were used to investigate the protein pathways and networks of dormant, post-active, and facial periosteum cell niches involved in antler regeneration. Their goal was to identify key regulatory proteins and discover the signaling pathways and mechanisms involved in antler stem cell activation to add to the proteomics research field of antlers as a model for mammalian organ regeneration (Dong et al., 2020).
Three tissue samples—three before, and three after antler regeneration—were collected. As shown in Figure 6, the samples included parts of the mid-beam antler periosteum, antler growth center, dormant pedicle periosteum, and facial periosteum (Dong et al., 2020).
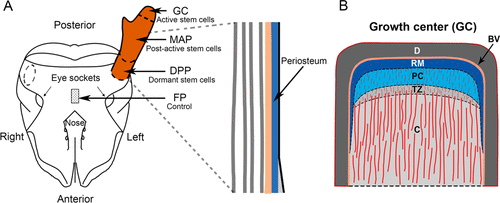
Fig. 6 Samples taken for the experiment: A) Schematic diagram of deer antler, including the facial periosteum (FP), dormant pedicle periosteum (DPP), mid-beam antler periosteum (MAP), and growth center (GC); B) Reverse mesenchymal layer (RM), which encompasses the stem cells from which antler growth is driven: other layers include dermis (D), blood vessel layer (BV), precartilage (PC), transition zone (TZ), and cartilage (Dong et al., 2020).
Three MSC markers (CD73, CD90, and CD105) were detected by immunohistochemistry within the reserve mesenchyme (RM), precartilage (PC), and cartilage (C) layers. The detection of the markers was stronger in intensity in the RM and PC layers compared to the cartilage layer, suggesting that the stem cell niche constitutes both perivascular (i.e., cells involved in tissue repair) and interstitial cells (i.e., cells involved in signaling) within these regions (Dong et al., 2020).
Potential unique markers of dormant and post-active stem cells were also identified. For instance, sushi repeat containing protein X-linked (SRPX) (a tumor suppressor protein), collagen type XXV alpha 1 chain, which has an anti-amyloidogenic function, and haptoglobin—a protein involved in immune regulation—were found in dormant stem cells of the DPP. Proteins unique to the post-active stem cells include the S100 calcium binding protein A7 and plasmin-2, associated with hematopoietic stem cells (i.e., multipotent stem cells that can develop into all types of blood cells) (Dong et al., 2020).
In conclusion, unique markers for stem cell niches at dormancy and post-activation were identified. The upregulation of several molecular functions and pathways involved in antler regeneration provides insight into the control mechanisms involved in mammalian organ regeneration.
Regenerative Wound Healing in Rats Using Antler Stem Cell-Conditioned Medium
Previous studies have shown that antler stem cells—besides from being able to fully regenerate deer antlers—can rapidly promote cutaneous wound healing (i.e. the repair of damaged tissue to restore physiological and anatomical function) at the initial stage of antler regeneration (Singer & Clark, 1999; Rong et al., 2019; Lee et al., 2016). In fact, antler stem cells (ASCs) can stimulate healing on the deer’s pedicles as well as on skin anywhere on the deer’s body, illustrating that the healing is not specific to skin type (Lee et al., 2016).
While rats also have repair mechanisms at a particular stage of development, regeneration in rats is limited once these cells are differentiated and specialized. Rat healing cannot reach the same extent as the regeneration, for example, of a full tail in salamanders, or of antlers in deer. These species branched out from the phylogenetic tree and evolved towards specific regenerative mechanisms millions of years ago.
An experiment conducted by Rong et al. (2019) investigated whether the antler stem cell healing promotion was species-specific by injecting antler stem cells into wounded rats. Since wound healing consists of two phases—angiogenesis which mainly involves endothelial cells, and granulation tissue formation, which involves fibroblasts—the scientists selected both HUVECs (endothelial cell type) and NIH-3T3 (fibroblast cell type) to test whether the antler stem cell-conditioned medium (ASC-CM) could promote proliferation of these two cell types (Rong et al., 2019).
The researchers selected three sticks of antlers from three sika deer of age 2 and cut the mesenchymal layer for primary cell culture. After isolating the ASCs, they were cultured in DMEM and supplemented with various drugs and chemicals. Human umbilical cord mesenchymal stem cells were also isolated, cultured, and supplemented. The two different types of stem cells were used to create the conditioned media (Rong et al., 2019).
The scientists created full-skin thickness wounds in rats and treated the experimental group with 200 μl of the ASC-CM hydrogel every 2 days, while three controls were treated with other media (Mesenchymal stem cell-conditioned medium hydrogel (MSC-CM), DMEM and epidermal growth factor (EGF)) (see Figure 7). Skin damage was recorded every 4 days. As observed in Figure 7, the results illustrate that fastest healing rate occurred in the ASC-CM group, as the rats’ wound healing was completed on day 16 (± 3.5 days), compared to all three controls, which took much longer to heal (DMEM group, 25 ± 1.6 days; EGF group, 23 ± 2.6 days; MSC-CM group, 20 ± 1.8 days).
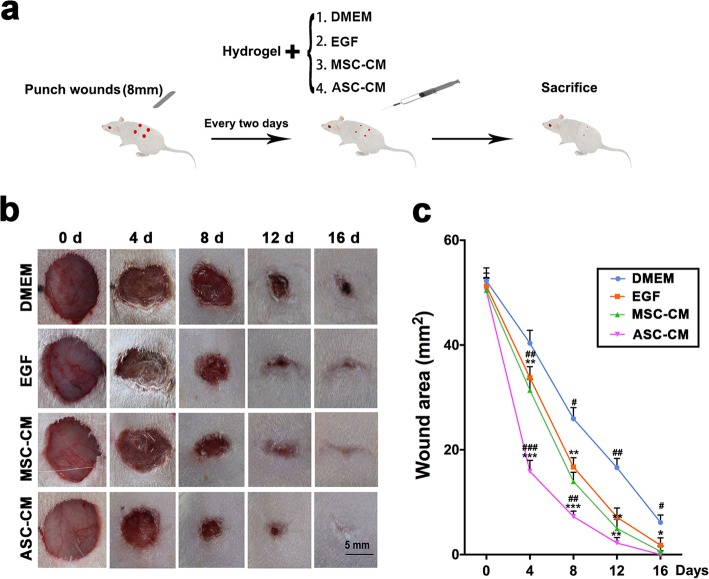
Fig. 7 The effects of ASC-CM on cutaneous wound healing in rats: a. Summary of the experimental procedure; b. The different morphological changes observed during the wound healing procedure; c. Changes in wound area during healing time. It can be observed that the ASC-CM group had the fastest wound healing rate as well as the smallest wound area (Rong et al., 2019).
The ASC-CM treatment upregulated the expression ratios of Col3A1/Col1A2 and TGF-β3/TGF-β1, genes which are known to recapitulate those involved in the regenerative healing of fetal skin wounds. Moreover, the ASC-CM successfully stimulated the proliferation of both the endothelial and fibroblast cell types, suggesting that ASC-CM may prompt cutaneous wound healing in rats (Rong et al., 2019). Therefore, antler stem cells accelerated wound closure rate in rats and could potentially be developed as a therapeutic for wound healing in medicine. The challenge remains that limb or organ regeneration is highly specific to certain species and is difficult to apply to other organisms.
Moreover, clinical applications of mesenchymal stem cells raise numerous ethical and safety concerns. For instance, MSCs are responsible for promoting tumor growth and metastasis, and public debates have argued that manipulating stem cells violates the order of nature (Volarevic et al., 2018). Thus, regenerative medicine sparks an ethical dilemma which is addressed by different legislations regulating research in stem cells.
Conclusion
Deer antlers are distinct mammalian appendages, meaning that they are cast and fully regenerate from permanent bone protuberances every year. This process of regenerating and shedding within a certain interval is referred to as antlerogenesis and is mainly dependent on seasonal variations and hormone secretion. Both testosterone and estrogen have similar effects on an adult cervid, such as promoting velvet shedding, but they have the opposite impact on fawns. A well-balanced diet is essential for encouraging deer antler growth throughout the critical antler growing season. As calcium and phosphorus are mostly contained as hydroxyapatite in antlers, enough calcium and phosphorus supplementation is required for attainment of maximum growth and adequate protection against periodic osteoporosis. Furthermore, antlers can be utilized in a variety of fields, including environmental research to study the temporal evolution of changes in the concentration of environmental toxins like fluorine or lead, and medicine to treat unsolved syndromes and diseases. The upregulation of various molecular processes and pathways involved in antler regeneration provides insight into the regulatory mechanisms involved in mammalian organ regeneration via distinct physiological stem-cell-based regeneration of antlers. Also, deer antlers have near-perfect generative wound healing, and these antler stem cells can rapidly accelerate cutaneous wound healing and the repair of injured tissue to restore physiological and anatomical function. Overall, antler regeneration is not only a natural wonder, but can also be brought into clinical application, shedding light on modern pharmaceutical studies to further prevent and treat diseases.
References
Amemiya, C.T., Jessica, A., Alison, P.L., Shaohua, F., Hervé, P., Iain, M., Ingo, B., Tereza, M.,
Igor, S., Nicolas, R., Chris, O., Domitille, C., Jeramiah, J.S., Mark, R., Rosemary, A.D., Marco, G., Bronwen, A., Maria, A.B., Marco, B., & Denis, B. (2013). The African Coelacanth Genome Provides Insights into Tetrapod Evolution. Nature, 496(7445) 311–316. https://doi.org/10.1038/nature12027
Banks, W. J., Epling, G. P., Kainer, R. A., & Davis, R. W. (1968). Antler growth and osteoporosis I. Morphological and morphometric changes in the costal compacta during the antler growth cycle. The Anatomical Record, 162(4), 387–397. https://doi.org/10.1002/ar.1091620401
Bubenik, A. (1968). The significance of the antlers in the social life of the Cervidae. Deer, 1(6), 208–214.
Caro, T.M., Graham, C.M., Stoner, C.J., & Flores, M.M. (2003). Correlates of horn and antler shape in bovids and cervids. In Behavioral Ecology and Sociobiology, 55(1), 32–41. https://doi.org/10.1007/s00265-003-0672-6
Chapman, D.I. (1975). Antlers–bones of contention. Mammal Review, 5(4), 121–172. https://doi.org/10.1111/j.1365-2907.1975.tb00194.x
Chapman, D.I. (1981). Antler structure and function—A hypothesis. Journal of Biomechanics, 14(3), 195–197. https://doi.org/10.1016/0021-9290(81)90026-9
Dong, Z., Haines, S., & Coates, D. (2020). Proteomic Profiling of Stem Cell Tissues during
Regeneration of Deer Antler: A Model of Mammalian Organ Regeneration. J. Proteome Res, 19(4), 1760–1775. https://doi-org.proxy3.library.mcgill.ca/10.1021/acs.jproteome.0c00026
Feleke, M., Bennett, S., Chen, J., Hu, X., Williams, D., & Xu, J. (2021). New physiological
insights into the phenomena of deer antler: A unique model for skeletal tissue regeneration. Journal of Orthopaedic Translation, 27, 57-66. https://doi.org/10.1016/j.jot.2020.10.012
Franco, C., Soares, R., Pires, E., Koci, K., Almeida, A.M., Santos, R. & Coelho, A.V. (2013).
Understanding regeneration through proteomics. Proteomics, 13(3–4), 686– 709. https://doi.org/10.1002/pmic.201200397
French, C.E., McEwen, L.C., Magruder, N.D., Ingram, R.H., & Swift, R.W. (1956). Nutrient requirements for growth and antler development in the white-tailed deer. The Journal of Wildlife Management, 20(3), 221. https://doi.org/10.2307/3796954
Gaspar-López, E., Landete-Castillejos, T., Estevez, J.A., Ceacero, F., Gallego, L., & García, A.J. (2008). Biometrics, testosterone, cortisol and antler growth cycle in Iberian red deer stags (Cervus elaphus hispanicus). Reprod. Dom. Anim., 45(2), 243-249. https://doi.org/10.1111/j.1439-0531.2008.01271.x
Goss, R. J. (1983). Deer antlers: regeneration, function and evolution. New York: Academic
Press.
Hall, K. B. (2015). Bones and Cartilage. Academic Press. https://doi.org/10.1016/B978-0-12-416678-3.00008-2
Hanfee, F. (2010). Wildlife Trade: A Handbook for Enforcement Staff. Natraj Publishers.
Heckeberg, S.N. (2017). Origination of Antlerogenesis. Journal of Morphology, 278, 182-202. https://onlinelibrary-wiley-com.proxy3.library.mcgill.ca/doi/pdfdirect/10.1002/jmor.20628
Hemmings, B.A., & Restuccia, D.F. (2012). PI3K-PKB/Akt pathway. Cold Spring Harb
Perspect Biol., 4(9), 11-189. https://doi.org/10.1101/cshperspect.a011189
Hillman, J.R., Davis, R.W., & Abdelbaki, Y.Z. (1973). Cyclic bone remodeling in deer. Calcified Tissue Research, 12(1), 323–330. https://doi.org/10.1007/bf02013745
HyvÄrinen, H., Kay, R. N.B., & Hamilton, W.J. (1977). Variation in the weight, specific gravity and composition of the antlers of red deer (Cervus elaphus L.). British Journal of Nutrition, 38(3), 301–311. Cambridge Core. https://doi.org/10.1079/BJN19770094
Ilic, D., & Polak, J.M. (2011). Stem cells in regenerative medicine: introduction. Br. Med. Bull.
98, 117–126, https://doi.org/10.1093/bmb/ldr012
Kierdorf, U., Kierdorf, H. (2005) Antlers as biomonitors of environmental pollution by lead
and fluoride: a review, Eur. J. Wildlife Res., 51, 137–150. https://doi.org/10.1007/s10344-005-0093-0
Kierdorf, H., & Kierdorf, U. (2002). Reconstruction of a decline of ambient lead levels in the Ruhr area (Germany) by studying lead concentrations in antlers of roe deer (Capreolus capreolus). Science of The Total Environment, 296(1), 153–158. https://doi.org/10.1016/S0048-9697(02)00073-6
Kierdorf, U., & Kierdorf, H. (2000). The fluoride content of antlers as an indicator of fluoride exposure in red deer (Cervus elaphus): A historical biomonitoring study. Archives of Environmental Contamination and Toxicology, 38(1), 121–127. https://doi.org/10.1007/s002449910015
Kolios, G., & Moodley,Y. (2013). Introduction to Stem Cells and Regenerative Medicine.
Respiration, 85(1), 3-10. https://doi.org/10.1159/000345615
Ko, K. M., Yip, T. T., Tsao, S. W., Kong, Y. C., Fennessy, P., Belew, M. C., & Porath, J. (1986).
Epidermal growth factor from deer (Cervus elaphus) submaxillary gland and velvet antler. General and comparative endocrinology, 63(3), 431–440. https://doi.org/10.1016/0016-6480(86)90143-7
Landete-Castillejos, T., Kierdorf, H., Gomez, S., Luna, S., García, A. J., Cappelli, J., Pérez-Serrano, M., Pérez-Barbería, J., Gallego, L., & Kierdorf, U. (2019). Antlers—Evolution, development, structure, composition, and biomechanics of an outstanding type of bone. Bone, 128, 115046. https://doi.org/10.1016/j.bone.2019.115046
Ledbetter, Macy. (2015, August 11). Antler Growth 101. Spring Greek Outdoor. https://springcreekoutdoors.com/blog/antler-growth-101/
Lee, D.E., Ayoub, N., Agrawal, D.K. (2016). Mesenchymal stem cells and cutaneous wound
healing: novel methods to increase cell delivery and therapeutic efficacy. Stem Cell Res Ther, 7(37). https://doi.org/10.1186/s13287-016-0303-6
Li, S., Jovelin, R., Yoshiga, T., Tanaka, R., & Cutter, A.D. (2014). Specialist versus
generalist life histories and nucleotide diversity in Caenorhabditis nematodes. Proc Biol Sci, 281(1777), 2013-2858. https://doi.org/10.1098/rspb.2013.2858
Meister, W. W. (1956). Changes in histological structure of the long bones of white-tailed deer (Odocoileus virginianus) during the growth of the Antlers. The Anatomical Record, 124(4), 709–721. https://doi.org/10.1002/ar.1091240407
Menon, V., Panjwani, R., Capilla, P., Sharma, A., & Ghosh, M. (1994). Wildlife Trade-A Handbook for Enforcement Staff. TRAFFIC India-WF India.
Moen, R. A., Pastor, J., & Cohen, Y. (1999). Antler growth and extinction of Irish elk. In Evolutionary Ecology Research 1(2), 235–249)
Miller, K. V., Marchinton, R. L., Beckwith, J. R., & Bush, P. B. (1985). Variations in density and chemical composition of white-tailed deer antlers. Journal of Mammalogy, 66(4), 693–701. https://doi.org/10.2307/1380795
National Institute of General Medical Sciences. (2022, May 5). Regeneration. https://nigms.nih.gov/education/fact-sheets/Pages/regeneration.aspx
Pais, I., & Jones Jr, J. B. (1997). The handbook of trace elements. CRC Press.
Price, J., & Allen, S. (2004). Exploring the mechanisms regulating regeneration of deer antlers. Philosophical Transactions of the Royal Society of London. Series B: Biological Sciences, 359(1445), 809–822. https://doi.org/10.1098/rstb.2004.1471
Pokorny, B., Jelenko, I., Kierdorf, U., Kierdorf, H. (2009). Roe deer antlers as historical
bioindicators of lead pollution in the vicinity of a lead smelter, Slovenia. Water, Air
Soil Pollut., 203(1-4), 317–324. https://doi.org/10.1007/s11270-009-0014-z
Purdey, M. (2004). Elevated silver, barium and strontium in antlers, vegetation and soils sourced from CWD cluster areas: Do Ag/Ba/Sr piezoelectric crystals represent the transmissible pathogenic agent in TSEs? Medical Hypotheses, 63(2), 211–225.
Rafferty, K., Davies, K. M., & Heaney, R. P. (2005). Potassium intake and the calcium economy. Journal of the American College of Nutrition, 24(2), 99–106. https://doi.org/10.1080/07315724.2005.10719450
Rong, X., Chu, W., Zhang, H., Wang, Y., Qi, X., Zhang, G., Wang, Y. & Li, C. (2019). Antler
stem cell-conditioned medium stimulates regenerative wound healing in rats. Stem Cell Res Ther, 10(326). https://doi.org/10.1186/s13287-019-1457-9
Singer, A.J., & Clark, R.A. (1999). Cutaneous wound healing. N Engl J Med, 341, 738–746.
https://doi.org/10.1056/nejm199909023411006
Singh, R. R., Khanna, P. P., Singh, A. K., & Goyal, S. P. (2022). Elemental characterization of antlers of various deer species using X-Ray Fluorescence (XRF): A tool for forensic examination. Forensic Science International, 332, 111-172. https://doi.org/10.1016/j.forsciint.2022.111172
Stuttfeld, E., & Ballmerhofer, K. (2010). Structure and function of VEGF receptors. Iubmb
Life, 61(9), 915–922. https://doi.org/10.1002/iub.234
Sunwoo, H. H., Nakano, T., Hudson, R. J., & Sim, J. S. (1995). Chemical composition of antlers from wapiti (Cervus elaphus). Journal of Agricultural and Food Chemistry, 43(11), 2846–2849. https://doi.org/10.1021/jf00059a014
Tajchman, K., Bogdaszewski, M., & Kowalczuk-Vasilev, E. (2020). Effects of supplementation with different levels of calcium and phosphorus on mineral content of first antler, bone, muscle, and liver of farmed fallow deer (dama dama). Canadian Journal of Animal Science, 100(1), 17–26. https://doi.org/10.1139/cjas-2018-0234
Tataruch, F. Red deer antlers as biomonitors for lead contamination (1995). Bull. Environ. Contam. Toxicol. 55, 332–337. https://doi.org/10.1007/BF00206669
Vashishth, D. (2004). Rising crack-growth-resistance behavior in cortical bone: Implications for toughness measurements. Journal of Biomechanics, 37(6), 943–946. https://doi.org/10.1016/j.jbiomech.2003.11.003
Volarevic, V., Markovic, B.S., Gazdic, M., Volarevic, A., Jovicic, N., Arsenijevic, N.,
Armstrong, L., Djonov, V., Lako, M., & Stojkovic, M. (2018). Ethical and Safety Issues of Stem Cell-Based Therapy. Int J Med Sci, 15(1), 36-45. https://doi.org/10.7150/ijms.21666
Walker, D., White, D., & Roubin, R. (2001). Deer Antler: Velvet Research in Australia and Overseas. Rural Industries Research and Development Corporation.
Wang, D., Ba, H., Li, C., Zhao, Q., & Li, C. (2018). Proteomic Analysis of Plasma Membrane
Proteins of Antler Stem Cells Using Label-Free LC(−)MS/MS. Int. J. Mol. Sci., 19(11), 3477. https://doi.org/10.3390/ijms19113477
Williams, E. S. (2005). Chronic Wasting Disease. Veterinary Pathology, 42(5), 530–549. https://doi.org/10.1354/vp.42-5-530
Zhao, A., Qin, H., & Fu, X. (2016). What Determines the Regenerative Capacity in Animals?
BioScience, 66(9), 735– 746. https://doi.org/10.1093/biosci/biw079
Zioupos, P., Currey, J. D., & Sedman, A. J. (1994). An examination of the micromechanics of failure of bone and antler by acoustic emission tests and Laser Scanning Confocal Microscopy. Medical Engineering & Physics, 16(3), 203–212. https://doi.org/10.1016/1350-4533(94)90039-6