Abstract
The designs that enable arteries and veins to execute their functions are the products of evolutionary processes. This report explores this notion within the framework of biomolecular, cellular, and tissue engineering. Nitric oxide will be presented as an example of how blood vessels employ biochemicals to maintain homeostasis. Biosensor design will be examined as a vehicle to obtain data on the properties of nitric oxide that suit it to vascular regulation. The major cell types that dictate vessel function, endothelial and smooth muscle cells, will be presented as heterogenous and abundant structures. Vascular tissue engineering will be discussed as a means to solving problems that arise in cardiovascular function. Lastly, conclusions drawn about the complementary relationship between design and function will be extended to examine the current state and outlook of tissue engineering.
Biomolecules: Nitric Oxide
There are countless biochemicals involved in the function of veins and arteries, including epinephrine and acetylcholine, as discussed in “Comparative Analysis of the Biological Mechanics of Veins and Arteries in Animals” (Aniche et al., 2020). Biomolecules are essential to all biological processes. In the vascular system, biochemicals influence the physical state of vessels and their function to ensure efficient operation. One of the most important and fascinating chemicals is nitric oxide, which will be used to illustrate the effects of biochemicals on blood vessels.
Function
Nitric oxide (NO) is an essential biochemical capable of regulating the structure and function of veins and arteries. Nitric oxide is continuously being synthesized in endothelial cells through a series of reduction-oxidation reactions. Synthesis of NO is dependent on calcium ions, which are released by the shear forces from blood flowing in endothelial cells. This activates the nitric oxide synthase enzyme. In the presence of oxygen and nicotinamide adenine dinucleotide phosphate (NADPH), this transforms L-arginine into L-citrulline and NO (Furchgott and Zawadzki, 1980). Subsequently, NO can be used to communicate with cells in numerous ways, having two primary impacts on the vascular system: inducing vasodilation – relaxation – and controlling the oxygen consumption of mitochondria (Chen et al., 2008).
The first impact of nitric oxide is vasodilating veins and arteries. It diffuses to smooth muscle cells and binds to the enzyme guanylate cyclase. This induces a change in the enzyme’s conformation, catalyzing the conversion of guanosine triphosphate into cyclic guanosine monophosphate (cGMP) inside the smooth muscle cells (Calver et al., 1992). cGMP activates protein kinase G, which activates myosin phosphatase, releasing calcium stored in the smooth muscle cells, and finally relaxing the cells (“Cyclic GMP” | Nitric oxide research group | University of Reading, n.d.). Fig. 1 summarizes the complex biochemical pathway of NO through the vascular system. Most importantly, the presence of NO induces the relaxation of blood vessels, allowing them to regulate blood flow through various mechanical properties.
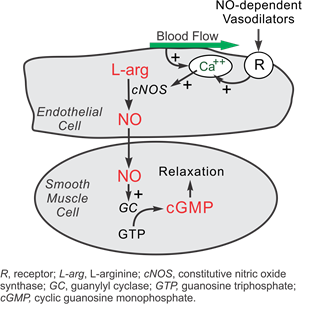
In vivo experiments in guineapigs, mice, and various other animals shows that inhibiting the synthesis of NO results in vasoconstriction of blood vessels and a rise in blood pressure (Aisaka et al., 1989). Thus, blood vessels vasodilate when NO concentrations are high and vasoconstrict when NO concentrations are low. Altering the dilation of veins and arteries is essential in controlling the distribution of blood throughout the body and adapting to various external stimuli.
The intricacy of the relationship between blood flow, dilation, and NO concentrations demonstrates nature’s ability to design efficient biochemical systems. When shear forces are high, blood is flowing quickly and in high volumes. To accommodate the high volume of blood, the vessels must relax. Luckily, the production of NO is dependent on calcium ions, and more calcium ions are released when shear forces are high. Consequently, more NO is produced when shear forces are high, regulating the physical state of veins and arteries to meet the needs of the system. Evidently, NO has a pivotal impact on the physical state and function of blood vessels.
On the organelle level, nitric oxide plays a role in cellular respiration rates and oxygen delivery. Cytochrome c is an electron transporter protein found in the inter-cristal space of mitochondria (Garrido et al., 2006). NO competes with oxygen to bind with cytochrome c, and therefore the oxygen consumption of tissues depends on the local NO concentration. Higher NO concentrations result in lower mitochondrial cellular respiration and thus oxygen consumption, as seen quantitatively in Fig. 2. The presence of nitric oxide in blood vessels is essential, as it ensures the arteries themselves do not over-consume oxygen, especially under hypoxic conditions (Chen et al., 2008). Since oxygen and NO are competing, under low oxygen conditions, less nitric oxide is needed to regulate cellular respiration rates; thus, arteries consume less of the oxygen contained in blood and deliver more to the organs in need. The relationship between oxygen, nitric oxide, and cellular respiration allows arteries to regulate the quantity of oxygen they deliver under various conditions.

In summary, nitric oxide is one of the most important regulator biomolecules in blood vessels. High NO concentrations induce relaxation in blood vessels. The absence of NO results in increased blood pressure and constriction of vessels. Further, its synthesis is regulated by blood flow rate, allowing vasodilation to be regulated by the needs of the system. NO competitively inhibits cytochrome c, an enzyme essential in cellular respiration. This is vital to blood vessels consuming less oxygen and delivering it to various organs under hypoxic conditions. Since NO plays a pivotal role in the function of blood vessels, it is important to quantify the relationship between nitric oxide concentration, its distribution throughout the vascular system, and the physical state of blood vessels. Quantifying this relationship heavily relies on bioengineering.
Nitric Oxide Biosensor Design
To explore the impact NO has, it is crucial to accurately determine its concentration, and hence carefully designed biosensors must be used. Many other biological processes use nitric oxide and the data obtained is not limited to the vascular system. Biosensors must be designed to a sufficient sensitivity, specificity, and linearity (Wachsmann-Hogiu, 2020). Designing NO biosensors is difficult since other biomolecules have similar electric properties, and slight concentration differences greatly impact its influence on the vascular system; differences of less than one nanoMolar (nM) triggers the vascular relaxation mechanism, changing the state and function of blood vessels (Hall and Garthwaite, 2009). Many approaches can be taken to design biosensors to construct a clear picture of nitric oxide throughout vascular tissues.
The first approach uses electrodes as electric transducers. Selectivity must be achieved since many other biochemicals, such as dopamine and nitrite, have very similar redox potentials (Hall and Garthwaite, 2009). The effects of interfering chemicals can be reduced by coating the electrodes with members impermeable to charged species, such as Nafion and ο-phenylenediamine. This ensures nitrite and dopamine, which are charged, will not influence the detected concentration of NO. However, the coatings can be problematic, and thus the integrity of the biosensors may be compromised. Bioengineers must carefully choose their electrode coating and include the intrinsic uncertainty of the material when detecting NO. The level of uncertainty makes it problematic to confidently use electrodes to determine NO concentrations, and thus other approaches are also explored.
Fluorescent probes are another approach at biosensing for NO concentration and provide in depth data. These biosensors use optical transducers, emitting light in the presence of nitric oxide. Two classes of biochemicals are commonly used, namely diamino derivatives of fluorescein (DAF) and rhodamine (DAR) (Kojima et al., 1998). Some examples are shown below in Fig. 3.
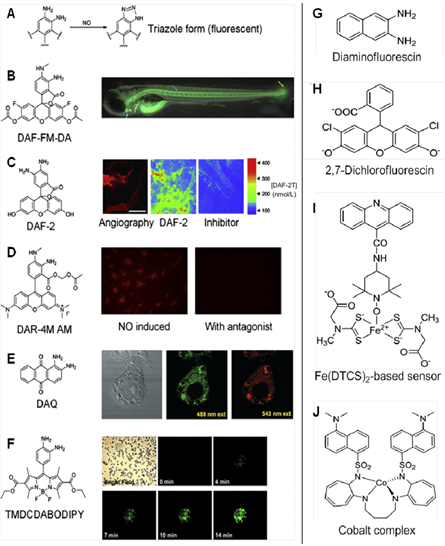
4,5-diaminofluorescein is one commonly fluorescent probe, chosen for its high levels of sensitivity and selectivity. It reacts in the presence of NO to form DAF-2 triazole (DAF-2T), a highly fluorescent species, illustrated in Fig. 4. The fluorescent emissions for 4,5-diaminofluorescein are graphed at varying concentrations of NO in Fig. 5. Experimental data shows that DAF-2 fluorescent biosensors are accurate to the nM scale and can help to provide an accurate picture of NO distribution (Uhlemhut and Högger, 2012). As researchers continue to explore different fluorescent probes, the investigation for the most efficient and accurate means on biosensing for nitric oxide continues.
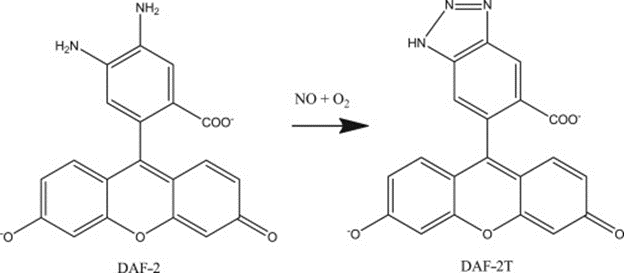

In summary, electrodes and fluorescence are the most common approaches to nitric oxide biosensor design. When engineering biosensors, it is essential to consider selectivity and sensitivity to obtain accurate data. These biosensors are used to understand the relationship between NO concentration and the regulation of blood flow throughout the body. This relationship is important to understand the influence of nitric oxide on blood vessels as it is an amazing and adaptive biomolecule essential to vascular regulation.
Vessel Tissue: A Common Histological Organization
As a result of evolutionary processes, local functional factors have caused vessels to undertake segmental differentiations to suit their operation. However, the components of the vascular system present a common histological organization in the tissues that comprise them. Three concentric layers of tissue characterize the vascular wall: tunica intima, media, and adventitia, ordered from the lumen outward.
Tunica intima contains a layer of endothelium, subendothelial layer, and fenestrated layer of a network of elastic fibers (“Blood Vessels Lab” | Histology @ Yale | Yale University, n.d.). Smooth muscle cells, an external elastic lamina, and collagen fibers compose tunica media (“Blood Vessels Lab” | Histology @ Yale | Yale University, n.d.). A simple squamous epithelium, basement membrane, and vasa vasorum make up the adventitia layer (Alberts et al., 2002).
Vascular Endothelial Cells: Shared and Heterogenous Structures
Displayed in Fig. 6, a monolayer of endothelial cells (ECs) is a shared feature of the tunica intima of all blood vessels (Alberts et al., 2002). Since they surround the inner vessel lumen, ECs contact the mechanical forces, hormones, and noxious substances of the blood directly (Hirashima and Suda, 2006). As a result, they have important functions in governing vascular resistance and growth, thrombogenesis, and inflammation (Hirashima and Suda, 2006).
Molecular properties that are common to all vascular endothelial cells include positive tests for von Willebrand factor (vWF) and CD31 glycoprotein (de Jong et al., 2019). vWF is a large, multimeric glycoprotein responsible for the adhesion of platelets to the sub-endothelium following vascular injury and the stabilization of the coagulation factor VIII (de Jong et al., 2019). CD31is a transmembrane glycoprotein located at intercellular junctions and functions in the adhesion of ECs (de Jong et al., 2019).
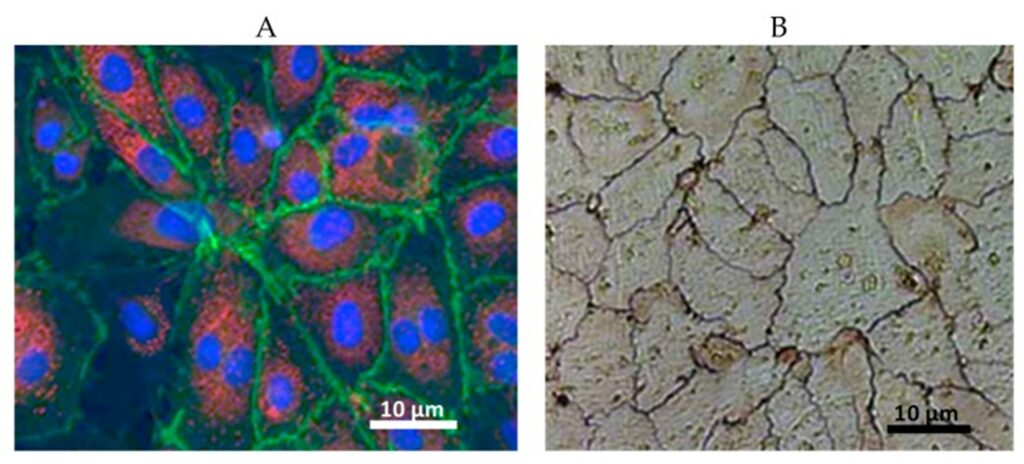
The pathophysiological responses of ECs to stimulation in different vascular beds and between different sections of the same bed reveals a defining property of the cell: heterogeneity (Jambusaria et al., 2020). The architectural, operational, and functional diversity of endothelial cells throughout the vascular tree requires tailor-made therapies for the prevention and treatment of disease. As a result, the engineering of novel targeted therapies for vascular dysfunction relies on advanced screening methods to characterize this heterogeneity (Jambusaria et al., 2020).
Arterial Versus Venous Endothelial Cells
The different functions of the arterial and venous systems correspond to design variations in their endothelial cells, reflecting their heterogeneity once again. Studies have been conducted to examine the phenotypic diversity of vascular endothelial cells through genetic profiling, whose results are displayed in Fig. 7 (Chi et al., 2003). The ECs of arteries are long, slender, or ellipsoidal in the direction of undisturbed blood flow (dela Paz and D’Amore, 2008). Conversely, they are shorter and wider in veins to reflect the lower rates of blood flow and shear stress levels (dela Paz and D’Amore, 2008). Although arterial ECs are predominantly thicker, the cuboidal cells of high endothelial venules (HEVs) serve as exceptions (dela Paz and D’Amore, 2008).
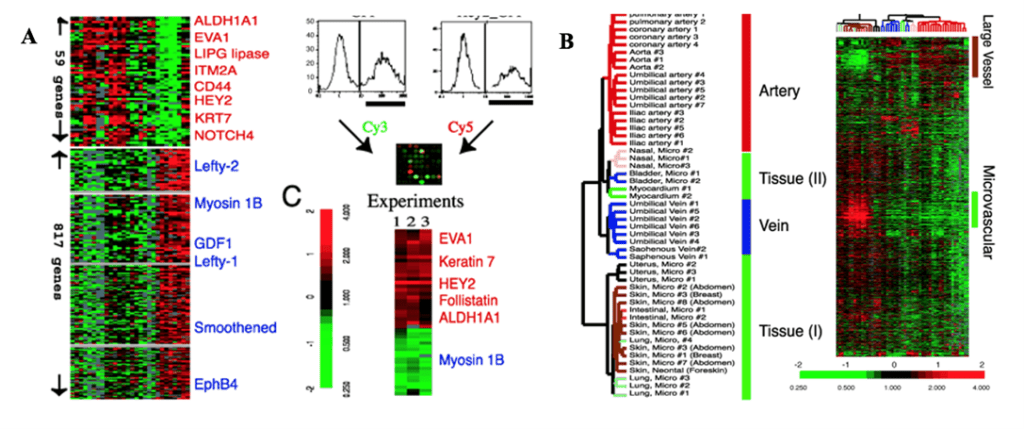
Structural diversity also exists between the endothelial cells of pulmonary and systemic arteries to reflect their different responses to changes in ambient oxygen levels. While reduced oxygen levels cause pulmonary vascular beds to vasoconstrict, this same stimulus causes systemic vessels to vasodilate (Sandoo et al., 2010). This variant suggests a difference in the cells that are situated within the wall to first detect the change in concentration: ECs (Sandoo et al., 2010). A study performed to characterize the morphology of the blood vessels of rats confirms this, where aortic ECs were reported to be long and narrow with dimensions of 55 x 10 μm while the pulmonary artery ECs displayed sizes of 30 x 14 μm (Aird, 2007).
The expression and organization of intercellular junctions also varies between arteries and veins. The junctions are involved in intercellular adhesion and communication, and they differ according to the site-specific functional needs of the vessel (Dejana et al., 2008). The junctions in arteries are tighter and present higher levels of organization than veins (Dejana et al., 2008). A comparative analysis of the endothelial cells across the vascular tree produces the conclusion that local environment conditions and genetic determinants suit structure to operation.
Vascular Smooth Muscle Cells: Abundant Cells
Vascular smooth muscle cells (VSMCs) located in the tunica media represent the most abundant cell type in blood vessels. As displayed in Fig. 8, venous and arterial SMCs present varying phenotypes (Wong et al., 2005). VSMCs have important roles in vasoconstriction, vasodilation, and the synthesis of the extracellular matrix (Rensen et al., 2007). They associate with elastic lamellae and laminae to produce concentric and helically arranged bundles (Rensen et al., 2007). The cells are further classified into synthetic and contractile structures with varying morphologies and functions (Rensen et al., 2007). Contractile SMCs present muscle-like appearances and contract with mechanical and biochemical stimuli to regulate pressure and flow (Wong et al., 2005). Synthetic SMCs resemble fibroblasts and are involved in the production of the extracellular matrix (Wong et al., 2005).

Tissue Engineering of Blood Vessels
Tissue Engineering: General Definition
With our current medical capacities, organ damage or tissue loss are only treated with transplantation, surgery or mechanical device applications such as dialysis (Bakhshandeh et al., 2017). However, these treatments have limitations, and the world is also confronted with an organ shortage. Therefore, it is paramount to develop alternative strategies like tissue engineering and regenerative medicine.
Tissue engineering is defined as an “interdisciplinary field that applies the principles of engineering and the life sciences toward the development of biological substitutes that restore, maintain, or improve tissue function” by Langer and Vacanti in 1993. They also described three strategies essential to the creation of new tissue in vitro (Carvalho et al., 2013; Bakhshandeh et al., 2017):
- Isolating an appropriate cell source, suitable for the type of tissue that needs to be created.
- Operating an effective cell modification; this includes tissue inducing substances like growth factors and other biomolecules that ensure proper formation of tissue.
- Using an appropriate supportive matrix; this can provide with an adequate tissue formation and fixation, and mechanical support.
In subsequent parts of this report, we will discuss the applications of these three strategies, illustrated in Fig. 9, in vascular tissue engineering.
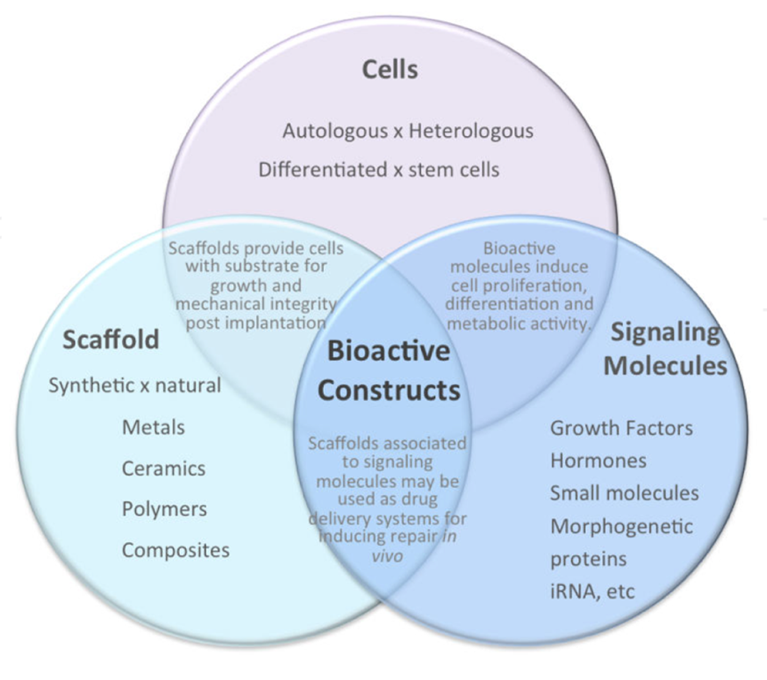
Vascular Tissue Engineering: An Overview
Development of effective vascular tissue engineering methods is important to address potential problems in the function of the vascular system. Furthermore, their applications exceed the treatment of cardiovascular diseases, such as for revascularization of graft vessels after organ transplantation, which directly affects their viability. Indeed, tissue engineering provides with biocompatible, non-thrombogenic, solutions, with low infection and low rejection rates (Zeng et al., 2019).
The development of the vascular tissue engineering field first began 40 years ago, however, early attempts to tissue engineered blood vessels (TEBVs) were quite unsuccessful and did not have any clinical uses. This was due to the focus on using isolated compounds such as collagen gels to produce them (Dimitrievska and Niklason, 2018). Tubular collagen gels seeded with endothelial cells were first used in 1986 but the constructs that resulted had poor mechanical strength and did not possess all the characteristics of specialized arteries. In the following years, other researchers aimed to create blood vessels by isolating specific proteins and cells. However, these methods still resulted in TEBVs with poor mechanical strength. Because of this, subsequent trials, in the 1990’s, were concentrated on the use of synthetic scaffolds to provide a more appropriate structure (Peck et al., 2012).
Nowadays, the goal of vascular tissue engineering is to design entirely biological vessels that have properties identical or at least similar to the native tissue where they are aimed to be transplanted in. These properties include size, non-thrombogenic and nonimmunogenic surfaces in contact with blood, similar material and mechanical characteristics, a capacity for vasoconstriction and vasodilation and a high regeneration and integration rate (Dimitrievska and Niklason, 2018; Fernandez et al., 2014).
Cells Sources and Differentiation
Vascular tissue engineering techniques vary depending on the diameter of the desired TEBV. They are generally classified into three categories of size: micro-vessels, when the diameter is smaller than 1 mm, small vessels, with a diameter ranging from 1 to 6 mm, and large vessels which have a diameter greater than 6 mm (Chang and Niklason, 2017).
Choosing an appropriate cell source is generally the first step to proper tissue engineering of blood vessels. Different types of cells will give different structural properties to the conduit formed, and impact in vivo integration once transplanted. To mimic the tissue structure of a vessel, three main types of cells that are needed are endothelial cells, smooth muscle cells and fibroblasts, which synthesize collagen and are responsible for producing the extra cellular matrix (Fig. 10). These can be obtained through differentiation of stem cells, which are capable of many cell divisions. Multiple types of stem cells exist, and generally, autologous sources are preferred since they minimize the risk of rejection (Fernandez et al., 2014).
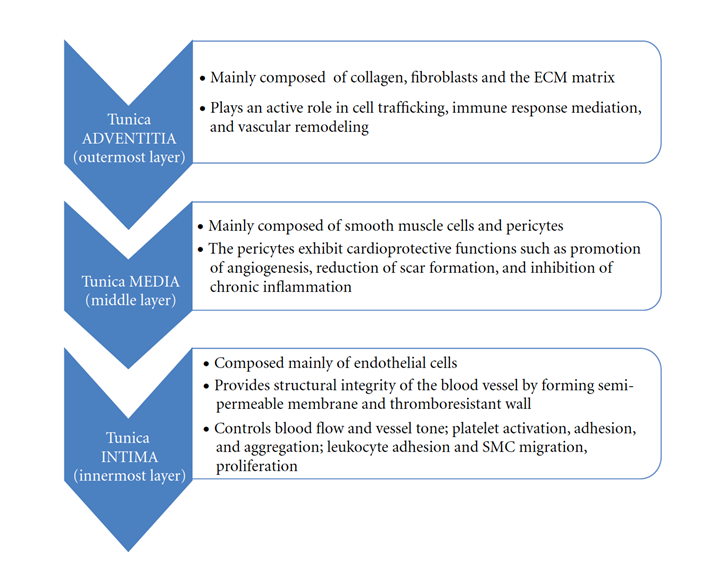
Embryonic stem cells (ESCs), which are pluripotent cells, meaning they can differentiate in all types of cells, are a very effective source of seeding cells for TEBVs, particularly to obtain smooth muscle cells. When exposed to specific growth factors, including TGFb-1, ESCs can be derived into mesenchymal stem cells (MSCs), then into smooth muscle cells. MSCs are multipotent as opposed to pluripotent. This means that they can differentiate in many, but not all types of cells in the body. TGFb-1 is a growth factor that is involved in cell growth, proliferation and differentiation. The smooth muscle cells obtained can produce collagen and form a connective tissue-like construct when maintained for a long period of time. However, during this differentiation process, chondrocytes may also be produced. These cells are irrelevant to vascular tissue engineering as they are involved in the production of cartilage extracellular matrix, so purification is a required step of the process (Zeng et al., 2019). In addition, under certain conditions, smooth muscle cells also run the risk of trans-differentiating into chondrocytes after implantation in the body. This, coupled with the surrounding ethical issues and the difficulty to retrieve ESCs in sufficient numbers for a clinical application, are the main disadvantages to using ESCs, making the current focus shift towards adult stem cells, including adult MSCs, extracted from bone marrow (Zeng et al., 2019; Schutte and Nerem, 2013).
To obtain endothelial cells (ECs), many methods can be employed. ECs are especially important for engineering micro and other small vessels since they are more prone to thrombosis and require the anti-thrombogenic properties of ECs (Fig. 10). Autologous ECs can be extracted from the jugular or saphenous vein. Unfortunately, these procedures are invasive, and the lifespan of extracted cells is very short. Alternatives such as differentiating adult endothelial progenitor cells to ECs exist. The latter type of cell is release from bone marrow and participate in angiogenesis and damage repair by differentiating into endothelial cells. They can be used to construct TEBVs of small diameter and recent studies have shown a high rate of integration in the vessel (Zeng et al., 2019; Peck et al., 2012; Fernandez et al., 2014).

Finally, fibroblasts can be obtained through skin biopsies of patients and cultured in a nutrients rich environment to produce TEBVs. However, recent developments in dermal tissue engineering have shown that allogeneic sources of fibroblasts do not trigger immune responses. This discovery could facilitate production by opening the possibility to produce cultures in greater batches and that are readily available (Peck et al., 2012). Further studies must be conducted to understand the applications of this discovery to vascular tissue engineering.
Scaffolds and Assembly
A suitable cell source is not sufficient to provide therapeutic effects once transplanted in the body as older studies demonstrated. Although this can have regenerative effects to a certain extent, delivering differentiated cells will not lead to an integrated vasculature with the host tissue. For this reason, as stated before, the current focus is to create a functional vessel, that possesses the architectural and functional characteristics of the host vasculature, before implantation (Song et al., 2018). Three approaches that are the most commonly used to produce TEBVs will be discussed. A summary of these techniques is given in Fig. 12.
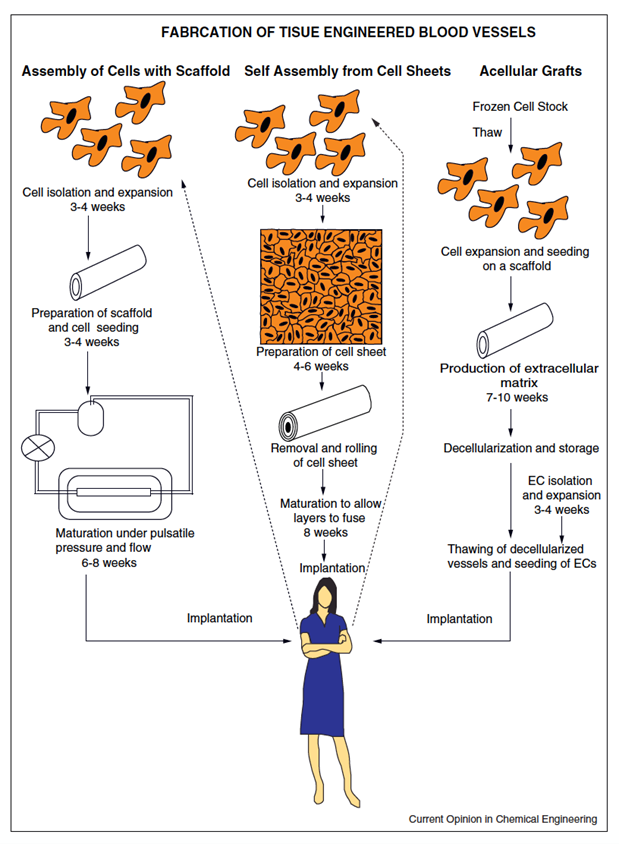
The in vitro assembly of precultured cells within synthetic or biological scaffolds results in a three-dimensional structure able to form an extra cellular matrix. This is a top-down approach, where the final geometry and architecture of the vessel is predetermined. The scaffold is prefabricated before cells are introduced. After implantation of the cells in the scaffold, the assembly is exposed to physiological stimuli (pulsatile pressure and flow), such as fluid shear stress, which prompts ECs to produce nitric oxide and prostacyclin, which possess anti-thrombogenic properties and promote vasodilation by smooth muscle cells. This is particularly important for small and micro-vessels as they are more prone to thrombosis. When exposed to specific conditions of shear stress, ECs can undergo further differentiation into artery specific ECs (Fernandez et al., 2014; Nemeno-Guanzon et al., 2012).
If a synthetic scaffold is used, the polymer must be degradable to promote biocompatibility – tissue growth and remodeling. – Indeed, when non degradable polymers like polytetrafluoroethylene (PTFE) are used, they cause a long-term foreign body response from the immune system, hindering the success and durability of the graft. Synthetic biocompatible types of scaffolds include polycaprolactone (PCL), polyglycolic acid (PGA), and polylactic acid (PLA). They are usually designed into three-dimensional porous tubes that can easily be seeded with cells. These sorts of structures stimulate the synthesis of extracellular matrix by the cells, improving the mechanical strength of the conduit (Dimitrievska and Niklason, 2018).
The second method is a top-down approach and involves in vivo vessel formation thanks to an implantation of acellular grafts after decellularizing cultured blood vessels. Blood vessels are mostly developed in a manner similar to the previous technique before they are washed with mixtures of detergents, buffers and enzymes to remove cellular components. Thus, allogeneic human cells can be used to produce these vessels since antigens will be removed during this step. After the washing process, what remains is the extracellular matrix so the tissue stays highly biocompatible and structurally the same, although slight atrophy can sometimes happen. The ability to use allogeneic cells opens up the possibility to produce acellular grafts ahead of time and store them, increasing their clinical availability. Acellular scaffolds can be incorporated with biomolecules that will slowly release once the graft is implanted in the body. They can contribute to the recruitment, and proliferation of neighboring cells to accelerate repair (Dimitrievska and Niklason, 2018; Fernandez et al., 2014; Zeng et al., 2019; Nemeno-Guanzon et al., 2012).
However, acellular TEBVs are efficient only for large vessels, as endothelial cell seeding of the inner surface, or the application of a chemical coat, before implantation, is required for smaller diameter to prevent thrombosis (Dimitrievska and Niklason, 2018; Fernandez et al., 2014). This is illustrated in Fig. 13.

Finally, in vitro assembly of cellular sheets will be considered. This method allows for the creation of a completely biological vessel. Smooth muscle cells were cultured to produce a cellular sheet. Ascorbic acid was introduced in the culture to increase collagen synthesis. The sheet is then placed around a tubular structure and a similar fibroblast cell sheet is stacked above it, which will form the tunica adventitia. The structure is then left to mature for a few weeks, and the two layers will fuse together. After removal of the tubular support, ECs are seeded in the lumen to form the tunica intima. The resulting TEBVs possesses a well-defined structure similar to the natural structure of blood vessels. The endothelial cell layer even displayed expression of von Willebrand factor (vWF). The first TEBVs using the cellular sheet technology also displayed an amazing mechanical strength and produced a robust tissue, as shown in Fig. 14. Its burst strength was 2594 ± 501 mmHg which far exceeds the burst strength of many human vessels. In comparison, the burst strength of the saphenous vein, commonly used for bypass grafting, is 1680 ± 307 mmHg (Zeng et al., 2019; Peck et al., 2012; Dimitrievska and Niklason, 2018).
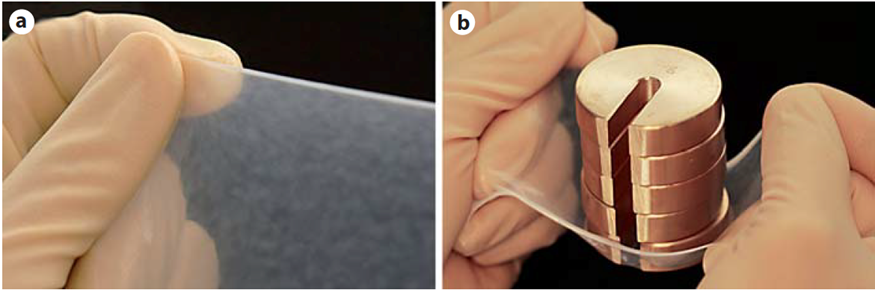

Conclusion
Nitric oxide is used as an example to reveal the complicated yet sophisticated ways which blood vessels synthesize and use biochemicals to maintain homeostasis. Through an elaborate biochemical pathway, NO acts as a vasodilator, relaxing and decreasing the pressure in veins and arteries. The rate of blood flow is tied to the production of NO, ensuring vasodilation occurs only when necessary. Low concentrations of NO result in vasoconstriction. By competing with oxygen to bind with cytochrome c, nitric oxide inhibits cellular respiration to preserve oxygen and deliver it to essential organs. Evidently, nitric oxide’s impact on the vascular system depends on specific conditions, ensuring it efficiently regulates function. It is crucial to understand how various concentrations of nitric oxide effect the relaxation of veins and arteries. Meeting the necessary requirements of such biosensors is a challenging feat, as they must be highly selective and sensitive. Electrodes and fluorescent probes are considered as accurate approaches to biosensor design.
The primary cell types of vascular tissue exhibit varying phenotypes; vascular endothelial cells are shared and heterogeneous structures that display structural diversity. Vascular smooth muscle cells also differ in their morphology. Numerous biochemicals are involved in the function and regulation of veins and arteries. Vascular tissues have properties that allow them to properly circulate blood around the body. Arteries have thick walls and are flexible to compensate for the high blood pressure, while capillaries are extremely small to ensure a smooth transition between high-pressured arteries and low-pressured veins.
Vascular tissue engineering has immensely improved since the very first tissue engineered blood vessel. It now offers an innovative approach to solving current medical limitations. Tissue engineered blood vessels must withstand the condition inherent to the body once transplanted to be viable. This includes shear stress and blood pressure. They must also possess similar biological properties as native bloods vessels, including thrombogenic and immunogenic responses, and an ability to integrate with host tissue. Choosing the right stem cell source is an important step of the process as it can directly influence the mechanical and biomolecular properties of the grafts. Existing technologies for engineering a blood vessel are very effective and provide a wide range of solutions depending on the clinical case. These range from acellular grafts to fully biological conduits, along with hybrid vessels, formed by cellular seeding of synthetic scaffolds. However, many advances still need to be made. A faster production time and more reliable results could allow vascular tissue engineering to become a viable solution for cardiovascular diseases. Biomolecular, cellular, and tissue engineering perspectives can be applied to study the multifaceted and diverse nature of veins and arteries. Engineering allows for a quantifiable and in-depth examination of their chemical and tissue properties.
References
Aird, W. C. (2007). Phenotypic heterogeneity of the endothelium: I. Structure, function, and mechanisms. Circulation Research, 100(2), 158-173. doi:10.1161/01.RES.0000255691.76142.4a
Aisaka, K., Gross, S. S., Griffith, O. W., & Levi, R. (1989). NG-methylarginine, an inhibitor of endothelium-derived nitric oxide synthesis, is a potent pressor agent in the guinea pig: does nitric oxide regulate blood pressure in vivo? Biochem Biophys Res Commun, 160(2), 881-886. doi:10.1016/0006-291x(89)92517-5
Alberts, B., Johnson, A., & Lewis, J. (2002). Blood Vessels and Endothelial Cells. In Molecular Biology of the Cell (4 ed.): Garland Science.
Aniche, A., Paliwal, D., Laflamme, H., & Raabel, J. (2020). Comparative Analysis of the Biological Mechanics of Veins and Arteries in Animals. Bioengineering. McGill University. Montreal, Canada. https://bioengineering.hyperbook.mcgill.ca/comparative-analysis-of-the-biological-mechanics-of-veins-and-arteries-in-animals/
Bakhshandeh, B., Zarrintaj, P., Oftadeh, M. O., Keramati, F., Fouladiha, H., Sohrabi-Jahromi, S., & Ziraksaz, Z. (2017). Tissue engineering; strategies, tissues, and biomaterials. Biotechnology and Genetic Engineering Reviews, 33(2), 144-172. doi:10.1080/02648725.2018.1430464
Brown, G. C. (2001). Regulation of mitochondrial respiration by nitric oxide inhibition of cytochrome c oxidase. Biochimica et Biophysica Acta (BBA) – Bioenergetics, 1504(1), 46-57. doi:10.1016/S0005-2728(00)00238-3
Calver, A., Collier, J., & Vallance, P. (1992). Nitric oxide and blood vessels: physiological role and clinical implications. Biochemical Education, 20(3), 130-135. doi:10.1016/0307-4412(92)90048-Q
Carvalho, J., Herthel, P., Gomes, D., & Góes, A. (2013). Innovative Strategies for Tissue Engineering. In (pp. 295-313).
Chang, W. G., & Niklason, L. E. (2017). A short discourse on vascular tissue engineering. npj Regenerative Medicine, 2(1), 7. doi:10.1038/s41536-017-0011-6
Chen, K., Pittman, R. N., & Popel, A. S. (2008). Nitric oxide in the vasculature: where does it come from and where does it go? A quantitative perspective. Antioxidants and Redox Signaling, 10(7), 1185-1198. doi:10.1089/ars.2007.1959
Chi, J.-T., Chang, H. Y., Haraldsen, G., Jahnsen, F. L., Troyanskaya, O. G., Chang, D. S., . . . Brown, P. O. (2003). Endothelial cell diversity revealed by global expression profiling. Proceedings of the National Academy of Sciences, 100(19), 10623-10628. doi:10.1073/pnas.1434429100
de Jong, A., Weijers, E., Dirven, R., de Boer, S., Streur, J., & Eikenboom, J. (2019). Variability of von Willebrand factor-related parameters in endothelial colony forming cells. Journal of thrombosis and haemostasis : JTH, 17(9), 1544-1554. doi:10.1111/jth.14558
Dejana, E., Orsenigo, F., Molendini, C., Baluk, P., & McDonald, D. M. (2009). Organization and signaling of endothelial cell-to-cell junctions in various regions of the blood and lymphatic vascular trees. Cell Tissue Res, 335(1), 17-25. doi:10.1007/s00441-008-0694-5
dela Paz, N. G., & D’Amore, P. A. (2009). Arterial versus venous endothelial cells. Cell Tissue Res, 335(1), 5-16. doi:10.1007/s00441-008-0706-5
Dimitrievska, S., & Niklason, L. E. (2018). Historical Perspective and Future Direction of Blood Vessel Developments. Cold Spring Harbor Perspectives in Medicine, 8(2). doi:10.1101/cshperspect.a025742
Fernandez, C. E., Achneck, H. E., Reichert, W. M., & Truskey, G. A. (2014). Biological and engineering design considerations for vascular tissue engineered blood vessels (TEBVs). Current opinion in chemical engineering, 3, 83-90. doi:10.1016/j.coche.2013.12.001
Furchgott, R. F., & Zawadzki, J. V. (1980). The obligatory role of endothelial cells in the relaxation of arterial smooth muscle by acetylcholine. Nature, 288(5789), 373-376. doi:10.1038/288373a0
Garrido, C., Galluzzi, L., Brunet, M., Puig, P. E., Didelot, C., & Kroemer, G. (2006). Mechanisms of cytochrome c release from mitochondria. Cell Death and Differentiation, 13(9), 1423-1433. doi:10.1038/sj.cdd.4401950
Goshi, E., Zhou, G., & He, Q. (2019). Nitric oxide detection methods in vitro and in vivo. Medical Gas Research, 9(4), 192-207. doi:10.4103/2045-9912.273957
group, N. o. r. (n.d.). Cyclic GMP. Retrieved from https://www.reading.ac.uk/nitricoxide/intro/no/cgmp.htm
Hall, C. N., & Garthwaite, J. (2009). What is the real physiological NO concentration in vivo? Nitric Oxide, 21(2), 92-103. doi:10.1016/j.niox.2009.07.002
Hirashima, M., & Suda, T. (2006). Differentiation of arterial and venous endothelial cells and vascular morphogenesis. Endothelium, 13(2), 137-145. doi:10.1080/10623320600698078
Jambusaria, A., Hong, Z., Zhang, L., Srivastava, S., Jana, A., Toth, P. T., . . . Rehman, J. (2020). Endothelial heterogeneity across distinct vascular beds during homeostasis and inflammation. Elife, 9. doi:10.7554/eLife.51413
Klabunde, R. E. (2018, 5 Jan 2018). Nitric Oxide. Retrieved from https://www.cvphysiology.com/Blood%20Flow/BF011
Kojima, H., Nakatsubo, N., Kikuchi, K., Kawahara, S., Kirino, Y., Nagoshi, H., . . . Nagano, T. (1998). Detection and imaging of nitric oxide with novel fluorescent indicators: diaminofluoresceins. Analytical Chemistry, 70(13), 2446-2453. doi:10.1021/ac9801723
Krüger-Genge, A., Blocki, A., Franke, R. P., & Jung, F. (2019). Vascular Endothelial Cell Biology: An Update. International Journal of Molecular Sciences, 20(18). doi:10.3390/ijms20184411
Langer, R., & Vacanti, J. P. (1993). Tissue engineering. Science, 260(5110), 920-926. doi:10.1126/science.8493529
Nemeno-Guanzon, J. G., Lee, S., Berg, J. R., Jo, Y. H., Yeo, J. E., Nam, B. M., . . . Lee, J. I. (2012). Trends in tissue engineering for blood vessels. J Biomed Biotechnol, 2012, 956345. doi:10.1155/2012/956345
Peck, M., Gebhart, D., Dusserre, N., McAllister, T. N., & L’Heureux, N. (2012). The evolution of vascular tissue engineering and current state of the art. Cells Tissues Organs, 195(1-2), 144-158. doi:10.1159/000331406
Rensen, S. S., Doevendans, P. A., & van Eys, G. J. (2007). Regulation and characteristics of vascular smooth muscle cell phenotypic diversity. Netherlands Heart Journal, 15(3), 100-108. doi:10.1007/bf03085963
Sandoo, A., van Zanten, J. J., Metsios, G. S., Carroll, D., & Kitas, G. D. (2010). The endothelium and its role in regulating vascular tone. Open Cardiovascular Medicine Journal, 4, 302-312. doi:10.2174/1874192401004010302
Schutte, S., & Nerem, R. (2013). Blood Vessel Tissue Engineering. In (pp. 1237-1246).
Song, H. G., Rumma, R. T., Ozaki, C. K., Edelman, E. R., & Chen, C. S. (2018). Vascular Tissue Engineering: Progress, Challenges, and Clinical Promise. Cell Stem Cell, 22(3), 340-354. doi:10.1016/j.stem.2018.02.009
Uhlenhut, K., & Högger, P. (2012). Pitfalls and limitations in using 4,5-diaminofluorescein for evaluating the influence of polyphenols on nitric oxide release from endothelial cells. Free Radical Biology and Medicine, 52(11-12), 2266-2275. doi:10.1016/j.freeradbiomed.2012.03.006
Wachsmann-Hogiu, S. (2020). Design of Biosensors (in-class lecture). In: McGill University.
Wong, A. P., Nili, N., & Strauss, B. H. (2005). In vitro differences between venous and arterial-derived smooth muscle cells: potential modulatory role of decorin. Cardiovascular Research, 65(3), 702-710. doi:10.1016/j.cardiores.2004.10.012
Yale, H. (n.d.). Blood Vessels Lab. In. medcell.med.yale.edu: Yale University. Retrieved from http://medcell.med.yale.edu/histology/blood_vessels_lab.php
Zeng, W., Li, Y., Wang, Y., & Cao, Y. (2019). Tissue Engineering of Blood Vessels.
Zhang, X., Kim, W. S., Hatcher, N., Potgieter, K., Moroz, L. L., Gillette, R., & Sweedler, J. V. (2002). Interfering with nitric oxide measurements. 4,5-diaminofluorescein reacts with dehydroascorbic acid and ascorbic acid. Journal of Biological Chemistry, 277(50), 48472-48478. doi:10.1074/jbc.M209130200