Abstract
The survival of animals within the animal kingdom is heavily contingent on their ability to hunt and eat, which is directly related to the functionalities of their jaws, thus demonstrating this structure’s vitality. Jaws can be compared to a swiss army knife in the sense that they are used for a multitude of functions important to an animal’s survival, such as hunting, eating, producing sounds, or even climbing trees. Jaws have evolved to be complex and efficient as there is no mercy in the animal kingdom. Understanding the complexity of such a polyvalent tool is quite a difficult task. In the past, a lever model acted as the fundamental system explaining the functionalities of the jaw; however, this reductionist model can not explain the versatility of this amazing system. In fact, closed kinematic chains need to be considered in order to properly explain the jaw’s complexity as a cohesive unit. In this essay, fish and invertebrates are analyzed more in-depth to understand their specificities. Lacking hands, jaws are the only tool that fish can use to interact with their environment. In their highly kinetic skulls, complex mechanisms such as suction feeding and protrusion are used to catch prey, while the food is simultaneously processed in a second set of jaws. In the case of invertebrates, these species have acquired one of the fastest and most complex systems known to date within their jaw. Using the concepts of latches and springs, hunting and defence mechanisms occur with a greater speed and intensity than muscles are capable of alone, allowing for maximized power outputs. The more complex models developed in this paper solve the jaw modelisation conundrum by allowing the representation of jaw mechanisms to have a higher amplitude and a greater precision.
Introduction
Throughout history, species have evolved in order to be more adapted to their respective environments. Feeding is a key behaviour in all species, so having a better feeding performance is an important asset for any animal’s survival. In order to achieve that, more complex and specific behaviours appeared in all clades to have a better catch and processing of their food, depending on their environment (water, land) and alimentation (prey size, hardness…). The manner of catching and processing the food differs in each species due to the simple fact that they live in different environments and have different eating habits. For example, some species might need a faster prey catch, while a strong bite is more important in others. These behaviours could not be accomplished with a simple jaw represented by two bars connected by a joint, as we can see in children’s toys. Jaws are, in fact, very complex structures in which many components, mostly skeletal elements and muscles but not solely, are involved in forming multiple closed kinematic chains representing the different mechanisms found in each species.
Biological systems and mechanisms are usually represented by scientific models to provide a better understanding of the natural world. Models use familiar objects to represent more complex structures, making it easier to visualize and understand processes and then make predictions. Modelling also has its limitations. Simple models do not always include all details and are usually based on approximations, leading to a lack of accuracy. In relation to the jaw, the complexity of the jaw must be represented by a sufficiently complex theoretical model in which most components and mechanisms are taken into account. Using a reductionist model with biological elements could lead to a miscomprehension and diminishment of the complexities of structures. Indeed, as George Box said, “All models are wrong, but some are useful” (George Box, 1976). Understanding the full complexities of biological mechanisms allows for improved developments in the medical field or simply to better understand an animal’s structure and function relationships without diminishing the intricacy of its biology (Van Regenmortel, 2004).
Organisms are biologically complex and are composed of multiple interacting parts. The more the operation of a system depends on each of its parts, the more integrated these parts tend to be. Integration thus becomes an emergent property of complexity, which is evident in the topic of this essay, the jaw. When looking at each component making up the jaw alone, it is rather simple to make this mistake of developing reductionist models, as done with the lever model, however, considering the totality of integration within the jaw, more complex systems can be discovered (Kane & Higham, 2015).
The Lever System: An Old-Fashioned Mainstream Theory
Overview of the lever system
First recognized by Borelli (1608-1679) over three hundred years ago, the lever system model is the product of analysis and research on the locomotor apparatus in man but also birds, fishes, etc. Borelli’s work relied on observations, critical thinking, and probing experiments of the time. He states that the muscles of a creature work with short lever arms to balance the body weight that works with a longer lever arm. This ensures that the joints transmit forces equal to or greater than the weight of that same body (Maquet, 1992).
In biomechanics, lever systems have been compared to synovial joints found in the body. The reason why scientists in iatrophysics (physics applied to medicine) relied on that system is because lever systems and synovial joints have three parts of their functioning in common: a force, a fulcrum, and a load (Parent, Desiree 2020).
Jaws, in particular, according to the lever system theory, could be modeled by third-class levers (for most mammalian jaws) where the resultant muscle force is between the jaw joint and the teeth (the fulcrum and the output force) (Cassini & Vizcaíno, 2012). Thus, only the masticatory muscles are considered to provide the input force, and the output force is thought to be produced by the teeth on food. The temporomandibular joint (TMJ) corresponds to the pivot in this system (the fulcrum). The forces and moments in this system are shown in Fig. 1 below. The input moment arm (Mi) and output moment arm (Mo) are the segments perpendicular to the action line of the forces and the pivot point.
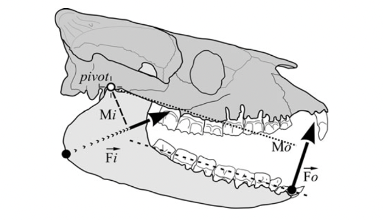
Fig. 1 : Jaws shown as acting like a third-class lever system in a Diadiaphorus skull. Fi is the input force vector, Fo is the output force vector (Cassini & Vizcaíno, 2012).
Given this representation, the following equation is satisfied when the system is in equilibrium:

To be brief, this functioning involves the contraction of the masseter and the pulling of the mandibular angle, which results in a closing force (Cassini & Vizcaíno, 2012).
Following this lever system theory of Borelli, Turnbull (1970) even points out that some mammalian masticatory systems in many rodents convert the system into a second-class lever with a resultant force anterior to the distal cheek teeth. This is due to the size and position of the masseter in these animals (Cox, Philip G., et al., 2017).
Limits of Lever System
In the lever system, the temporomandibular joint is represented as the pivot point of the mechanism. This joint is a complex and essential mechanical system in various mammals since it contributes to everything related to mastication and speech, such as biting, chewing, swallowing, etc. In Fig. 2 A below, a human jaw is shown to illustrate the mechanism, which is closely analogous in most mammals or other animals. In general, the mandibular condyle (major moving structure in the TMJ situated on the temporal bone), along with the compression of the TMJ disc, facilitates the articulation and mouth opening by rotating and sliding forward, with help from complex muscle moments. However, it is not as simple as explained by this model.
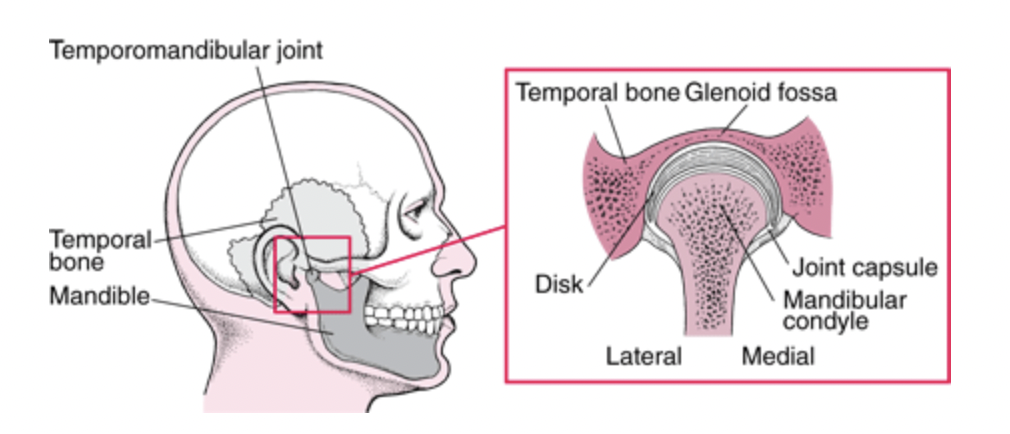
Fig. 2 : (A) The temporomandibular joint (Klasser & Gary D, 2022)
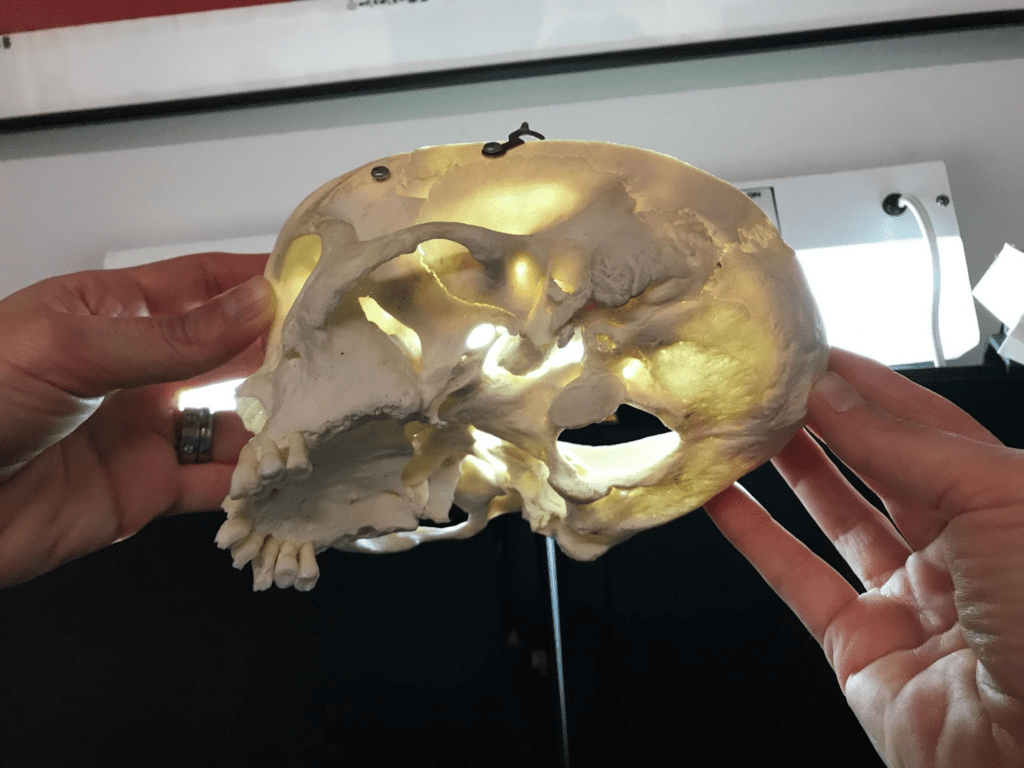
Fig. 2: (B) picture of a human skull (courtesy of Dr. Reznikov, personal collection) – the arrow points at the glenoid fossa viewed against a source of light.
In fact, the mechanics behind the TMJ are still controversial even if it is generally accepted that it is “loaded under compression,” like a third-class lever. The controversy exists partially because of the difficulty of gaining information on this joint, and because of the changes that these articular structures suffer after multiple stresses. There are also debates on the contribution of other bones, muscles, and ligaments on this joint’s mobility/stability (Scarr & Harrison, 2017). However, the glenoid fossa of a human temporal bone is paper-thin and transparent in a dry skull, as seen in Fig. 2 B. A fulcrum must be robust; however, in this theory, we consider the fulcrum as a fragile element. There is an inconsistency between this old theory and anatomic facts.
As mentioned before, the already established lever model has remained unchanged since its conception. It compares “man-made” machines to animal bodies and mechanisms. Levers generate stress concentrations that engineered designs are adapted to, whereas the same stresses would greatly damage and tire developing tissues. In addition, living structures do not move around fixed fulcrums. The reliability of this analogy is questionable since it considers that joint surfaces must be compressed, whereas that is not how all joints work (Levin et al., 2017). The lever system, being analogical to a machine, does not follow the tendency of biological systems to adapt and change over the course of evolution (Scarr & Harrison, 2017).
Biotensegrity systems are, for example, another type of system that analyzes living organisms with a complex balance of force vectors (tension or compression) that are interconnected but maintain a stable equilibrium. In a biotensegrity model, each structure acts within a larger interlinked structural entity (Levin et al., 2017). It considers that each part of the body influences another, instead of isolating the jaw completely from the rest of the body, as the lever system does. The TMJ is therefore considered a system with multiple systems within it, allowing the mobility of the mandible. The mechanics of biotensegrity are described by closed-chain kinematic geometry, which gives researchers the chance to study and reveal aspects of the TMJ that were otherwise obscured by reductionist methods such as the lever theory, which is less inclusive of the multi-disciplinary factors involved in the mobility of the jaw (Scarr & Harrison, 2017).
Even though lever models have dominated biomechanical research with their kinematic analysis, they ignore the geometric organization of complex biological evolutionary systems. To better understand biological motion, including the motion of the jaw, closed kinematic chain systems have been used. Indeed, like the biotensegrity systems (a type of CKC themselves), the closed kinematic chains consider each part of the mechanism; it also responds to constantly changing conditions and acts in synergy with the nervous system. Viewing the jaw as a network of CKCs helps us understand the contribution of other body parts to the movement of the jaw. The jaw system cannot be isolated since it is connected to other mechanisms, tissues, muscles, etc (Levin et al., 2017).
Fish Jaw: More Than Just a Lever System
Skull mechanisms in fish were always a great subject of research throughout history due to their variety of movements, particularly the movement of the jaw. To name a few mobile elements of the jaw, there is the upper jaw which has a role in pushing the premaxilla forward into a protruded position, the lower jaw, and the mandible, which is powered by the adductor mandibulae muscles and other elements shown in Fig. 3 below (Westneat, 2005).
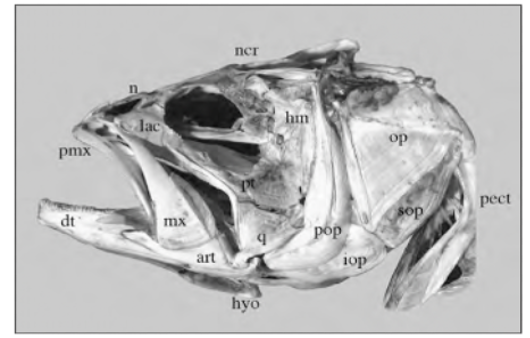
Fig. 3 : Skull morphology of the large-mouth bass, Micropterus salmoides. Pmx, premaxilla, hyo, hyoid, mx, maxilla (Westneat, 2005).
The studies on this structure of fish focus not only on kinematics but also on modelling cranial function and muscle contraction patterns. The feeding of fish includes suction, the advent of jaw protrusion and many kinetic features of the skull, which is why, if we represent the procedure with only a lever system, it will reduce this whole complex mechanism to only an opening/closing of the mouth, which is inaccurate. We can see in Fig. 4 the many interactions and couplings between the different parts of the skull responsible for feeding in fish. Mechanisms such as the mouth opening and protrusion (explained further on) are connected to the different jaw components such as the premaxilla, maxilla and mandible. Suction is also one mechanism that creates multiple interactions between parts of the skull, as shown in Fig. 4.
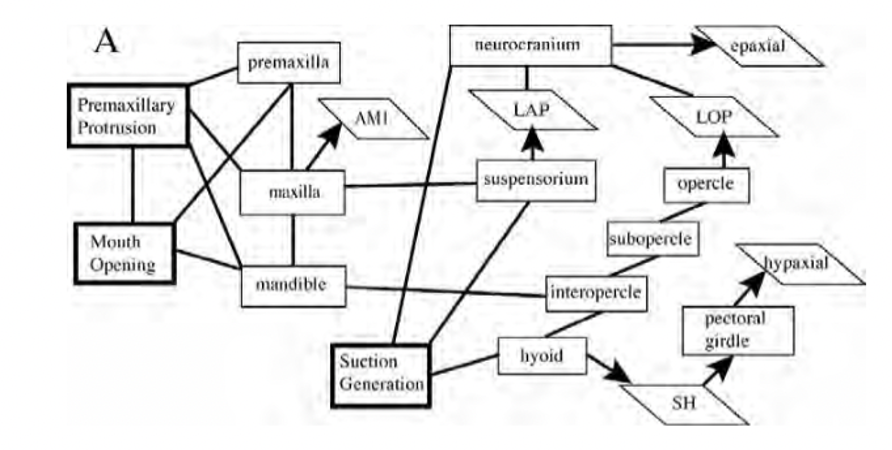
Fig. 4 : Mechanical couplings and interactions of cranial bones and muscles during feeding in teleost fishes (Westneat, 2005).
Although the lifting of the skull (which is a feature of fish feeding) has been seen as a relatively simple third-class lever system (biomechanics of the mandible) that contributes to mouth opening, the more important contributor to the increase of the gap between the upper and lower jaw is the rotation of the lower jaw. This action and the forces needed for it are very complex and are modelled by multiple couplings/linkages that transmit forces. There are three possible jaw opening mechanisms in fish, which include a mechanism modelled as a four-bar linkage mechanism – therefore, a CKC (Westneat, 1990) that will be developed below. The jaw functioning does not only include the jaw opening but also an upper jaw protrusion that will be explained later in further detail . The closing of the jaw mechanism includes the adductor mandibulae muscles, cranial elevation, and retraction of the premaxilla in order to bring the upper jaw and lower jaw into a closed gap position (Westneat, 2005).
To simplify matters and apply biomechanical calculations, this mechanism has been reduced to a lever system. However, later studies include the geometry, muscle morphology, and exterior factors such as the effect of water studied by Van Wassenbert et al. (2005). The interaction system between mobile elements in the skull/jaw of fish has been analyzed with linkage theory from mechanical engineering, like the four-bar linkage mechanism by Anker (1974) (Westneat, 2005).
Degrees of Freedom, Another Limitation of Lever System Models
A mechanical system in space has several degrees of freedom, “DoF”, that correspond to the number of independent variables which define the system (Sheldon, Robert. 2022). We can use the same example of fish skull/jaw to illustrate the significance of DoFs in jaw modeling. Fish have a skull that performs very complex manipulations with over 20 movable skeletal elements including the mandibular or the pharyngeal jaw. They have behaviors that are in direct contact between the jaw/skeletal elements and the food. All of the fish behaviors are related in one way or another to a control of the flow of water where the food particles are suspended (Olsen et al., 2020).
In order to control the position and orientation of an object this way, it is necessary to control all the DoFs of the object. Internal mobility and task mobility are strongly related. Thus, a system needs to have as many, or more DoFs than the ones required for the task performed. According to Olsen (Olsen et al., 2020), fish skulls require a minimum of 3 DoFs to control 3D translation (along the three axes) or 6 DoFs if rotation is considered too. Lever systems only rotate and translate about one axis (planar), which gives them only 2 DoFs. That isn’t enough to accurately represent the intricacy of the real skull composition/mechanism.
The results of the research presented in Olsen et al.’s article (Olsen et al.,. 2020) conclude that fish skulls have 7 DoFs that are needed during feeding, which is also confirmed by the different motion patterns that they observed. Therefore, because of the high interconnectivity in the catfish skull, which can be generalized to most fish skulls as claimed in the study, fish skulls have a potentially high DoF system (Olsen et al., 2020). Hydrodynamic models can be used to solve the issue of limiting DoFs in lever systems. This concept will be explained in more depth later on.
Closed Kinematic Chains: A New and Improved Theory
The Omnipresence of Kinematic Chains
Before we define a closed kinematic chain (CKC), we need to understand what a kinematic chain is. A kinematic chain from both the mechanical standpoint and biological one can be defined as a combination of several joints linking several rigid segments or links together, during a specific movement (Kinematic Chain, 2012).
In traditional mechanical engineering, a kinematic chain is a group of links either joined together or arranged in a manner that permits them to move relative to one another. The chain is said to be closed if the two ends of the series are fixed (constrained). A functional consequence of a closed chain is that the movement of one joint will cause every other joint to move in a predictable fashion. However, if we un-fix just one of the two ends of the group of links, the chain is considered to be open and in such a case, the chain is no longer restricted to moving in a prescribed fashion. In such a case, each joint can move without necessarily impacting another joint to also move. An open kinematic chain, such as a 3-bar kinematic chain, can be considered a “chaotic pendulum” that serves no use without a closed connection. An open two-bar kinematic chain can be interpreted as a lever system. Fig. 5 shows the difference between a closed kinematic chain (A) and an open one (B). (Schoenfeld B. Snarr R. L, 2022)
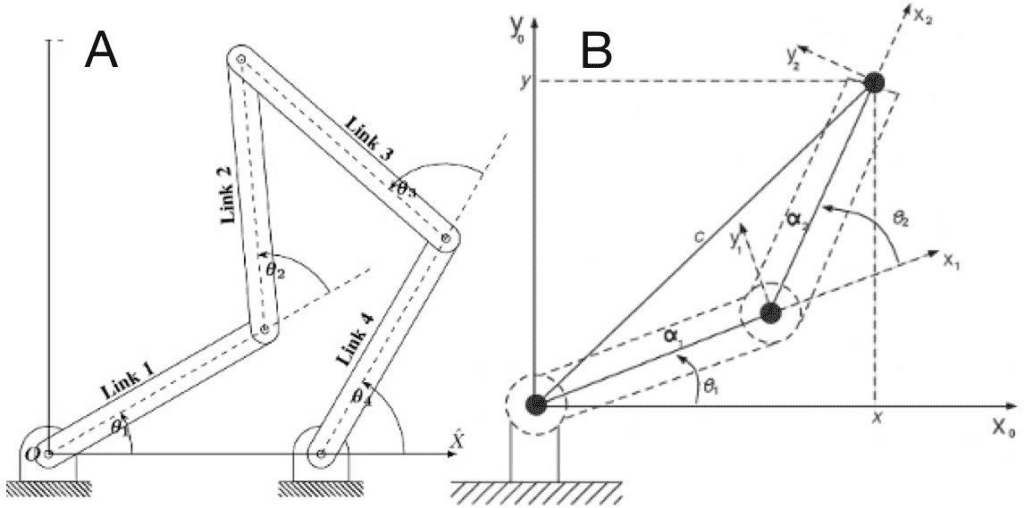
Fig. 5 : Closed (A) vs open (B) kinematic chains (Balaji, 2017) (Farooq et al., 2013)
It is also important to note that people often confound kinetic chains with kinematic chains. Kinematics is the branch of science that studies the motion of systems without considering the forces which cause the motion, used mainly in mechanical engineering. On the other hand, kinetics focuses foremostly on the forces as well as their weight and inertia effects and is used when describing joints and linkages as they relate to the human body (Schoenfeld B. Snarr R. L, 2022). This paper will focus on kinematic chains and, more specifically, how closed kinematic chains are prevalent in the functioning of the jaw of certain species.
Application in Mechanics
CKCs have several applications in the design of mechanical systems. One can easily find them in systems such as heavy excavation machinery, piston assemblies and automobile steering wheels. The most common CKC is the 4-bar chain, also known as the Quadratic Cycle Chain. The 4-bar chain is composed of 4 links (bars) of different lengths, each connected by a joint and each linkage (link-joint-link set) makes a turning pair. As one linkage completes a revolution, it transmits a corresponding oscillating motion to the other linkage. Fig. 6 below offers a few variations of this kinematic mechanism.
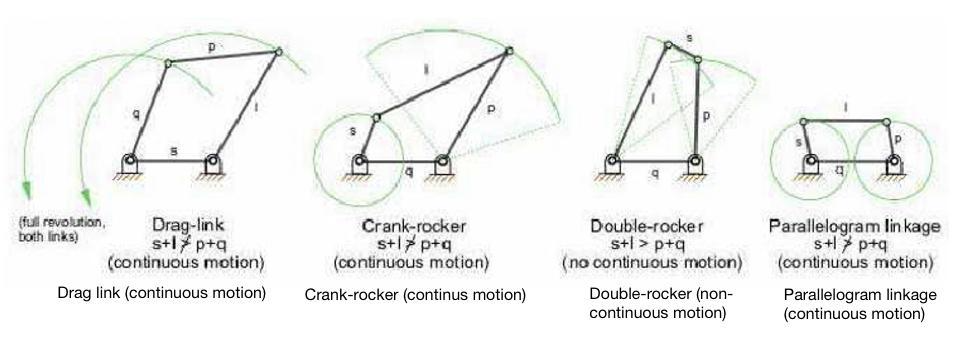
Fig. 6 : Four-bar mechanism classification. Three four-bar mechanisms can produce partial straight-line motion. They are characterized by two joints connected to the fixed base (Rolland, 2022).
CKCs have been present since the Industrial Revolution. They are a simple yet very efficient and effective mechanism which can be found in many applications. All parts work in tandem to influence one another’s behavior and with controlled force and speed (i.e. kinetic energy) as its final resultant. Sometimes referred to as a “complex lever system”, this CKC can also be found in biological systems, but in a much more sophisticated formation.
For over 300 years, the lever system, which is a simple two-bar open kinematic chain, was the most acceptable biomechanical model. Most biological bodies were studied and regarded as open kinematic chains, where the supporting joints were modeled as levers composing a body that is structured as an inverted pendulum. The model in Fig. 7 below demonstrates how each joint must be stabilized by local forces, seeing as there is no closed chain connecting element. Lever systems are now less regarded as the most accepted biomechanical models when considering the effects of OKCs in the body. The forces generated by these OKCs put a lot of pressure on surrounding tissues, and attempting to stabilize more than one joint at a time becomes a lot more difficult as the help of external systems like nerves, muscles and joints are required (Levin, 2013).
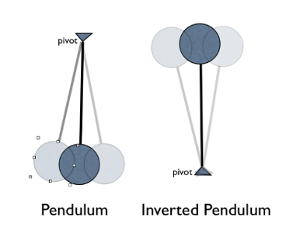
Fig. 7: The body has been modeled as an inverted pendulum and compared to balancing a series of sticks in the palm of one’s hand (Reeves NP, Narendra KS, Cholewicki J: Spine stability: lessons from balancing a stick. Clin Biomech (Bristol, Avon) 2011, 26:325-330.) The inverted double pendulum is unstable (Levin, 2013).
The proposed solution to this biomechanical model is one of a multi-bar CKC, like a four-bar linkage kinematic chain. The movements which stem from CKC action simplify neurological control and play a role in affecting velocities from a motor source. Essentially stipulating that small movements in a muscle separated by distance and joints from the output area can produce large movements and high velocities at the terminal of a closed kinematic chain. This concept drives the main intrigue for closed kinematic chains in the jaws of animals as powerful core body muscles may add force to extremital structures that have smaller and weaker muscles (Levin, 2013).
For a long time, scientists have classified anatomical functions and structures based on traditional theories. It is only since the work done by Vesalius in (1514-1564) that many theories were debunked, bringing forward new evidence of muscolo-skeletal dualities that explain how motion is achieved through CKCs. The 4-bar planar CKC, explained earlier in mechanical systems, is the simplest configuration that enables the anatomical structure that exists naturally in the jaws of species to control motion through its mechanical properties. The 3-bar (rigid) systems and 5-bar (less controllable) systems can also be found in certain species; however, the 4-bar planar remains the most appreciated (Levin et al., 2017). Now, one can analyze the use of closed kinematic chains in the feeding mechanisms amongst various species and their importance of application, starting with fish and eels.
Closed Kinematic Chains in Fish & Eels
The most popular 4-bar CKC has been that of the feeding system (jaws) of fish. This mechanism is composed of the jaw, neurocranium, hyoid, suspensoria (cheeks) and opercula (gill covers), which all come together as multiple and coupled CKCs in different planes that change shape and enable the consumption of their prey. The structure, on its own, can respond rapidly to changing conditions of the prey and the ingestion process, almost self-regulating in a way beyond the capabilities of the nervous system. A series of interlinked tissues can create mechanical behavior that results in a geometry of motion. Devoid of muscular attachment, these structures move with incredible force and speed and can capture prey within 50 milliseconds, in a large-mouth bass (Fig.3), for example (Levin et al., 2017).
A single 4-bar CKC can also be found in parrotfish. This species lives on the coral reef. The parrotfish must feed from the polyps that are found within the very hard coral. Hence, the fish needs to possess an extremely strong bite, and this can be seen when looking at the other parts of its jaw. The teeth of parrotfish are capable of withstanding very high pressure, which is crucial because of their bite force. One square inch of parrotfish teeth can tolerate 530 tons of pressure which is equivalent to the weight of about 88 elephants. Without having teeth this hard, exerting a strong bite force would not be possible (Mutiara et al., 2021). The powerful bite force is a result of the 4-bar linkage structure of its jaw, as shown in Fig. 8 below, which also shows the forces at work in the open and closed positions. In reality, the parrotfish has 2 sets of jaws. The outer one, which can be seen, and a hidden one, called the pharyngeal jaw, which is located at the back of its throat in the pharynx. The outer jaw bites off the coral, and the pharyngeal jaw crushes it further to reveal its nutrient (Muller, 1996).
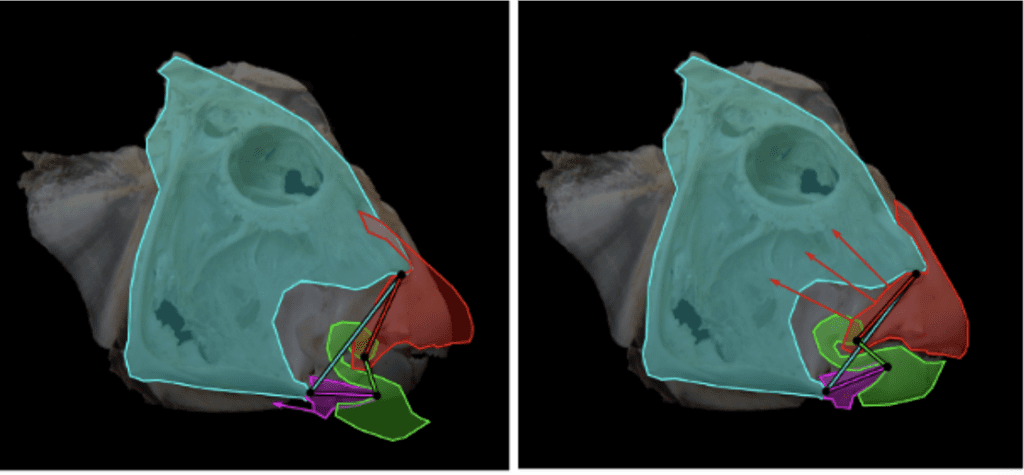
Fig. 8 : Single 4-bar CKC in parrotfish; acting forces in open and closed position (Zuber, 2012)
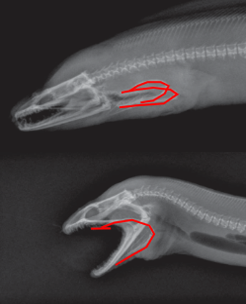
Fig. 9 : Moray eel; pharyngeal jaw (Mehta & Wainwright, 2007)
The Moray eel, shown in Fig. 9, also feeds in the same manner as the parrotfish, whereby it launches its inner (pharyngeal) jaw up into its mouth to capture prey and drag it back into the throat. This phenomenon also became the focal point of the fictional creatures in the movie “Alien” (Mehta & Wainwright, 2007).
The Upper Beak Rotation Mechanism of Birds: A Permanent CKC with Constant Mobility
As previously mentioned, closed kinematic chains allow for several different ways in which a mechanical system can change conformation. They enable higher degrees of freedom which helps explain the intricate mechanisms and functioning of parts of the skull like the feeding apparatus. A great example to investigate the effect of DoF’s in CKCs is with the beak of birds, which is a permanent CKC with constant mobility. A permanent CKC is one in which the number of loops involved in the chain remains the same. Having constant mobility assesses the biological capability of constituent joint mobilities and whether they are constant or variable. In the case of the skull of birds (Fig. 10), the bones of the palate (jugal, palatine, pterygoid and quadrate) form four- and five-bar parallel linkages that elevate and depress the upper beak. When bones and muscles are linked into 4 or 5-bar closed kinematic chains, the multiple bars making up this chain are stabilized by slow-twitch muscles, and thus the desired stability can be achieved a lot more easily. When the different stabilizing muscles are alternated, different portions of a multi-bar kinematic chain can become anchored and/or exert force. This is what can be seen with the upper beak rotation mechanism of birds where the consequential action of multiple CKCs working in tandem creating different ways of motion permits the quadrate to rotate forward and backward with upper beak rotation (Fig. 10E) and adduct–abduct independently of upper beak rotation (Fig. 10F). Moreover, as seen in (Fig. 10A), birds with greater linkage mobility are capable of rotating their lower beaks about a dorsoventral axis (yaw), as the quadrates suspend the lower beak and can control spreading between the left and right sides of the lower beak (wish boning) (Olsen, 2019). An interesting example can be drawn from granivorous finches, who have beaks that are wide and deep, optimized for crushing seeds (Soobramoney & Perrin, 2007). They are capable of feeding this way due to the closed kinematic chains, which allow for this rotational and translational movement of the upper and lower beak. Within these beaks, the formation of a lever system to crush seeds would not be possible as such a system would require an excessive amount of space to generate enough force to crush the seeds. Instead, CKCs allow these carnivorous finches to crush seeds effectively. A study showed that the bite force of granivorous finches can range from 2.9 N to 38.4 N, which is less than a full functioning lever system, which can generate 40 N of force when given enough space to operate (9 cm input force to the fulcrum and 4.5 cm output force to the fulcrum). Regardless, this is still very impressive, and the multifunctionality that closed kinematic chains offers to granivorous finches must not go without notice (van der Meij and Bout, 2006).
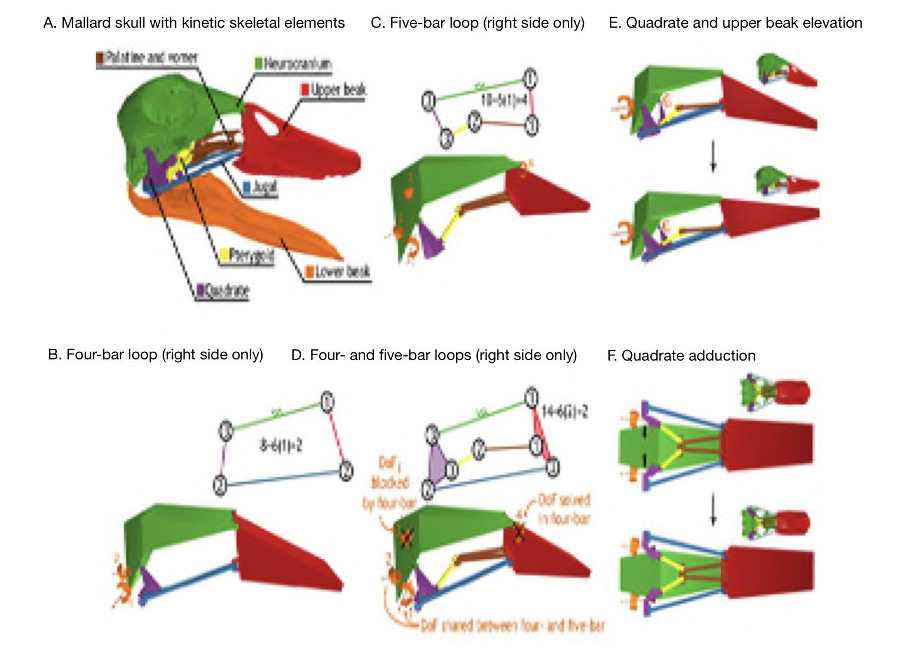
Fig. 10 : The kinetic skeletal elements of the bird skull form a permanent closed chain with permanent mobility. The lower beak is shown only for reference. The skeletal elements of the bird jaw apparatus (A) form a two-DoFs four-bar linkage (B) and a four-DoFs five-bar linkage (C) on each side of the skull (orange arrows indicate DoF). The four- and five-bar linkages connect to form a multi-loop linkage with two DoFs (D) that couples rostral rotation of the quadrates with upper beak elevation (E) and permits quadrate adduction–abduction independent of upper rotation (F) (Olsen, 2019).
From an engineering standpoint, depending on the number of links in a closed kinematic chain, the more links, the less stability there is. However, because the links are connected to each other selectively and stabilized by muscles, if a muscle that is holding two adjacent links contracts, then it can effectively change the type of chain, for example a 5-link chain becoming a 4-link chain. By making the closed kinematic chain static, by which there is no movement, the performance of this kinematic chain is optimized through the contraction of the muscles that attach the neighboring links of the chain.
Closed Kinematic Chains in Mantis Shrimp
CKCs are crucial in some crustacean feeding mechanisms. For example, the striking claw of the mantis shrimp uses a CKC mechanism with an impressively powerful force of 1.5 kN and the speed of a bullet. Elaborating more on this, the ladder can be seen in Fig.11, the diagram below.
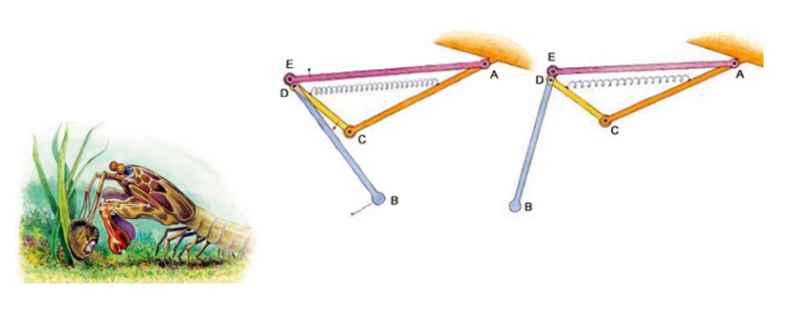
Fig. 11 : Mantis shrimp striking and feeding mechanism (Levin, 2013)
The mechanism that the mantis shrimp uses for striking and feeding is made up of 3 bars of fixed length: bar AC which is stationary, a muscle AE that acts as fixed length bar and a spring that in reality lies above the muscle AE. When cocked, the spring is compressed, and after release, the spring pushes against the bar CD, which makes D turn counterclockwise. The movements of points D and E locked onto bar BE create a strong and fast propulsion force at the extremity, point B, a deadly strike for its prey (Claverie et al., 2011).
In this 4-bar chain containing a lever system, once the spring is contracted, there is a huge amplification of the amplitude of the raptorial appendage (point B). The portion DE is very tiny, whereas the segment DB is very long, so the resultant swing is very powerful. The absolute displacement can be very tiny, but the swing of the raptorial appendage is affected with huge amplitude, and the speed increases so dramatically that it causes water cavitation. Each strike can generate two brief, high-amplitude force peaks, typically 390-480 μs apart and peak limb impact forces range from 400 to 1501 N, and peak cavitation forces reach 504 N (Patek & Caldwell, 2005).
Closed Kinematic Chains in Insects
The jaws of some insects are referred to as mandibles. Ants use their mandibles for a variety of functions, and as such, they have evolved into a variety of shapes and configurations. Like the fish species, ants lack limbs (forelegs) with which to grasp things, and, as such, they rely on their mandibles to manipulate food particles, even liquid. In addition, ants also use their mandibles to process their food. The carnivorous species of ants use them to decapitate or dismember their prey. Mandibles are generally used to puncture or grind their food. But where does their 4-bar mechanism come into play? It is said that, in some species of ants, the power-amplified trap-jaw mandibles have evolved 4 times. This type of mandible is based on elongated mandibles locked open with a latch mechanism (Fig. 12, A). The muscles stretch the jaws apart so that there is a 180o angle between them. The consequence of this action is the deformation of the sides of the ant’s head, making it slightly shorter (3.2 percent) and narrower (6 percent) in the middle. As the exoskeleton deforms inwards, it stores elastic potential energy that can be used when the jaws are released (Sutton et al., 2022). and when mechanosensory trigger hairs are stimulated, the latch is released rapidly, resulting in a rapid closure of the mandibles (“strike”). These mandible strikes are said to generate forces that are 300 times the mass of the ant. Fig. 12, B shows the correlation of the ant’s trap-jaw kinematics to that of a latch-mediated spring actuation (LaMSA) mechanism, which is based on the concept of CKCs (Gibson et al., 2018). This is covered in more detail in the next section.
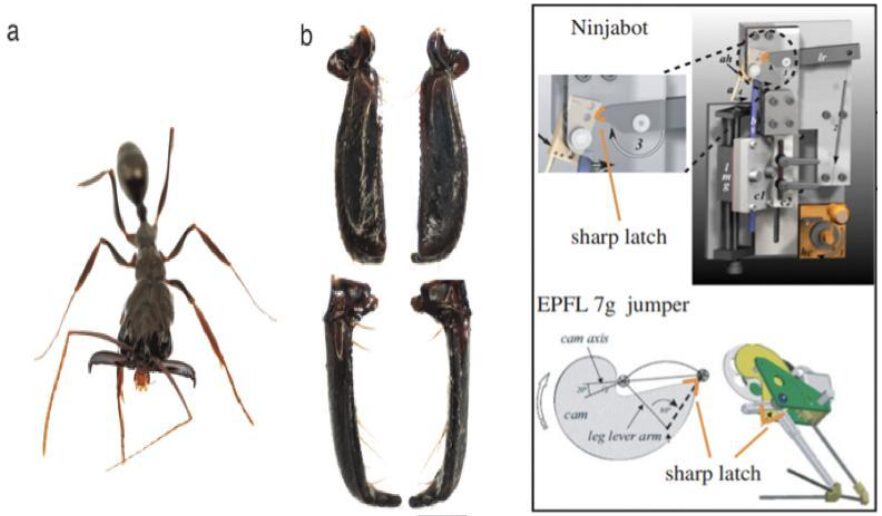
Fig. 12 A : Trap-jaw mandibles in ants (Patek et al., 2006) ; Fig. 12 B : Latch-mediated spring actuation (LaMSA) mechanisms (Divi et al., 2020)
Particularities of Fish Jaw Mechanisms
Fish use their mouth for multiple tasks, including filter feeding, mouthbrooding, suction feeding, and prey processing. These complex behaviors require careful control over the flow of water going in and out of the fish’s mouth, termed “hydraulic transport” (Bemis & Lauder, 1986). This is achieved by their highly kinetic skulls, where 20 movable skeletal elements, including the mandibular and hyoid arches, the branchial apparatus that supports the gills, the shoulder girdle, and in some fishes, the pharyngeal jaws (Olsen et al., 2020).
Suction Feeding
During suction feeding, fish explosively expand their mouth and throat to capture prey (Gibb & Ferry-Graham, 2005). The body muscles supply up to 95% of the power by actuating the cranial linkage through attachments on the neurocranium and shoulder (Camp et al., 2015). This expansion and compression motion could be compared to the mechanism of an umbrella, which has 2 degrees of freedom, or even to a syringe, with only 1 degree of freedom (Camp et al., 2015). However, considering that fish have over 24 smaller cranial muscles active during feeding (Wainwright et al., 2012), those models are oversimplifying the actual mechanism. If the fish skull had these few degrees of freedom, the relative timing and magnitudes of expansion in different parts of the skull could not be varied to generate the different motion patterns associated with its jaw. Taking the example of the channel catfish, a recent study estimated that the mobility of its skull involved 19 potential degrees of freedom, and at least seven of those are involved in the feeding mechanism (Olsen et al., 2020). Moreover, for every degree of freedom, at least two muscles are needed to form an agonist-antagonist pair, meaning that a minimum number of 14 cranial muscles associated with the mandibular arch, hyoid arch, and shoulder are involved in the movement of the jaw creating a multitude of closed kinematic chains (Fig. 13) that couple together and mutually change shape. That way, they enable structures to move with remarkable force and speed permitting rapid expansion of the head and buccal cavity, mouth opening, sucking in and containment of the prey (Levin et al., 2017). They are also very efficient since they use a relatively small number of muscles to initiate complex movements in different planes.
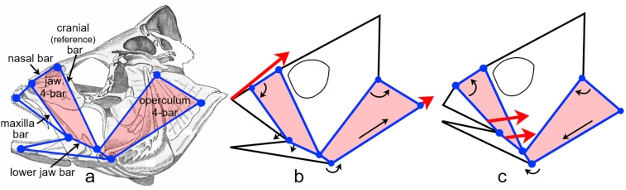
Fig. 13: The coupling of multiple closed kinematic chains. A fish skull (perch) showing two of the many 4-bar mechanisms that facilitate mouth opening and sucking in of the prey; and how muscles (large arrows) change the relative position of individual ‘bars’ (small arrows) and alter the shape of the multiple closed kinematic chains during feeding (Levin et al., 2017).
For many years, closed kinematic chains were considered as acting within a single plane, oversimplifying the analysis. However, it is now known that they do not operate in isolation. The multiple bars that contribute to the motion of the different components of the jaw are structurally and functionally coupled together and operate in different planes using the power of local muscles and more distantly placed swimming muscles (Westneat & Olsen, 2015). In this example (Fig. 13), a particular closed kinematic chain will have multiple functions, such as lower jaw depression, maxillary rotation and nasal elevation, depending on the lengths of the bars that determine its overall shape and mechanical properties (Wainwright et al., 2005).
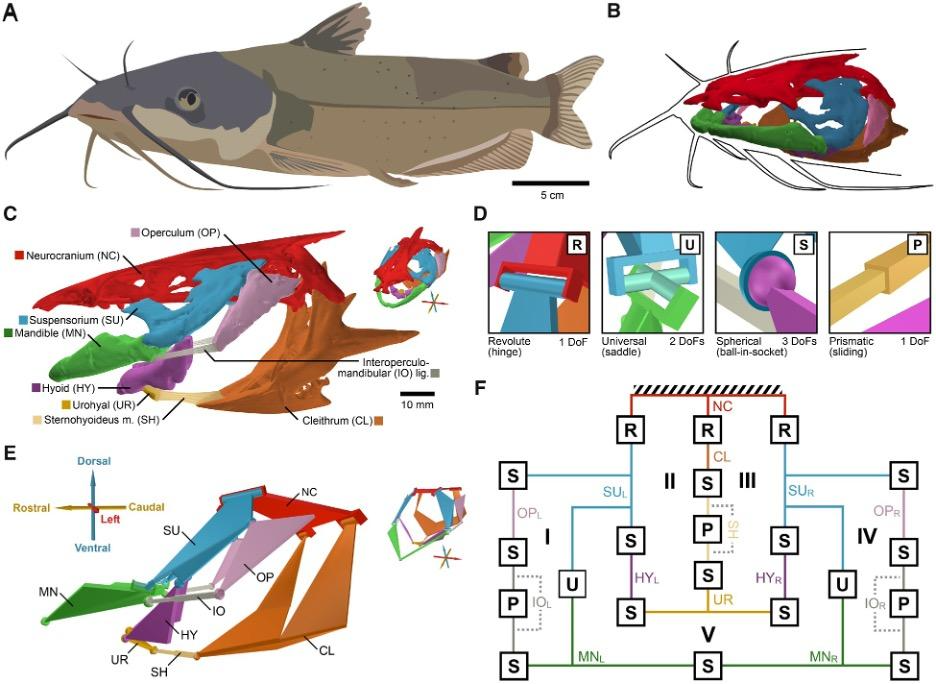
Fig. 14 : Anatomy of the channel catfish (Olsen et al., 2020).
As mentioned previously, the hydrodynamic model was more recently developed to represent fish jaw mechanisms more accurately. It simulates the fluid flow patterns created by the skull based on bidirectional flows generated by fish. These models can be used to solve the issue of limiting DoFs and better represent the complex mobility of fish jaws. This model takes into consideration not only the mechanical linkage but also hydrodynamic motions. In comparison to the planar four-bar linkage model previously analyzed, this model suggests greater mobility. In the case study about channel catfish (Olsen et al., 2020), a new 3D mechanical linkage model (Fig. 14) of a fish skull that incorporates the pectoral girdle and the mandibular and hyoid arches was validated using an in vivo motion dataset of suction feeding in channel catfish in which the degrees of freedom in the fish skull were quantified. That way, it was possible to categorize the motion patterns of the cranial linkage during feeding and to evaluate the association between these patterns and food motion.
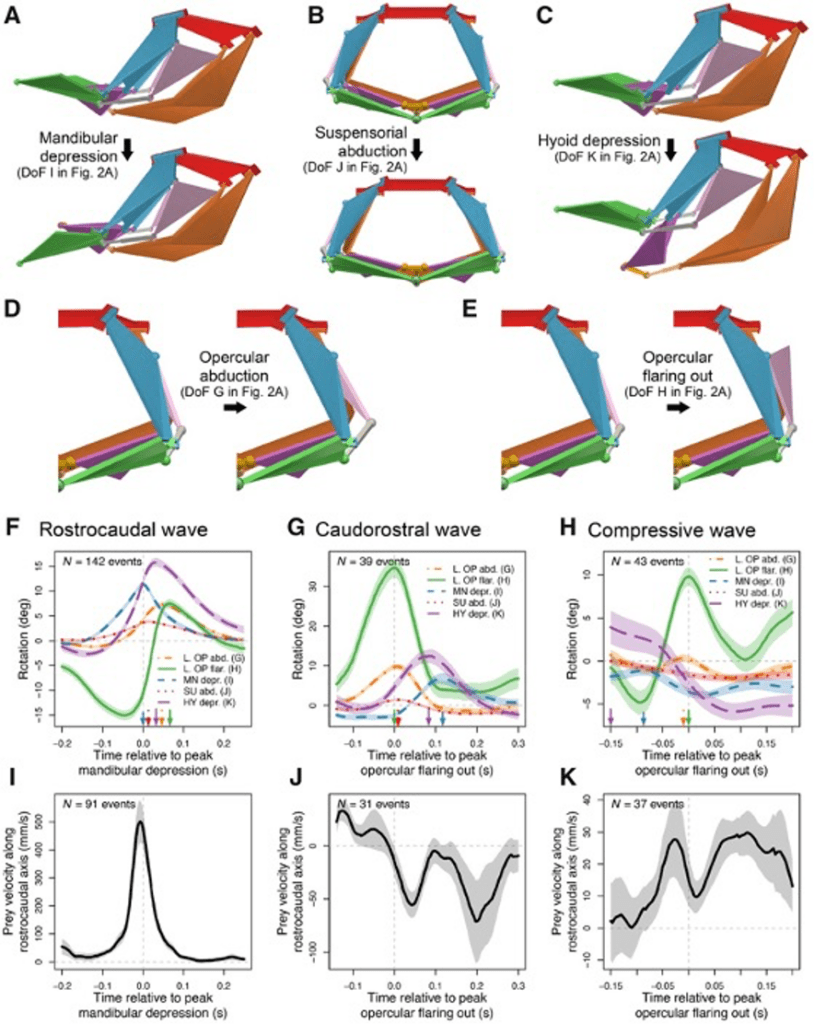
Fig 15 : A multi-DoFs mechanism enables multiple prey manipulation patterns (Olsen et al., 2020).
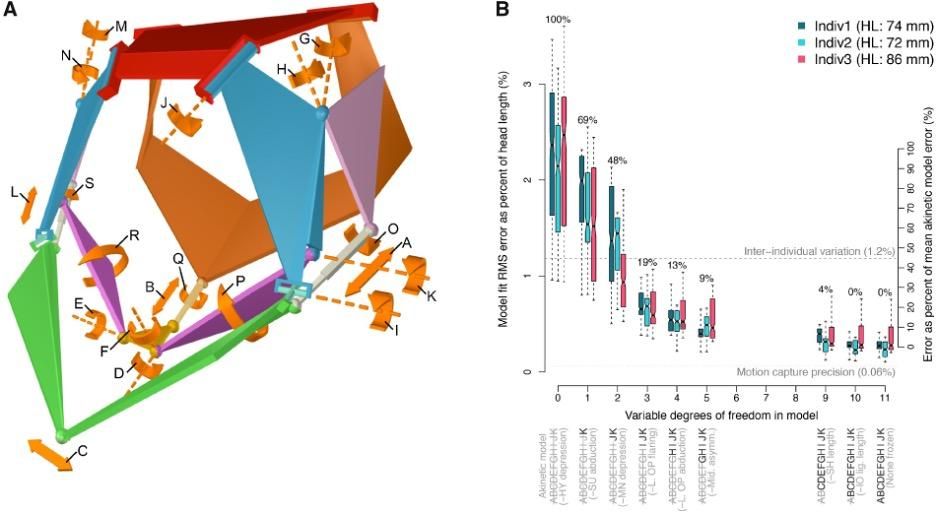
Fig. 16: Mechanism model fit to in vivo motion (Olsen et al., 2020).
Although the channel fish has at least 19 degrees of freedom, indicated by orange arrows in Fig. 16, just seven are sufficient to describe most of the motion during suction feeding, having non-linear relationships. In this species, most of the motion in the channel catfish skull can be described by five independent degrees of freedom (Fig. 17), namely mandibular depression (A), suspensory abduction (B), hyoid depression (C), opercular abduction (D), and opercular flaring out (E). Channel catfish use this specific linkage to generate three motion patterns, namely a rostrocaudal wave (F) associated with a sharp positive peak in prey velocity (I) occurring before prey capture, a caudo-rostral (G) wave associated with moderate negative prey velocities (J), and a compressive wave (H) associated with moderate positive prey velocities (K), both occurring after prey capture. For each motion pattern, a minimum of degrees of freedom is required to effectively control objects in water. (Olsen et al., 2020) Therefore, this model which combines high mobility with flow-based manipulations is a better representation of the fish jaw mechanism, which is far from being simple as it was believed in the past.
Protrusion
Protrusion is a specific mechanism present in some species of fish that enables them to deploy their mouth forward at a high speed to rapidly decrease the distance between themselves and their prey, and ultimately catch food (Fig. 17). This deployable mouth gives a very significant advantage when the prey is in close range.
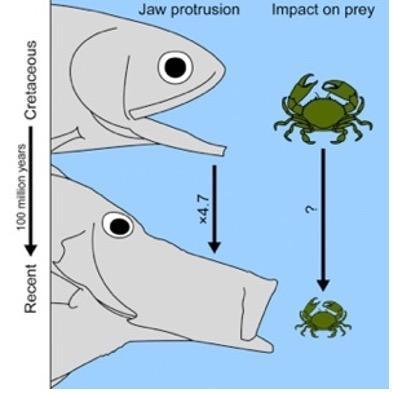
Fig. 17: Graphical representation of protrusion functionality (Bellwood et al., 2015).
Premaxillary protrusion mechanisms have evolved from species to species, each involving very different musculoskeletal mechanics. In all teleosts, regardless of how the jaws are connected, depression of the lower jaw acts to pull the upper jaw into the protruded state. There are four different upper jaw protrusion mechanisms; however, the most widely observed is the “mandible depression model” (Matott et al., 2005). Three separate kinematic pathways allow the lower jaw to move anteriorly, resulting in the passive anterior protrusion of the upper jaws (Fig. 18). In the first one, lower jaw depression at the quadro mandibular joint separates the upper and lower jaws, allowing space for prey, while facilitating the protrusion movement of the jaws (Fig. 14A). A second pathway for protrusion would combine cranial elevation and lower jaw depression (Fig. 14B). Finally, a third kinematic pathway involves anterior rotation within the suspensorium. These points of flexion allow portions of the suspensorium to swing forward independently of the neurocranium. Since the suspensorium suspends the lower jaw, this results in anterior movement of the lower jaw, while the ligamentous connections between both jaws result in synchronous anterior protrusion (Fig. 14C).
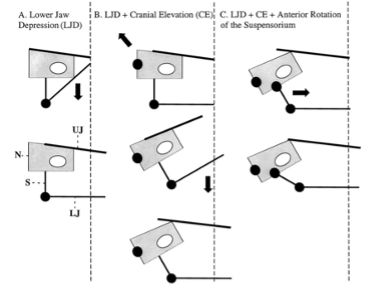
Fig. 18: Three kinematic pathways that cause jaw protrusion. A: Depression of the lower jaw (LJ) B: Both depression of the LJ and cranial elevation at the joint between the vertebral column and the neurocranium (N). C: Depression of the LJ, cranial elevation, and anterior rotation of the suspensorium (S) at joints (Waltzek & Wainwright, 2003)
Jaw protrusion improves feeding performance by enhancing both ram velocity, composed of a locomotor component plus a jaw protrusion component (Lauder & Liem, 1981), as well as suction generation. Suction is generated by rapid buccal expansion and is essential to draw water and prey into the predator’s mouth. The ability to draw prey into the mouth from a further distance and at a greater velocity makes a fish a better suction feeder. In order to evaluate these abilities, a comparative study was carried out by T. Walzek and P. C. Wainwright on the New World cichlids Petenia splendida, Caquetaia spp. and Astronotus ocellatus, all possessing extraordinary protrusible jaws, over a total of 15 feeding events per species. (Waltzek & Wainwright, 2003).
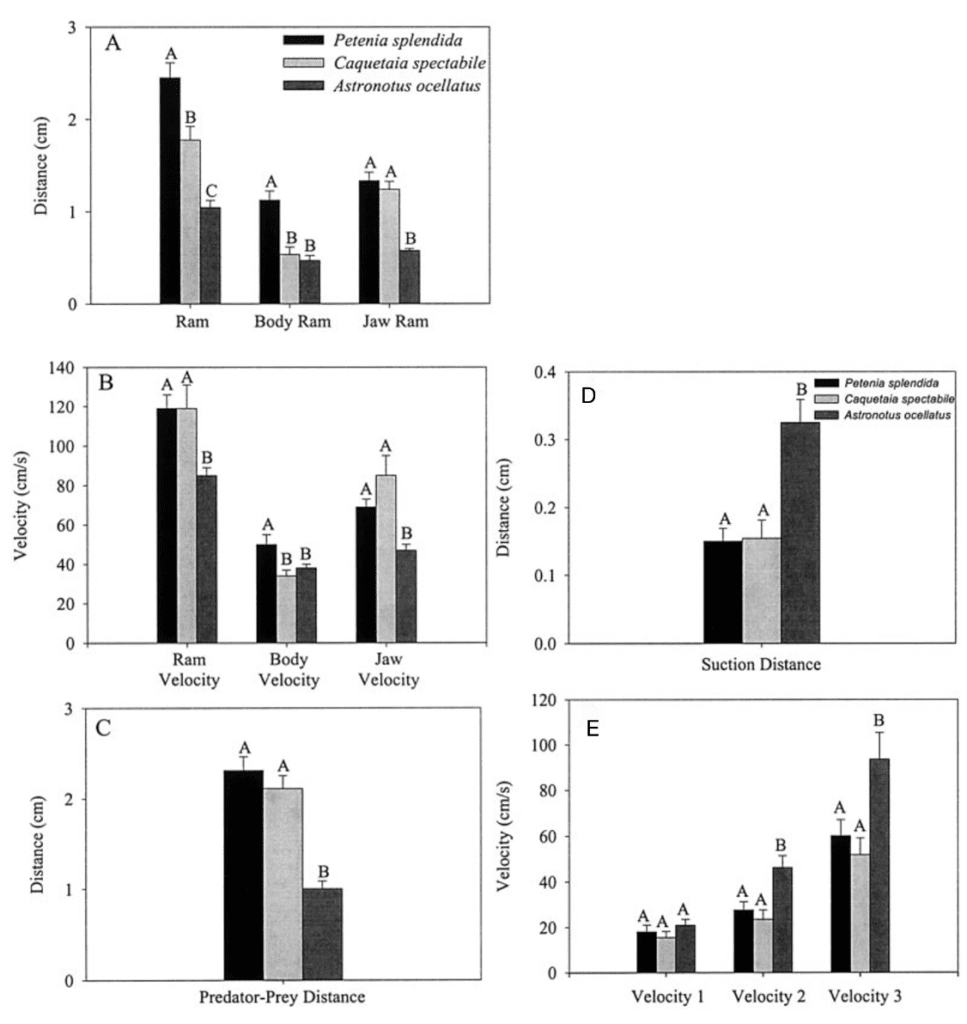
Fig. 19 : Mean values and standard errors of suction performance variables during feeding on live guppy prey (Waltzek & Wainwright, 2003).
The first three graphs of Fig. 19 compare these three species in terms of the distance the predator moved because of locomotion (body ram), upper jaw protrusion (jaw ram), and the combined total movement of the predator (ram) (A), predator body velocity, jaw velocity, and the combined ram velocity (B), and predator–prey distance at mouth opening (C). In order to analyze how protrusion enhances ram performance, one can focus on jaw ram and velocity. In these graphs, Petenia splendida and Caquetaia spectabile exhibited greater initial predator prey-distance and ram velocity than Astronotus ocellatus. These are primarily due to increased upper jaw protrusion in the first two mentioned species. In the last two graphs, the distance the prey moved toward predators because of suction generation (D), and average prey velocity are compared for the same three species (E). In this study, average prey velocity was calculated from the last four frames before the prey’s center of mass entered the predator’s mouth. Velocity 1 is the velocity from frames 4 to 3, velocity 2 is the velocity from frames 3 to 2, and velocity 3 is the velocity from frames 2 to 1, with frame 1 being the frame before the prey’s center of mass passed the predator’s mouth. Here, Astronotus ocellatus exhibited a higher suction distance and suction-induced prey velocity than Petenia splendida or Caquetaia spectabile. Thus, the extreme jaw-protruding taxa appear to generate less suction than smaller ones. Interestingly, in a different study (Wainwright et al., 2001) no significant difference was found in suction distance between Astronotus ocellatus and Petenia splendida. This interpretation is complicated because attack velocities were highest in P. splendida and C. spectabile, indicating that the suction-induced flow does not reach the prey until later in the strike, potentially constraining suction distance (Waltzek & Wainwright, 2003). Overall, these observations confirm that jaw protrusion mechanisms enhance feeding performance in fishes.
Pharyngeal Jaw
The complexity of fish jaws goes beyond everything developed previously since they do not even have just a single set of jaws. Pharyngeal jaws are a second set of jaws contained within an animal’s throat or pharynx distinct from the primary or oral jaw. While the oral jaw is used to catch prey as seen previously, the pharyngeal jaw is used to break up food (Fig. 20). Catching and processing prey independently provides fish with a better feeding performance, since they can do both at the same time. Also, the pharyngeal jaw has other functionalities related to sound production.
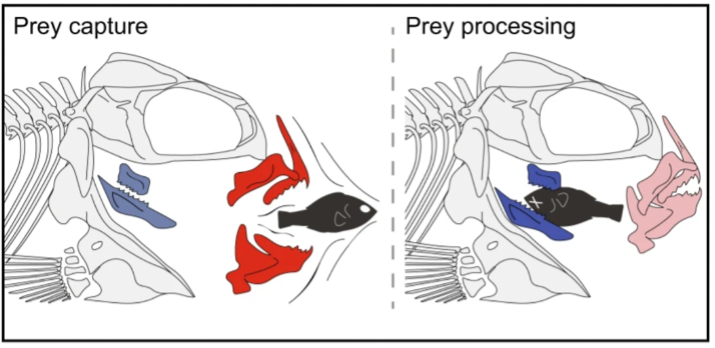
Fig. 20 : Schematic illustrating the relative roles of each jaw complex in prey capture and prey processing (Conith & Albertson, 2021)
The pharyngeal apparatus involves simultaneous upper jaw depression and retraction against a stabilized and elevating lower jaw. The movement is achieved by overlapping activity in most branchial muscles (Fig. 21) and can be divided into three phases. The first one involves four muscles, namely obliquus dorsalis 3, levator posterior, levator externus 3/4, and obliquus posterior, that act to depress the upper jaws. Then, the retractor dorsalis activates causing upper jaw retraction. Finally, the activity in several muscles, including transversus ventralis, pharyngocleithralis externus, pharyngohyoideus, and protractor pectoralis attach to the lower jaws (Wainwright, 1989). That way, fish can elevate and stabilize their lower jaws against the depressing and retracting upper jaws. Thanks to this strong depression of the upper pharyngeal jaws, many fish can crush hard prey in their pharyngeal apparatus.
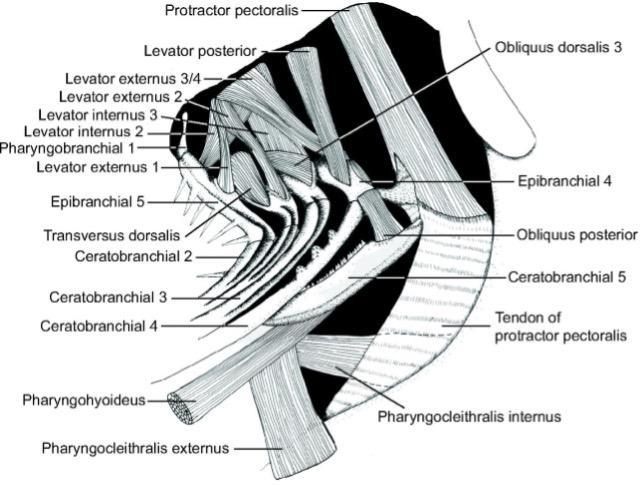
Fig. 21 : Left lateral line drawing of the muscles of the branchial basket of H. flavolineatum (Bertucci et al., 2014)
These pharyngeal jaws are not only involved in food processing but are also related to sound-producing mechanisms. It is well known that many fishes produce sound in various social contexts, such as agonistic interaction, courtship, or to defend territories (Amorim & Neves, 2008; Amorim et al., 2003). A recent study on grunts (Bertucci et al., 2014) showed that the sound produced by this species is directly connected to its pharyngeal jaw. Grunting sounds result from teeth scratching between the upper pharyngeal jaw and the lower pharyngeal jaw. This type of sound is usually a simple by-product of food processing. However, the similarity of sounds produced during food processing and in distress situations means that these two processes are related and have identical kinematic patterns involving cyclic jaw movements. In fact, there are observed similarities in motor patterns of the upper and lower pharyngeal jaws between food processing and sound production. Sounds produced in distress situations and during food processing were recorded and compared by Bertucci et al. (Bertucci et al., 2014). In distress situations, sounds consisted of grunts uttered alone or in a series of 2.2±2 grunts and had a dominant frequency of 718±180 Hz, which did not vary significantly in sounds recorded during food processing (536±148 Hz). The number of pulses within a grunt (5±2) did not vary significantly either. However, some differences can be observed. The duration and period of distress calls are longer than food-processing sounds. In distress situations, grunts lasted 47±11 ms, with a period of 155±31 ms, whereas sounds recorded during food processing showed a significantly shorter duration of 27±9 ms and period of 108±30 ms than distress calls. This suggests that the same mechanism could be used but at different speeds. This analysis leads us to the conclusion that the same mechanism is involved in mastication and sound production, and thus pharyngeal jaws would not only be essential for mastication and food processing but also serve for communication. The mechanism of sound production could be an example of exaptation (Parmentier et al., 2007).
In conclusion, fish use their jaw for multiple complex behaviors which require very specific mechanisms. Food suction, modelled by the hydrodynamic model, as well as protrusion, enhance their prey-catching ability. Moreover, their pharyngeal jaw not only makes the food processing and prey catching simultaneous, which greatly enhances fish feeding performance in their environment, but also provides fish with the ability to produce sounds for communication purposes in distress situations.
Latch Mediated Spring Actuation (LAMSA) System Utilized By Invertebrates
Introduction of LAMSA System and a Latch and Spring
A common trade-off exists in living organisms between the amount of force that can be generated by a muscle, the size of the organ/body part in question, and the velocity of the action in question (Burress & Muñoz, 2022). This is referred to as the force-velocity trade-off in order to maximize power output. With a larger organ, and therefore a larger weight, the muscles lack the ability to generate as great of a force, and therefore velocity, as they would with a lighter body part (Arnold et al., 2011). One major way that animals have figured out how to overcome this trade-off is by incorporating a latch-spring mechanism, called a latch-mediated spring actuation (LaMSA). This system uses concepts of closed kinematic chains as well as elastic properties to form a complex system that can generate greater forces than muscles can on their own. The complexity of the LaMSA system is an example of how closed kinematic chains have evolved to allow animals to overcome trade-offs and create forces at speeds that would previously have been unheard of (Divi et al., 2020).
This system works by building up potential energy in a spring and then converting it to kinetic energy by the release of a latch. This, therefore, produces more energy than can originally be achieved without the spring and solely the animals’ muscles (Divi et al., 2020). The potential energy of a spring can be represented by the following equation:

k is the spring constant which depends on the specific spring’s characteristics. The spring constant and potential energy are directly proportional, and therefore with a larger spring constant, more potential energy can be created. Potential energy can also be applied to the following formula:

F is the force applied, and x is the extension of the spring. These formulas allow for a deeper insight into the forces and energies involved in the LaMSA system, as will be seen with ants and mantis shrimp. Incorporating these types of springs with a high spring constant can allow the animals to generate more force in their jaws (Burress & Muñoz, 2022).
The spring constant is also proportional to the amount of force that is being put on it, and this can be represented by Hooke’s Law:

This equation demonstrates an object being deformed (the elastic properties allow for this) and the force is equal to the spring constant multiplied by the displacement of the deformation. With a greater deformation, therefore a larger x, the amount of force generated is greater and therefore there is an increased potential energy that will eventually transform into kinetic energy once the latch is released (Khan, 2008). A latch is a temporary hold on the spring preventing it from being released. These latches are vital to the overall function of the trap jaw as it is the component that allows the energy to be built up as potential energy and eventually be converted into kinetic energy. When the spring cannot be further compressed, an actuator, a muscle or motor, is responsible for releasing the latch and allowing the muscles to contract and trap shut. Once the latch is released, the average velocities reached by trap jaw ants were approximately 38.4 m/s, demonstrating one of the quickest mechanisms known to living organisms (Divi et al., 2020).
This system is vital in biology because it allows for the force of certain animal jaws to be greater than what solely their muscles would be capable of. With this system, the velocity-force trade-off is overcome, and a greater force is generated. The fact that this trade-off can be minimized as a problem allows for important biological applications such as in many predatory-prey interactions. Animals with this type of jaw often use it to quickly catch their prey or as a defense mechanism to escape dangerous situations. This system is most widely recognized to be used in the jaws of ants and mantis shrimp (Arnold et al., 2011).
The Mechanism of the LAMSA System in the Jaws of Specific Animals
In ants, there exists a snap jaw as well as a trap jaw. While these two types of jaws share major similarities, some differentiating characteristics exist. In both jaw types, two mandibles protrude from the top of the head, and they are flattened, bent structures originally set in an open state (Divi et al., 2020). Both types contain numerous muscles in their head, involved in their fast-acting movements, and they all act together in a closed kinematic chain. As illustrated in Fig. 22 below, the contractions of the muscles are depicted and demonstrate the synergistic effect of all the muscles resulting in one cohesive movement. As can be seen, the muscles that operate the jaw are highlighted specifically in blue, red and white, while purple is the mandible itself (Dockrill, 2017).
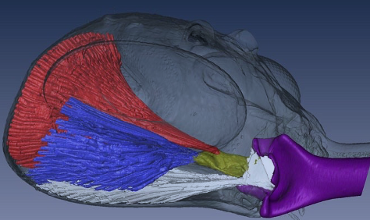
Fig. 22 : Muscles present in the skull of ants contributing to jaw movements and energy storage (Dockrill, 2017).
Fig. 23 below depicts a trap jaw mechanism. In this type of jaw, ants first stretch out their mandibles, putting them at an angle of up to 180 degrees (Patek et al., 2006). The exoskeleton undergoes a deformation during this process, which, following Hooke’s law, causes a force directly proportional to the amount of deformation caused. As seen in potential energy formulas, the force causes potential energy to be stored. Once the force causes a maximum deformation allowed by the exoskeleton, an actuator causes the muscles to contract, analogous to a latch being released and the mandibles trap being shut at speeds reaching up to 193 km/h (Thompson, 2017).
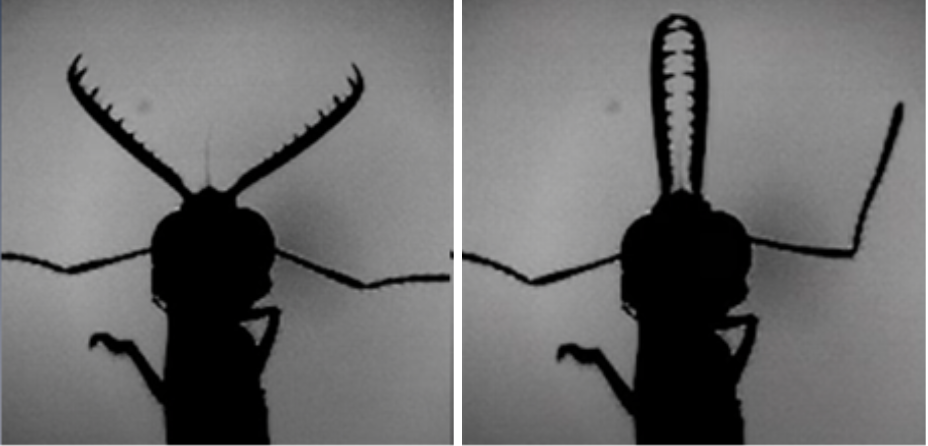
Fig 23 : Closed (A) vs. open (B) mandibles in the trap jaw ants (Thompson, 2017).
Snap jaw ants have a similar mechanism with slight differences. They contain two mandibles; one is associated with the latch mandible, and the other with the strike mandible. As the muscles in the jaw generate more and more energy, the force accumulates in the mandibles, and they begin to lean onto each other with a greater force, exerting force in the form of torque onto each other (J. et al., 2018). Similar to trap jaw ants, as the force exertion increases, the mandibles continue to deform, creating a larger force demonstrated by Hooke’s law, therefore generating potential energy, as can be seen in Fig. 24. There exists a latch radius where the mandibles lie within as they are in that energy storage phase and are building up the potential energy. Once the latch mandible exits this radius and the deformation in the mandibles gets too large, the mandibles collapse and snap shut, grabbing their prey within that area (Divi et al., 2020). The latch is released when the latch mandible slides across the strike mandible, and all the potential energy is then converted into kinetic energy.
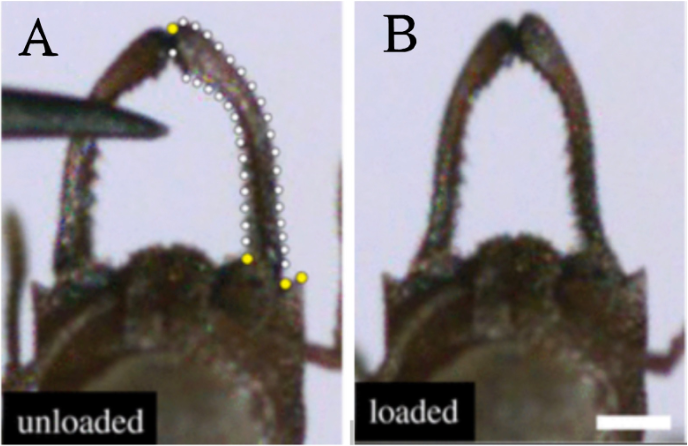
Fig. 24 : The mandibles of a snap jaw ant in the process of energy storage in the form of potential energy (J. et al., 2018).
These two systems demonstrate one of the fastest known systems in animals. It has been studied that the snap or trap shut mechanism of ants is 700 times faster than the blink of an eye. The snap and trap shut ants both use these mechanisms to capture prey, however, other animals use these types of systems as means of defence, or even to travel from one surface to another by amplifying their jump. Another method is to crush a hard shell in order to capture prey, and this can be observed in the mantis shrimp.
Mantis Shrimp
The mantis shrimp demonstrates another example of using the LaMSA system to maximize power output and override the force-velocity trade-off. In the mantis shrimp, by contrast with the ant, their power amplification system has only been proven to have evolved once. This demonstrates that perhaps their system is more efficient than the snap jaw ants. While the two systems contain major similarities, particularly in the energy storage systems, the goals of the two animals differ. While the ants contain two mandibles that snap or trap shut, the mantis shrimp uses its one appendage to generate a large amount of force to destroy a hard shell of prey (Anderson et al., 2014).
As shown in the image below, the mantis shrimp appendage that lies at the top of their heads is the effector organ (Divi et al., 2020). This system begins with potential energy accumulating in the merus section (the top section of their skull) by causing a deformation in the exoskeleton. Following this deformation, a 4-bar linkage system is vital for converting this elastic energy into kinetic energy and causing the seamless movement at incredible velocities (McHenry et al., 2012). Due to the rotating motion of the appendage, the force is released in the form of torque as shown in Fig. 25 below. The merus flexor muscles exert an opposite but equal force onto the spring, which causes the equilibrium to be at 0, allowing for the muscles to store potential energy before it can be released. These muscles are analogous to latches, and once they are released, the energy is converted to kinetic energy reaching speeds of up to 80.5 kilometers per hour (Dimery, 2019).
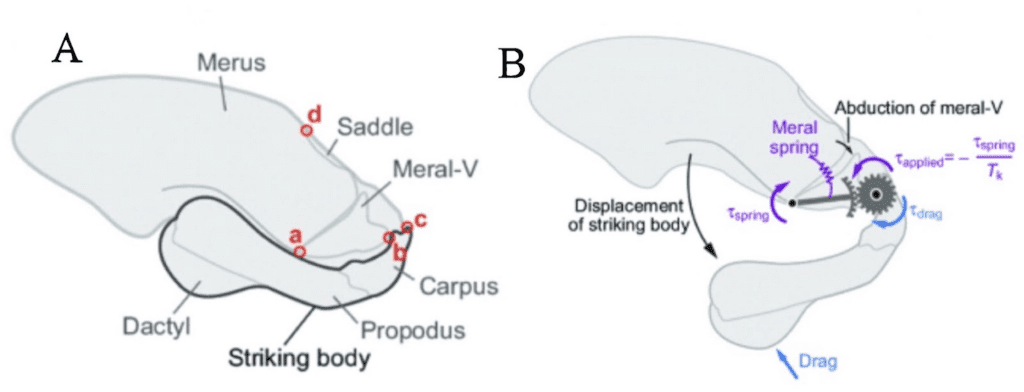
Fig. 25 : The apparatus present in mantis shrimp jaws as potential energy is building up and released in the form of torque (McHenry et al., 2012).
This power amplification system seen in ants and mantis shrimp is beginning to become present in many other species as well such as spiders, fleas and more. The LAMSA system utilizes the concepts of potential energy and springs to its advantage to gain an upper hand against their predators and more efficiently capture prey (Anderson et al., 2014). Not only does this provide a useful demonstration of the complexities of jaw systems, but it also exemplifies useful concepts that could be learnt from evolution as to how to amplify a mechanical system and override the trade-offs imposed on a species.
Conclusion
To catch a prey and process it properly without damaging their own apparatus, animal jaws need a certain force, precision, stability, and a safe mechanism. The modeling of jaws has evolved greatly with new technologies and discoveries. In the past, lever systems have been used to collect data about jaw movement, but a lot of complexity of the jaw mechanisms was neglected with this model. Although jaws and lever systems have common points, jaws are far more intricate. In lever systems, many biological properties and the DOFs of jaws are not taken into consideration. By using example animals, this essay dissected the limits of lever systems and other model options that could replace it. Closed kinematic chains are a new and improved theory that is often applied to mechanics but can relatively accurately represent jaws. More specifically, the 4-bar chain. This new model is more adapted to jaws since it can represent the implication of jaws in a much bigger mechanism and can respond to changing conditions. It also solves a conundrum by allowing feeding apparatus to be modeled as precise and robust through an engineering lens. As we studied, this applies to multiple animals such as fish, eels, mantis shrimps and also insects. The jaw mechanisms of fish have lots of particularities. Suction feeding, protrusion and the pharyngeal jaw make all of the mechanisms complex to model. Hydrodynamic models can be used in the case of fish to cover their indirect behaviors that are simplified with a lever system. In invertebrates, the latch mediates spring actuation and is used to represent jaws. This system allows new greater forces in animal jaws – more force than what their muscles are capable of. The close kinematic chain theory is evolving greatly and mechanisms like that of biotensegrity, the hydrodynamic models and the LaMSA models are continuously used by scientists and researchers to explain animal jaws.
References
Amorim, & Neves. (2008). Male painted gobies (Pomatoschistus pictus) vocalise to defend territories. Behaviour, 145(8), 1065-1083. https://doi.org/https://doi.org/10.1163/156853908784474498
Amorim, M. C. P., Fonseca, P. J., & Almada, V. C. (2003). Sound production during courtship and spawning of Oreochromis mossambicus: male–female and male–male interactions. Journal of Fish Biology, 62(3), 658-672. https://doi.org/https://doi.org/10.1046/j.1095-8649.2003.00054.x
Anderson PSL, Claverie T, Patek SN. Levers and linkages: Mechanical trade-offs in a power-
amplified system. Evolution (N Y). 2014;68(7):1919-1933. https://www.jstor.org/stable/24033792#metadata_info_tab_contents
Arnold, A. S., Richards, C. T., Ros, I. G., & Biewener, A. A. (2011). There is always a trade-off between speed and force in a lever system: comment on McHenry (2010). Biol Lett, 7(6), 878-879; discussion 880-871. https://doi.org/10.1098/rsbl.2011.0431
Balaji, N. N. (2017). Dynamics and Control of Closed Kinematic Chains: A Numerical Investigation. https://doi.org/10.13140/RG.2.2.15304.62726
Bellwood, David R., Goatley, Christopher H. R., Bellwood, O., Delbarre, Daniel J., & Friedman, M. (2015). The Rise of Jaw Protrusion in Spiny-Rayed Fishes Closes the Gap on Elusive Prey. Current Biology, 25(20), 2696-2700. https://doi.org/https://doi.org/10.1016/j.cub.2015.08.058
Bemis, W. E., & Lauder, G. V. (1986). Morphology and function of the feeding apparatus of the lungfish, Lepidosiren paradoxa (Dipnoi). Journal of Morphology, 187(1), 81-108. https://doi.org/https://doi.org/10.1002/jmor.1051870108
Bertucci, F., Ruppé, L., Van Wassenbergh, S., Compère, P., & Parmentier, E. (2014). New insights into the role of the pharyngeal jaw apparatus in the sound-producing mechanism of Haemulon flavolineatum (Haemulidae). Journal of Experimental Biology, 217(21), 3862-3869. https://doi.org/10.1242/jeb.109025
Burress, E. D., & Muñoz, M. M. (2022). Functional Trade-offs Asymmetrically Promote Phenotypic Evolution. Syst Biol.https://doi.org/10.1093/sysbio/syac058
Camp, A. L., Roberts, T. J., & Brainerd, E. L. (2015). Swimming muscles power suction feeding in largemouth bass. Proceedings of the National Academy of Sciences, 112(28), 8690-8695. https://doi.org/doi:10.1073/pnas.1508055112
Cassini, G. H., & Vizcaíno, S. F. (2012). An Approach to the Biomechanics of the Masticatory Apparatus of Early Miocene (Santacrucian Age) South American Ungulates (Astrapotheria, Litopterna, and Notoungulata): Moment Arm Estimation Based on 3D Landmarks. Journal of Mammalian Evolution,19(1), 9-25. https://doi.org/10.1007/s10914-011-9179-5
Claverie, T., Chan, E., & Patek, S. N. (2011). Modularity and scaling in fast movements: Power Amplification in mantis shrimp. Evolution, 65(2), 443-461. https://doi.org/https://doi.org/10.1111/j.1558-5646.2010.01133.x
Conith, A. J., & Albertson, R. C. (2021). The cichlid oral and pharyngeal jaws are evolutionarily and genetically coupled. Nature Communications, 12(1), 5477. https://doi.org/10.1038/s41467-021-25755-5
Cox, Philip G., et al. “The Jaw Is a Second-Class Lever in Pedetes Capensis (Rodentia: Pedetidae).” PeerJ, PeerJ Inc., 30 Aug. 2017, https://doi.org/10.7717/peerj.3741
Dimery, R. (2019, April 5). The mantis shrimp packs the most powerful punch in the Animal Kingdom. Guinness World Records. Retrieved September 30, 2022, from https://www.guinnessworldrecords.com/news/2019/4/the-mantis-shrimp-packs-the-most-powerful-punch-in-the-animal-kingdom-567501
Divi, S., Ma, X., Ilton, M., St. Pierre, R., Eslami, B., Patek, S. N., & Bergbreiter, S. (2020). Latch-based control of energy output in spring actuated systems. Journal of The Royal Society Interface, 17(168), 20200070. https://doi.org/doi:10.1098/rsif.2020.0070
Dockrill, P. (2017, August 31). Spring-loaded ant jaws snap shut on prey 700 times faster than you can blink. ScienceAlert. Retrieved October 1, 2022, from https://www.sciencealert.com/spring-loaded-ant-jaws-snap-shut-on-prey-700-times-faster-than-you-can-blink
Farooq, B., Hasan, O., & Iqbal, S. (2013). Formal Kinematic Analysis of the Two-Link Planar Manipulator. https://doi.org/10.1007/978-3-642-41202-8_23
Gibb, A. C., & Ferry-Graham, L. (2005). Cranial movements during suction feeding in teleost fishes: Are they modified to enhance suction production? Zoology, 108(2), 141-153. https://doi.org/https://doi.org/10.1016/j.zool.2005.03.004
Gibson, J. C., Larabee, F. J., Touchard, A., Orivel, J., & Suarez, A. V. (2018). Mandible strike kinematics of the trap-jaw ant genus Anochetus Mayr (Hymenoptera: Formicidae). Journal of Zoology, 306(2), 119-128. https://doi.org/https://doi.org/10.1111/jzo.12580
J., L. F., Fredrick J. Larabee Fredrick J. Larabee http://orcid.org/0000-0001-8404-0146 Department of Entomology, Larabee, F. J., Fredrick J. Larabee http://orcid.org/0000-0001-8404-0146 Department of Entomology, Smith, A. A., Adrian A. Smith Research and Collections, Suarez, A. V., Andrew V. Suarez Department of Entomology, Electronic supplementary material is available online at https://dx.doi.org/10.6084/m9.figshare.c.4310744., & Al., E. (2018, December 12). Snap-jaw morphology is specialized for high-speed power amplification in the Dracula Ant, Mystrium Camillae. Royal Society Open Science. https://royalsocietypublishing.org/doi/10.1098/rsos.181447
Kane, E. A., & Higham, T. E. (2015). Complex Systems Are More than the Sum of Their Parts: Using Integration to Understand Performance, Biomechanics, and Diversity. Integrative and Comparative Biology,55(1), 146-165. https://doi.org/10.1093/icb/icv033
Khan, S. (2008). What is Hooke’s law? (article). Khan Academy. Retrieved October 1, 2022, from https://www.khanacademy.org/science/physics/work-and-energy/hookes-law/a/what-is-hookes-law
Kinematic chain. (n.d.) Medical Dictionary for the Health Professions and Nursing. (2012). Retrieved September 29 2022 from https://medical-dictionary.thefreedictionary.com/kinematic+chain
Klasser, Gary D. “Overview of Temporomandibular Disorders (TMD) – Dental Disorders.” Merck Manuals Professional Edition, Merck Manuals, 19 Sept. 2022, https://www.merckmanuals.com/professional/dental-disorders/temporomandibular-disorders/overview-of-temporomandibular-disorders-tmd.
Lauder, G. V., & Liem, K. F. (1981). Prey capture by Luciocephalus pulcher: implications for models of jaw protrusion in teleost fishes. Environmental Biology of Fishes, 6(3), 257-268.https://doi.org/10.1007/BF00005755
Levin, S. (2013). Closed kinematic chain mechanisms comprise the fundamental mechanics of biologic movement and stability. https://www.researchgate.net/publication/256196463_Closed_kinematic_chain_mechanisms_comprise_the_fundamental_mechanics_of_biologic_movement_and_stability
Levin, S., de Solórzano, S. L., & Scarr, G. (2017). The significance of closed kinematic chains to biological movement and dynamic stability. Journal of Bodywork and Movement Therapies, 21(3), 664-672. https://doi.org/10.1016/j.jbmt.2017.03.012
Maquet, P. (1992). Iatrophysics to biomechanics. From Borelli (1608-1679) to Pauwels (1885-1980). J Bone Joint Surg Br, 74(3), 335-339. https://doi.org/10.1302/0301-620x.74b3.1587872
Matott, M. P., Motta, P. J., & Hueter, R. E. (2005). Modulation in Feeding Kinematics and Motor Pattern of the Nurse Shark Ginglymostoma cirratum. Environmental Biology of Fishes, 74(2), 163-174. https://doi.org/10.1007/s10641-005-7435-3
McHenry, Matthew & Claverie, Thomas & Rosario, Michael & Patek, s. (2012). Gearing for speed slows the predatory strike of a mantis shrimp. The Journal of experimental biology. 215. 1231-45. 10.1242/jeb.061465.
Mehta, R. S., & Wainwright, P. C. (2007). Raptorial jaws in the throat help moray eels swallow large prey. Nature, 449(7158), 79-82. https://doi.org/10.1038/nature06062
Muller, M. (1996). A novel classification of planar four-bar linkages and its application to the mechanical analysis of animal systems. Philos Trans R Soc Lond B Biol Sci, 351(1340), 689-720. https://doi.org/10.1098/rstb.1996.0065
Mutiara, Tresnati, J., Yanuarita, D., Irmawati, Yasir, I., Yanti, A., Rahmani, P. Y., Aprianto, R., & Tuwo, A. (2021). Urgent need for sustainable fishing of Blue-barred Parrotfish Scarus ghobban (Forsskal, 1775) in Wallace Line, Spermonde Islands, Makassar Strait, Indonesia. IOP Conference Series: Earth and Environmental Science, 860(1), 012015. https://doi.org/10.1088/1755-1315/860/1/012015
Olsen, A. M. (2019). A mobility-based classification of closed kinematic chains in biomechanics and implications for motor control. Journal of Experimental Biology, 222(21). https://doi.org/10.1242/jeb.195735
Olsen, A. M., Hernandez, L. P., & Brainerd, E. L. (2020). Multiple Degrees of Freedom in the Fish Skull and Their Relation to Hydraulic Transport of Prey in Channel Catfish. Integr Org Biol, 2(1), obaa031. https://doi.org/10.1093/iob/obaa031
Parent, Desiree.“Biomechanics: Lever Systems in the Body.” Visible Body 3d Human Anatomy. https://www.visiblebody.com/blog/biomechanics-lever-systems-in-the-body
Parmentier, E., Colleye, O., Fine, M. L., Frédérich, B., Vandewalle, P., & Herrel, A. (2007). Sound Production in the Clownfish Amphiprion clarkii. Science, 316(5827), 1006-1006. https://doi.org/10.1126/science.1139753
Patek, s., Baio, J., Fisher, B., & Suarez, A. (2006). Multifunctionality and mechanical origins: Ballistic jaw propulsion in trap-jaw ants. Proceedings of the National Academy of Sciences of the United States of America, 103, 12787-12792. https://doi.org/10.1073/pnas.0604290103
Patek, S. N., & Caldwell, R. L. (2005). Extreme impact and cavitation forces of a biological hammer: strike forces of the peacock mantis shrimp Odontodactylus scyllarus. Journal of Experimental Biology, 208(19), 3655-3664. https://doi.org/10.1242/jeb.01831
Rolland, L. (2022). Kinematics Synthesis of a New Generation of Rapid Linear Actuators for High Velocity Robotics with Improved Performance Based on Parallel Architecture. https://www.researchgate.net/publication/221908918_Kinematics_Synthesis_of_a_New_Generation_of_Rapid_Linear_Actuators_for_High_Velocity_Robotics
Scarr, G., & Harrison, H. (2017). Examining the temporo-mandibular joint from a biotensegrity perspective: A change in thinking [journal article]. Journal of Applied Biomedicine, 15(1), 55-62. http://dx.doi.org/10.1016/j.jab.2016.10.002.
Sheldon, Robert. (2022). “What Is Degrees of Freedom (Mechanics)?” WhatIs.com, TechTarget, https://www.techtarget.com/whatis/definition/degrees-of-freedom.
Soobramoney, S., & Perrin, M. R. (2007). The effect of bill structure on seed selection and handling ability of five species of granivorous birds. Emu – Austral Ornithology, 107(3), 169-176. https://doi.org/10.1071/MU05018
Sutton, G. P., St Pierre, R., Kuo, C.-Y., Summers, A. P., Bergbreiter, S., Cox, S., & Patek, S. N. (2022). Dual spring force couples yield multifunctionality and ultrafast, precision rotation in tiny biomechanical systems. Journal of Experimental Biology, 225(14). https://doi.org/10.1242/jeb.244077
Thompson, A. (2017, November 14). Researchers uncover intricate mechanism of trap-jaw ants. Popular Mechanics. Retrieved October 1, 2022, from https://www.popularmechanics.com/science/animals/news/a28045/trap-jaw-ants-mechanism/
Wainwright, P. C. (1989). Functional morphology of the pharyngeal jaw apparatus in perciform fishes: An experimental analysis of the haemulidae [https://doi.org/10.1002/jmor.1052000302]. Journal of Morphology, 200(3), 231-245. https://doi.org/https://doi.org/10.1002/jmor.1052000302
Wainwright, P. C., Alfaro, M. E., Bolnick, D. I., & Hulsey, C. D. (2005). Many-to-One Mapping of Form to Function: A General Principle in Organismal Design?1. Integrative and Comparative Biology, 45(2), 256-262. https://doi.org/10.1093/icb/45.2.256
Wainwright, P. C., Ferry-Graham, L. A., Waltzek, T. B., Carroll, A. M., Hulsey, C. D., & Grubich, J. R. (2001). Evaluating the use of ram and suction during prey capture by cichlid fishes. J Exp Biol, 204(Pt 17), 3039-3051. https://doi.org/10.1242/jeb.204.17.3039
Wainwright, P. C., Smith, W. L., Price, S. A., Tang, K. L., Sparks, J. S., Ferry, L. A., Kuhn, K. L., Eytan, R. I., & Near, T. J. (2012). The Evolution of Pharyngognathy: A Phylogenetic and Functional Appraisal of the Pharyngeal Jaw Key Innovation in Labroid Fishes and Beyond. Systematic Biology, 61(6), 1001-1027. https://doi.org/10.1093/sysbio/sys060
Waltzek, T. B., & Wainwright, P. C. (2003). Functional morphology of extreme jaw protrusion in Neotropical cichlids. Journal of Morphology, 257(1), 96-106. https://doi.org/https://doi.org/10.1002/jmor.10111
Westneat, M. W. (2005). Skull Biomechanics and Suction Feeding in Fishes. In Fish Physiology (Vol. 23, pp. 29-75). Academic Press. https://doi.org/https://doi.org/10.1016/S1546-5098(05)23002-9
Westneat, M. W., & Olsen, A. M. (2015). How fish power suction feeding. Proceedings of the National Academy of Sciences, 112(28), 8525-8526. https://doi.org/doi:10.1073/pnas.1510522112
Zuber, P. (2012). Four-Bar Linkages. Illinois.edu. http://dynref.engr.illinois.edu/aml.html
Schoenfeld B. Snarr R. L. & National Strength & Conditioning Association (U.S.). (2022). Nsca’s essentials of personal training (Third). Human Kinetics. Www.nsca.com. https://www.nsca.com/education/articles/kinetic-select/kinematic-and-kinetic-chains/#:~:text=In%20engineering%2C%20a%20series%20of%20linkages%20is%20referred
Van der Meij, M. A., & Bout, R. G. (2006). Seed husking time and maximal bite force in finches. J Exp Biol, 209(Pt 17), 3329-3335. https://doi.org/10.1242/jeb.02379
Van Regenmortel, M. H. (2004). Reductionism and complexity in molecular biology. Scientists now have the tools to unravel biological and overcome the limitations of reductionism. EMBO Rep, 5(11), 1016-1020. https://doi.org/10.1038/sj.embor.7400284