Abstract
A cursory glance at the diverse set of tails found in the animal kingdom quickly reveals an assortment of differently shaped tools meant to carry out an equally diverse, but often also similarly positioned, set of biomechanical roles. However, there are also somewhat more obscure functions that lay beyond the more obvious physical applications of the tail as an appendage that can be found when observing the tail’s internal systems on a chemical and often microscopic level. From the autotomy and regeneration of the tail, and the various fluids produced and dispensed by skunks and scorpions, to the influence of hormones on the properties of tails, there is an abundance of lenses that can be used to observe and understand some of the more unique animal tails and the purpose of chemistry in their functioning. Areas that will be covered include tail microstructures, conditions for tail fracture, gene expression in regeneration, venom toxin composition, neurotoxin effects, the synergistic relationship between certain hormones and the smell of certain chemicals.
Introduction
Chemistry –the science of studying elements and compounds– has found universal applications in multiple fields, from chemical engineering to medicine. One interesting tool that knowledge of chemistry provides is a better understanding of the diverse functions in living organisms, or, in other words, in the more general field of biochemistry. In our case, this paper will observe biochemistry as it pertains to the tails of different animals and witness how diverse its applications and relationships with chemistry can be.
The vast number of functions that tails have adapted in different vertebrae incite a fascinating investigation into the intricacies of biochemistry. The only uniting characteristic of tails is their location on a vertebrate: posterior to the anus. Therefore, when trying to discern the various levels at which chemistry is involved in the tail, there is a multitude of different paths one could take. In the case of this paper, the focus will be on autotomy and tail regeneration, the production and administration of toxins, venoms, and all sorts of weaponized fluids as well as the hormonal control of tail properties. More specifically, the lizard tail’s microstructures, the specialization that allows for tail regeneration, its skeletal development, the composition and use of toxins, venoms, and any such internally produced fluids, along with the innate immunity that the producers have towards their own dangerous fluids and the synergistic properties of hormones in tail properties, will all be dissected in this paper.
Autotomy and Regeneration in Lizard Tails
Autotomy: Understanding the Paradox
Autotomy is the process of self-amputation. Lizards have the capacity to perform autotomy of their own tail. The autotomy and regeneration process of a tail is complex and unique. It has been observed to be an adaptation to avoid predators, as the tail continues to writhe post-amputation, distracting the predator (Baban et al., 2022).
To make autotomy efficient, the structure of the lizard tail must be specialized to carry out this function. The majority of the time, the tail must be securely attached to the lizard’s body, but the tail must easily detach when desired or needed, which creates a near paradoxical requirement for the structure of the tail (Babban et al., 2022). Earlier studies into the microstructures present in the tail that created these conditions show diagrams with fracture planes located along the vertebrae of the tail (Araujo et al., 2010, Sanggaard et al., 2012). Figure 1 is a figure from Araujo et al. that shows the fracture plane of the tail as a schematic. The plane of cleavage is between two regions of muscle bundles. Figure 2 shows electron micrographs taken during their experiment showing the fracture plane at a variety of angles and magnifications. In particular, Figure 2A shows the wedge-shaped extension of the muscle bundles present on the distal end of the tail stump, consistent with the schematic of the fracture plane.

Figure 1. Schematic drawing of a longitudinal section of the middle portion of the tail showing autotomy planes (arrows). M, muscle; Ve, vertebra; A, adipose tissue; S, skin (Adapted from Araujo et al., 2010).

Figure 2. Scanning electron micrographs of the tail stump post-autotomy. A. The distal portion of the tail stump consists of muscle bundles (MB) which surround the vertebra (Ve) in the center. Scale bar: 500 μm. B. Higher magnification of a bundle of muscle fibers from Fig. 2A showing distal dilatation or bulging of the fibers (arrows). PC, plane of cleavage; S, skin. Scale bar: 100 μm. C. The distal portion of the tail stump showing bundles of muscle fibers (MB) and distal dilatations or bulges (arrows). PC, plane of cleavage. Scale bar: 100 μm. D. High magnification view of a muscle fiber, evident from the cross-striations, or ridges (arrows). The conspicuous dilatation at the distal end (B) does not have cross-striations. Scale bar: 10 μm (Araujo et al., 2010).
However, the observance of these fractal planes still does not complete the answer to the paradoxical nature of the tail. What keeps the tail together along these fractal planes? Sanggaard et al. sampled the liquid at the surface of the exposed tail stump post-autotomy and tested for proteolytic enzymes, which break down protein. They did not find the presence of these proteins, meaning that the fracture of the tail is not initiated by the breakage of proteins, and that the autotomy of the tail must be mechanical. Baban et al. expand on the understanding presented by these previous studies to produce a model of the micro and nano structures present on these wedge and socket fracture planes that make autotomy a realistic process. Figure 3 shows both SEM images from the autotomy of a Hemidactylus flaviviridis lizard along with a computer-generated model of the proposed structures present. In this figure, we can observe the plug and socket conformation of the fracture plane at a variety of scales, from the 8 wedges in the mm range on the distal end, to the mushroom-shaped micropillars in the 100 µm range, and the nano-beads present in the nm scale. These diagrams indicate that the attachment of the tail is contact-based, leading to a better understanding of how these surfaces connect pre-autotomy.
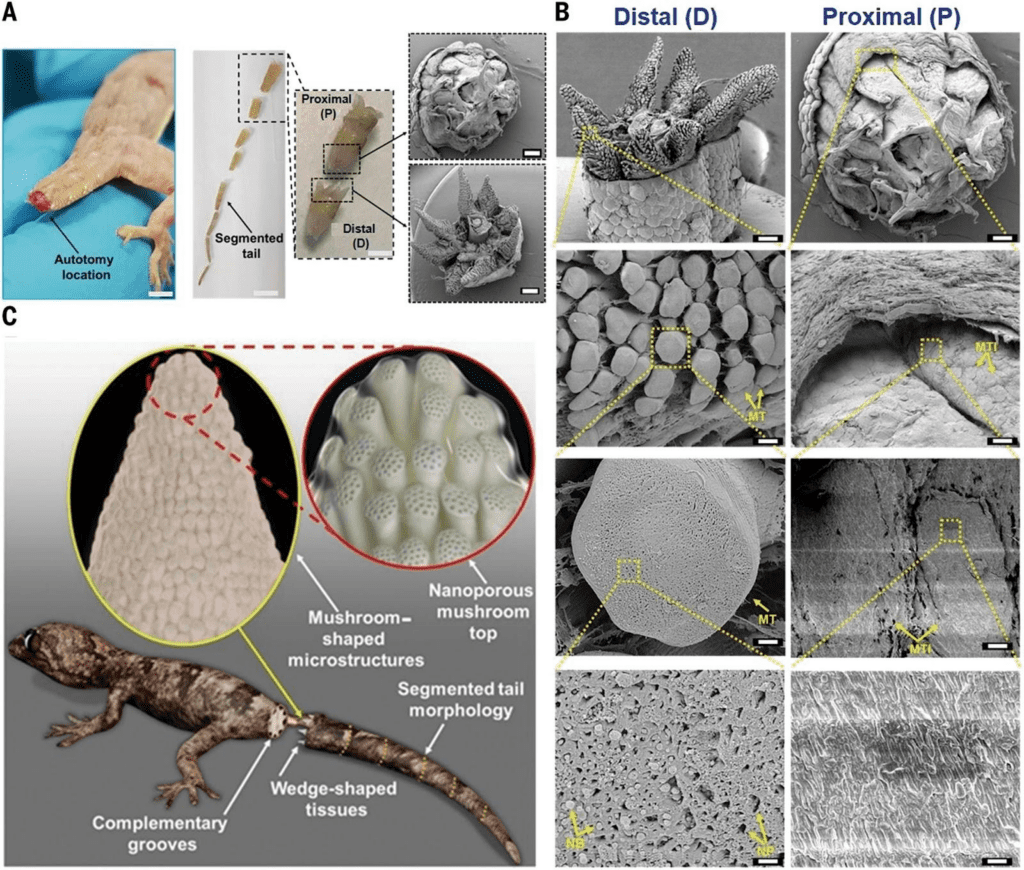
Figure 3. Scanning electron microscope (SEM) image of the post-autotomy surface of an H. flaviviridis tail. A. Autotomized tail location (scale bar: 1.5 cm). Segmented tail morphology (scale bar, 1 cm) shows the proximal part of the tail (P), and the distal part (D) (scale bar: 0.5 cm), in a plug-and-socket type assembly (scale bar: 1 mm). B. SEM of the distal (D) part showing the wedge-shaped tissues with highly dense mushroom-shaped microstructures (scale bar: 1 mm). The enlarged portion shows the mushroom-shaped micropillared arrangement (scale bar: 100 μm) with the single mushroom top labelled as MT (scale bar: 10 μm) containing the nanopores (NP) and nanobeads (NB) (scale bar: 1 μm). SEM of proximal (P) (scale bar: 1 mm) shows the corresponding mushroom top imprints indicated as MTI (scale bar, 100 μm). The single MTI (scale bar: 10 μm) shows a planar topology (scale bar: 1 μm). C. Hypothesized model of the lizard tail interface between two complementary segments before fracture, consisting of micropillared nanoporous top connections at the wedge-shaped tissue faces (Baban et al., 2022).
Baban et al. continued their investigation by observing the process by which the tail fracture is formed. Figure 4 shows images from their experiment. It is shown that a force applied parallel to the length of the tail does not generate a fracture plane, and the micro and nano structures in the tail are capable of retaining toughness, while a force applied at an angle with respect to the tail does initiate a fracture plane. This shows how the paradoxical nature of the lizard tail can be understood: the mushroom top and mushroom top imprints maintain contact until a bending force is applied to the tail.

Figure 4. A. Tensile mode, where the tail was fully stretched parallel to tail length; no fracture initiation was observed. B. Bending mode, where fracture initiation and catastrophic fracture propagation were both observed (Baban et al., 2022).
Regeneration: a Timeline
Given the microstructures present that create the unique autotomic abilities of the lizard tail, what is the process of lizard tail regeneration? The phenomenon of tail regeneration in lizards is a highly specialized process that has a set of unique characteristics, which makes it an interesting case study of tissue regeneration. One of these unique characteristics is that the lizard is only partially regenerative as a system (Lozito et al., 2016). Some species have regenerative capabilities, such as axolotls and zebrafish, which can both regenerate a variety of organs along with external appendages (Lozito & Tuan, 2015). On the other hand, some species, such as mammals, can regenerate particular tissues but not full organs or limbs. Lizards are able to regenerate their tail but they are not able to regrow their legs post-amputation (Lozito et al., 2016). This distinct capability of regeneration is an adaptation coupled with the autotomy of the tail. This process is likely a result of a defense mechanism: the loss of the tail makes escape easier from a predator. However, lizards are the only amniotes to have this ability for the purpose of selective regeneration (Lozito et al., 2016). There are two observed modes of tissue regeneration: fracture healing and blastema-based regeneration (Lozito et al., 2016). Fracture healing is where stem cells in the wound area differentiate into cartilage and, through a pathway, ossify into new bone (Lozito et al., 2016). However, lizard tails regenerate through blastema-based regeneration after autotomy. A blastema is a mass of cells that act as progenitors for new tissue. In this case, the new tissues are to recreate the tail.
The process of regeneration takes approximately 60 days and follows a set of consecutive steps (Hutchins et al., 2014). From 0 – 10 days post-autotomy (dpa), the tail stump undergoes wound healing and little outgrowth occurs. By 10 dpa, the tail stump is covered by a wound epithelium and there are blood vessels below this epithelium, which is shown in Figure 5B. From 10 – 15 dpa, outgrowth of the tail begins. At this time, there is an early growth of ependyma, which is made of ependymal cells that line the central canal of the spinal cord, into the surrounding mesenchymal tissue, which are unspecialized stem cells. There is also outgrowth of vascularized tissue, created from the growth of new blood vessels. Additionally, in this time frame, there is also the beginning of the formation of myofibers, or muscle fibers (Hutchins et al., 2014). All of these changes are shown and labelled in Figure 5C. From 15 – 20 dpa, the central cartilage tube begins to differentiate along with the surrounding skeletal muscle. However, the tip of the tail remains vascular, as shown and labelled in Figure 5D (Hutchins et al., 2014). From 20 – 25 dpa, the tail continues to lengthen and forms an ependymal core surrounded by developing muscle and cartilage. Also, myosin heavy chain (MHC) positive skeletal muscle is present along the entire length of the regenerated tail except for the distal tip (Hutchins et al., 2014). These changes are shown in Figure 5E. The final stage, 25 – 60 dpa consists of continual tail lengthening and additional differentiation. Post- 60 dpa, very little to no additional growth is observed, and tail regeneration is completed (Hutchins et al., 2014). The histology of the final regenerated tail is shown in Figure 5F, and an exterior view of the tail is shown in Figure 5A.
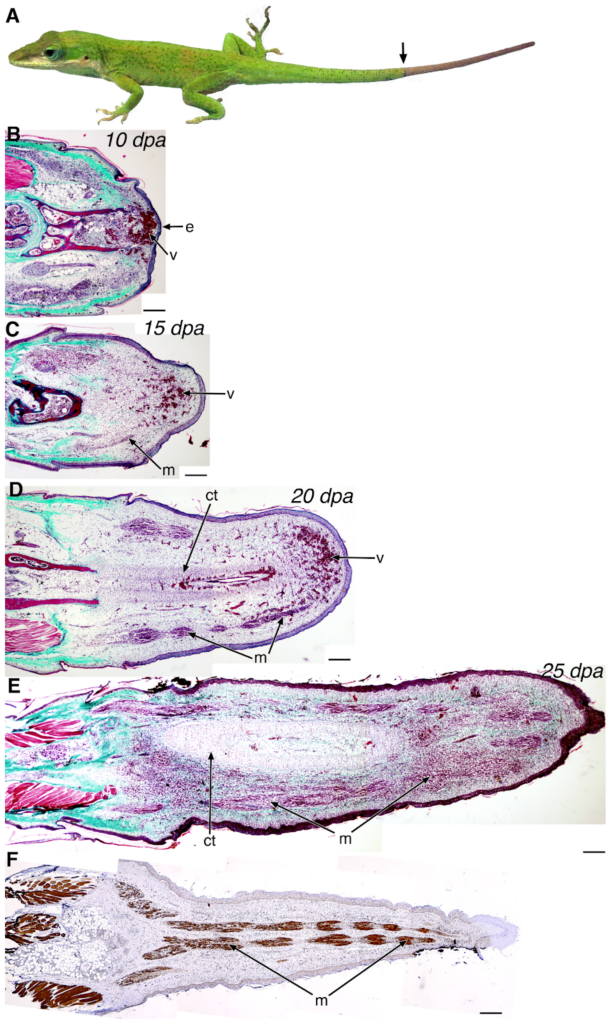
Figure 5. Overview of the stages of lizard tail regeneration. A. Anolis carolinensis lizard with a regenerated tail in the arrow to distal region. B-E. Histology of the 10 dpa (B), 15 dpa (C), 20 dpa (D), and 25 dpa (E) regenerating tail through Gomori’s trichrome stain; connective tissues and collagen stain green-blue; muscle, keratin, and cytoplasm stain red; nuclei are black. F. Immunohistochemistry of myosin heavy chain in a 25 dpa regenerating tail using the MY-32 antibody. e, wound epithelium; v, blood vessels; m, muscle; ct, cartilaginous tissue. Scale bars: 200 µm (Hutchins et al., 2014).
Regeneration: a Look into Gene Expression
The previous section described regeneration from a histological perspective, describing regeneration through the changes in tissue, but what is happening so that these changes are generated? Hutchins et al. performed RNA-sequence analysis on 25 dpa tails to determine which genes are expressed differentially through the process of regeneration. They found that approximately 326 genes are expressed differentially. To perform this, they segmented the regenerated tail into 5 different regions and found two different clusters of these 326 genes. Cluster I of 129 genes refers to genes that were mainly expressed in the proximal region, or base, of the regenerated tail, while Cluster II of 197 genes refers to genes that were mainly expressed in the distal region, or tip, of the regenerated tail. Hutchins et al. also found gene ontology groups for each of the genes, or categorizations of the functions of each gene. The categories for Cluster I are shown in Figure 6A and the categories for Cluster II are shown in Figure 6B. There are both overarching and general categories shown, as well as subcategories shown as a tree map.
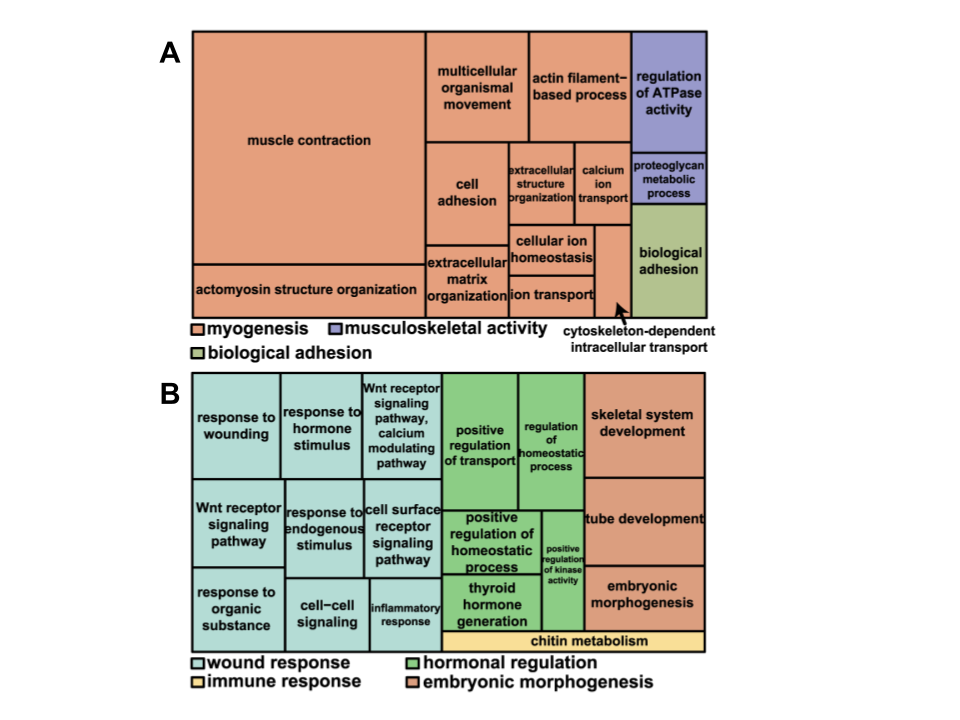
Figure 6. A treemap overview of differentially expressed genes in A. Cluster I and B. Cluster II based on representative Gene Ontology Biological Processes. Related terms are visualized with the same color, with the representative category for each color group denoted in the legend (Adapted from Hutchins et al., 2014).
The majority of gene ontology groups associated with the differentiation of tissues in the proximal region are related to muscle creation, growth, and repair. On the other hand, the gene ontology categories for the distal region were related to wound response, hormonal regulation, and embryonic morphogenesis. These differentially expressed genes indicate the variety of processes that must occur in order to regenerate the lizard tail.
Regeneration: Skeletal Development Through Endochondral Ossification
Discussing the process and pathway of each of these differentiated genes would be much too complicated to discuss in one paper, and a majority of the process is still not understood. However, one important process occurs at the interface of the tail stump and the regenerated tail. As the cartilaginous tube of the regenerated tail attaches to the last original tail vertebrae, the process very similarly resembles endochondral ossification, which is a process where bone is formed from cartilage (Lozito & Tuan 2015). This process is well-studied and understood, and occurs during vertebrate limb generation and tail/limb regeneration in urodeles (Lozito & Tuan 2015).
The process of endochondral ossification begins with mesenchymal cells differentiating into chondrocytes (Breeland, Sinkler, & Menezes 2022). Mesenchymal cells are a type of stem cell, while chondrocytes are a type of cell specifically responsible for cartilage formation. These chondrocytes multiply rapidly while secreting an extracellular matrix. The extracellular matrix forms a cartilage model, which is like a scaffold or template for bone formation. In the center of the cartilaginous model, chondrocytes begin hypertrophy, producing proteins needed for tissue remodeling and calcification (Breeland, Sinkler, & Menezes 2022). Calcification of the extracellular matrix begins, which leads to apoptosis, or cell death, of the chondrocytes. Blood vessels occupy the space left behind by the chondrocytes, and bring in osteogenic cells, which are stem cell precursors to more specialized bone cells. Then, osteoblasts, cells that form bone tissue, create a thickened region of compact bone, called the periosteal collar, which is the place where the primary ossification center occurs. At the end of the bone, epiphyseal plates also form, which is where cartilage is created and later turned into bone. These areas are also called growth plates (Breeland, Sinkler, & Menezes 2022).
This process can be compared to the phenomenon observed by Lozito & Tuan during lizard tail regeneration. They observed the blastema cells in the tail stump becoming chondrocytes, which form the cartilage tube. Around 21 dpa, they found that the chondrocytes in contact with the original portion of the tail performed hypertrophy. The various images and tests they performed to determine this are shown in Figure 7. While the general histology of the interface is shown in Figures 7A-B, Figures 7C-F show the variety of tests performed to confirm the hypertrophy of the proximal chondrocytes. Alk Phos (Fig. 7D) and BMP-6 (Fig. 7E) are both hypertrophic chondrocyte markers, while cartilage tube edges express Ihh (Fig. 7F) (Lozito & Tuan 2015).
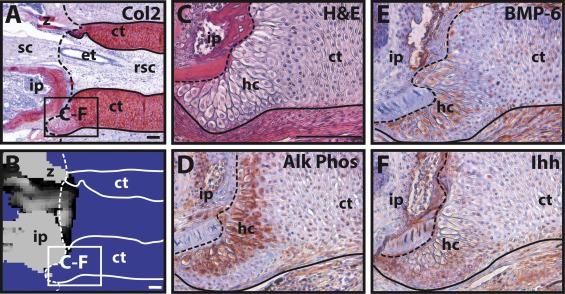
Figure 7. Hypertrophy of proximal-end chondrocytes in a regenerating tail. A-B. Boundary region between original and regenerated tail (21 dpa) analyzed by (A) Col2 IHC and (B) microCT. Solid line – cartilage tube boundary; dashed line – border between original tail and regenerating tail. C-F. Higher magnification of A-B images, border denoted in images A-B, analyzed by (C) histological staining (H&E) and Immunohistochemistry for (D) Alk Phos, (E) BMP-6, and (F) Ihh. ct, cartilage tube; et, ependymal tube; hc, hypertrophic chondrocyte region; ip, intervertebral pad; rsc, regenerated spinal cord; sc, spinal cord; z, zygapophysial joint. Scale bar = 100 µm (Lozito & Tuan 2015).
Then, at 35 dpa, the interface between this cartilage tube and the original tail vertebrae resembles a growth plate along with ossification centers made from osteoblasts, one of the later steps of endochondral ossification (Lozito & Tuan 2015). The histology of this step of the process is shown in Figure 8. Figure 4A shows the growth-plate like region of the interface, and Figure 4B shows how the ossification of the cartilage tube has made it continuous with the original vertebrae. Figures 4E-F show that the growth plate zone chondrocytes express Alk Phos and BMP-6. Additionally, osteoblasts are cathepsin k positive, so Figure 4H shows their location within the region. An important note is that Lozito & Tuan found that ossification of the cartilage tube terminated within 1 mm of the original cartilage tube and vertebrae interface. This means that the regenerated lizard tail is an imperfect copy of the original tail, forming a cartilaginous tube, except for the interface region, while the original tail was made from vertebrae.

Figure 8. The proximal cartilage tube mineralizes similarly to endochondral ossification. A-B. Boundary region between the original and regenerated tail (35 dpa) analyzed by (A) Col2 IHC and (B) microCT; solid line – cartilage tube boundary; dashed line – boundary between original (left) and regenerated (right) tail. C-H. Higher magnification views of regions identified in A-B, analyzed by (C) histological staining (Saf O/FG) and IHC for (D) Col2, (E) Alk Phos, (F) BMP-6, (G) Ihh, and (H) cathepsin K. Black arrowheads – cathepsin K-positive osteoclasts. ct, cartilage tube; gp, growth plate-like region; ip, intervertebral pad; oc, ossification center; po, periosteum; rsc, regenerated spinal cord; sc, spinal cord; z, zygapophysial joint. Scale bar = 100 µm (Lozito & Tuan, 2015).
The ability of the interface of the cartilage tube and the vertebrae to adopt a process similar to endochondral ossification is an important step in understanding the complex pathway of the regeneration of the lizard tail. This process explains how the cartilage tube connects to what previously remained on the tail, but there is still much to understand in terms of other processes that regenerate the lizard tail.
One important and interesting note, mentioned in Barr et al., covers the phenomenon of re-regeneration of the lizard tail. While autotomy cannot happen in the cartilaginous tube region, as there is not an appropriate fracture plane, if the tail breaks off for another reason, the tail is able to re-regenerate, as observed in Egernia Kingii (King’s skinks).
To conclude this section, the process of lizard tail autotomy and regeneration is an adaptation in order to avoid predation and begins with self-amputation of the tail made possible by a complex layout of microstructures, and continues through wound healing, outgrowth, specialization, and ossification of the cartilaginous tube near the distal end. While research on the regenerative abilities of the lizard tail is very intriguing to specialists studying tissue regeneration, the process is highly adapted and unique, which means that it has not yet led to any engineering breakthroughs or applications.
Secretion of Toxins in Scorpion Metasomas
Scorpions of the Arachnida class have highly venomous tails. They utilize this functionality both to capture prey and defend themselves. Their segmented tails, called metasomas, are characterized by a bulbous extremity known as a telson. The specific morphological and ultrastructural characterization of the telson varies depending on the species. However, all scorpion telsons are composed of two segregated venom glands, two venom ducts and a stinger (known as an aculeus). It is in these venom glands that the toxins are produced. For an example, see the morphological structure of the Neochactas delicatus in Figure 9.

Figure 9. Internal composition of the telson (left) and the fifth metasomal segment (right) of a Neochactas delicatus. Top: lateral view; bottom: dorsal view. The telson can be subdivided into the aculeus (Ac), the vesicle equaling a small sac (Ve) and the peduncle/joint (Pe). The venom glands are in transparent green, and the venom ducts are blue. Both can be found in the Telson inside the vesicle. Coagulated venom present in the ducts could explain their discontinuous colour. Scale bar: 4 mm (Van der Meijden & Kleinteich, 2016).
Depending on the venom composition, the sting delivered will vary from an uncomfortable swelling to a lethal dose in some cases. It is, therefore, pertinent to study the production of this toxic substance, its impact on the inflammatory system, as well as the incredible natural immune responses developed by some species.
Venom Toxin Composition
The venom secreted by the venom glands can be viewed as a cocktail of toxins. Some of these toxins activate voltage-gated sodium ion channels by targeting and binding themselves to specific molecules in the nerve cells. These ion channels, which come in threes, are situated on the outside of nerve cells for mammals and act like gates to the sensory system. The injection of venom disrupts the nervous system, subsequently causing intense pain. (Hopp et al., 2017). While the specific components of a scorpion’s venom vary from species to species and even from season to season, the toxins can be classified by structure, mode of action and binding site on the channel. (Petricevich, 2010) Furthermore, the action of these toxins can be classified in 2 categories: cytotoxins (referring to a toxicity for living cells) and neurotoxins (referring to a toxicity for the nervous system). The following components have been characterized thanks to advanced methods of chromatography, fractionation, and peptide sequencing (Petricevich, 2010).
First there are scorpion α-Toxins. These are cytotoxic membrane-damaging polypeptides which bind to site 3 on the sodium channel (See Figure 10 for illustration). α-Toxins prolong the action potential of muscles and nerves by quickly inactivating sodium channel receptors. Action potential refers to “a rapid rise and subsequent fall in voltage or membrane potential across a cellular membrane with a characteristic pattern” (Oxford languages). This change of electrical potential is critical to the functioning of the brain since it is what propagates information to the central nervous system and subsequently to the periphery (Bryne, 2021). This prolonged action potential is also known to cause tissue damage by disrupting the smooth muscle of the blood vessel (García-Hidalgo, 2022).

Figure 10. Schematic two-dimensional representation of the functional voltage-gated sodium ion channels and identification of known neurotoxin binding areas. The pertinent sites in studying scorpion toxins are sites 4 and 3 (Stevens et al., 2011).
Scorpion β-Toxins, which are not generally found in American scorpions, are neutral sphingomyelinases (hydrolase enzymes involved in a metabolic reaction) which bind to site 4 (see Figure 10 above) and produce a decrease in membrane potential. Membrane potential refers to the electrical potential between the inside and outside of a cell. It is what causes the direction of the movement of ions in a cell. When equilibrium of membrane potential is broken, ions will flow into the cell, resulting in depolarization. Once this depolarization occurs, repolarization is impossible, and the cell is no longer responsive to stimulation (DiBartola & Autran De Morais, 2012). This type of toxin can be either neurotoxic or cytotoxic.
These two types of toxins will either act upon the Sodium Channels, Potassium Channels or Calcium Channels.
Inflammatory Response
When scorpion venom interacts with the immune system, the inflammatory response depends on the cytotoxic and neurotoxic nature of the venom. However, the inflammatory reactions which occur are a result of the rapid production of antibodies and cytotoxins. Indeed, cytotoxins are produced by a body to kill harmful cells which have entered, and they can be either pro-inflammatory or anti-inflammatory (Petricevich, 2010). While these two compounds are imbalanced the body will suffer inflammatory symptoms. Their balance is thus crucial to finalizing the recovery process (See Figure 11).
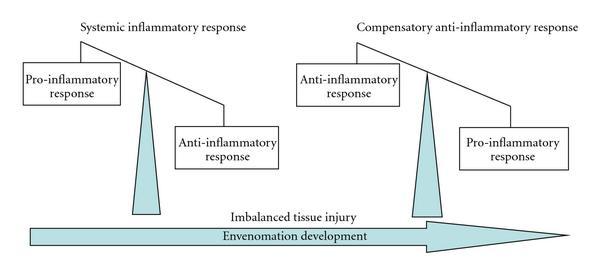
Figure 11. Diagram characterizing the envenomation development (Petricevich, 2010).
In Figure 11, one can observe how directly after the venom is injected, the pro-inflammatory response characterized by the release of pro-inflammatory cytotoxins is much greater than the anti-inflammatory response. As a result, the body overcompensates with the production of anti-inflammatory cytotoxins. Figure 12 depicts the final state of the envenomation development: tissue recovery.
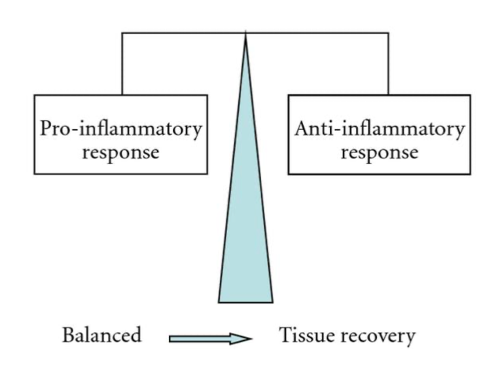
Figure 12. Diagram characterizing the link between tissue recovery and pro inflammatory/anti-inflammatory equilibrium (Petricevich, 2010)
Innate Immunity
Oddly, despite their toxic and sometimes highly lethal nature, scorpions are preyed upon by many animals. Pain is a useful adaptive function signaling tissue damage (Rowe et al., 2013). Therefore, most animals simply depend on innovative hunting strategies with quick and accurate movements aimed at removing the tail. However, some predators have developed an immunity to the toxins produced by a scorpion’s tail. The cases of the southern grasshopper mouse and the pallid bat are exceptional examples of evolutionary adaptation.
The Southern Grasshopper Mouse (Onychomys torridus) seems to have indeed developed a pain immunity or tolerance to the 6 toxins contained in The Bark Scorpion’s (Centruroides sculpturatus) venom. Both animals can be found in Figure 13.

Figure 13. Photograph of a bark scorpion (left) moments before it is devoured by the southern grasshopper mouse (right) (M Rowe & A Rowe, 2021). The full scene can be found under this link: https://www.youtube.com/watch?v=9lu65X8t8ig.
As stated previously, the pain caused by a scorpion injection is due to the toxin’s activation of voltage-gated sodium ion channels (Hopp et al., 2017).
Research from 2013 showed that, NAV 1.8, which is the channel responsible for transmitting pain signals to O. torridus’s central nervous system (CNS) “has amino acid variants [(namely glutamic acid and glutamine)] that bind venom peptides and inhibit channel current” (Rowe et al., 2013). The current specifically blocked by scorpion venom is TTX-R Na+. When venom enters its system, the channel therefore blocks pain signals instead of transmitting them, which explains the numbness O. torridus seems to exhibit to the Bark scorpion’s venom.
Furthermore, these pain receptors only seal once faced with these toxins. Researchers noticed that the mouse exhibited no pain response to the venom but did to formalin (a formaldehyde CH2O solvent). Lastly, it was noticed that a house mouse injected with formaldehyde exhibited more pain responsive behaviour than a O. torridus first injected with venom. This is because venom, by blocking the pain receptors, induces analgesia in O. torridus. This example is exceptional and unique as it allows the animal to maintain the gift of pain (this is indeed a gift!) while predetermining a situation in which the pain is not needed and neutralizing it.
It is clear that these amino acids are critical for venom-pain insensitivity as comparisons of orthologous sequences from 18 mammalian species exhibiting venom numbness showed that all of them expressed either glutamic acid or glutamine. These acids are critical for blocking sodium current in Nav 1.8.
The case of the pallid bat (Antrozous pallidus), shown in Figure 14, is relatively new.
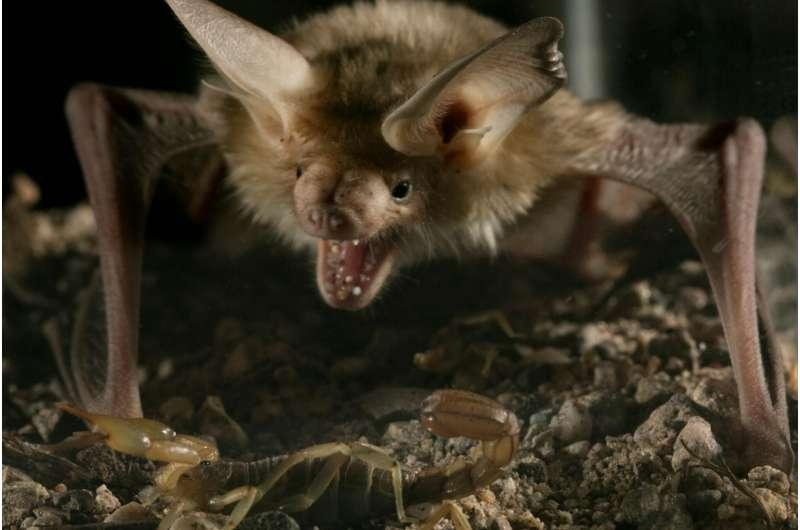
Figure 14. Photograph of the pallid bat preying on the Bark Scorpion (Provided by the university of California, 2017)
In 2017, researchers from the university of California conducted a study on these creatures and surprisingly found that the amino acid substitutions inhibiting pain signaling in the O. torridus were not present in the pallid bat, suggesting a novel venom resistance mechanism (Hopp et al., 2017). Research analysis suggests acid residues are the cause of this.
These mechanisms are particularly interesting for scientists who hope to use them as a basis for the conception of new pain killer technologies.
Production of Biochemicals
Hormonal Control of the Newt’s Tail Height
Hormones possess one of the most important roles when it comes to controlling an animal’s behavior and physiology. As a result, the tail, like any other appendage, is also influenced by the production and presence of certain hormones within the animal’s body. For example, it was observed in newts (Picture 1) collected from a small artificial pond near Charlottesville that tail height would fluctuate significantly for males throughout the year whereas a statistically insignificant difference of 7.15 mm for the females’ tail height was observed (Singhas et al., 1975). These fluctuations can be observed in the following figure (Figure 15).
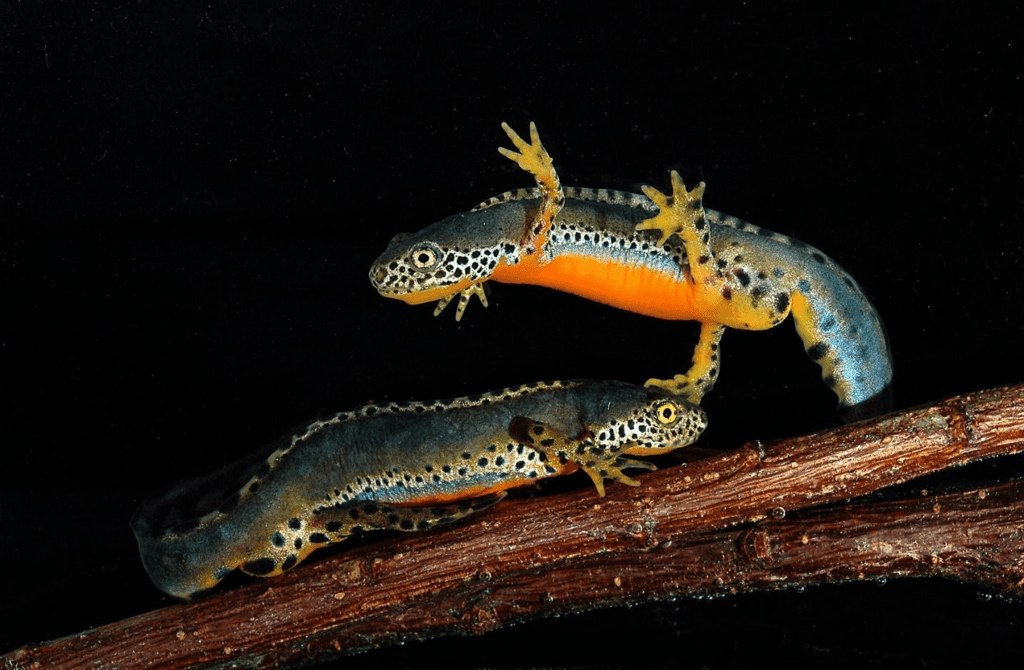
Picture 1. Image showing two newts interacting with each other (Wikipedia, 2022)
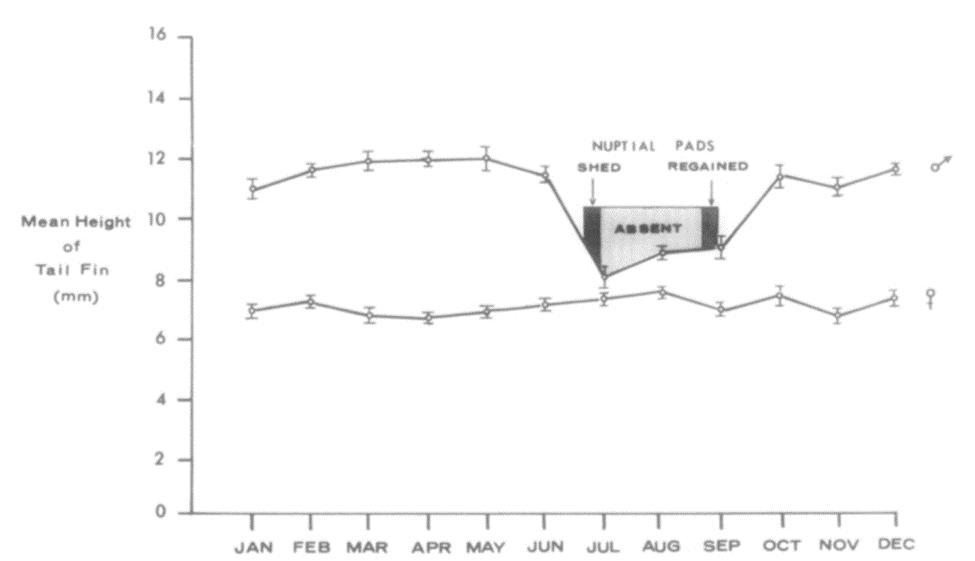
Figure 15 Mean newt tail height throughout the 12 months of the year, including periods where nuptial pads are lost and regained. Each point represents the mean, and the lengths of the vertical bars on those points represent the standard error of each mean (Singhas et al., 1975).
More specifically, 5 different groups of 12 male newts were placed in darkness, at temperatures of around 5, 10, 15, 20 and 25 degrees respectively. At weekly intervals, they were then brought into the light and room temperature long enough to measure their tail. Indeed, Results showed that, over 4 weeks at 5 degrees, there was roughly a 10% decline in tail height but at 20 and 25 degrees, the decline reached levels as high as 50%. At temperatures of 10 and 15 degrees the mean losses were intermediates between the two extremes (Singhas et al., 1975). However, the temperature itself cannot be responsible for these changes considering the major difference in the measurements found in this experiment for males and females and an involvement of hormonal factors is expected. In order to test for this likely possibility, newly collected male newts were then separated into different groups. In the first group, the pituitary gland was ectopically auto transplanted, a process which involves the seeding of healthy cells or tissues to a favorable ectopic location, and it was expected to elevate internal production of prolactin. In the second group, molting, the shedding of the exoskeleton, was inhibited by thyroidectomy, the removal of the thyroid gland. In others, mammalian prolactin and testosterone were directly administered (Singhas et al., 1975). The results of these procedures can be seen in the following table (Table 1).
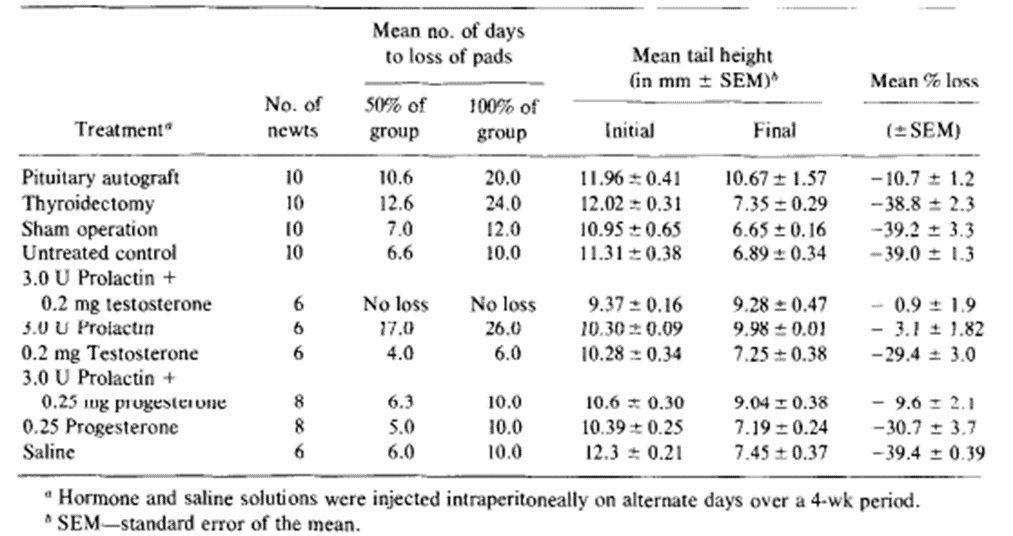
Table 1. The effects of pituitary gland autografting, thyroidectomy and mammalian hormones on the mean tail height and nuptial pads of male newts (Singhas et al., 1975).
It was witnessed that the tail fin did regress in autografted animals but not as rapidly as the control group. Furthermore, exogenous prolactin, that is to say prolactin originating from outside the organism, by itself and also in combination with testosterone counteracted almost entirely the expected effects of the tail fin’s laboratory conditioning. Regression of the tail fin after 4 weeks of testosterone treatment was marginally lower than that seen for control animals, but the result was too statistically insignificant (Singhas et al., 1975). Additionally, we can see that mammalian prolactin and testosterone are capable of restoring tail structures to their original condition which was observed when male newts with different hormone makeups had to be maintained in laboratory conditions for 4 weeks. From Figure 16, one can observe that tail heights for the control groups injected with saline would continue to decline during the experiment. It also shows that the tail heights of conditioned animals injected with testosterone also declined, albeit at a slightly slower rate, but those of animals treated with prolactin or prolactin combined with testosterone had their mean tail height increase sharply during the first 3 weeks, only slowing down as they approached the mean tail height of male newts in their normal breeding state. In many of the experiments, the mean tail height for animals given prolactin and testosterone would consistently surpass that of those that were only given prolactin. However, the differences between these two treatments showed differences that could not be considered significant with it only being one of 5%.

Figure 16. Mean percent changes in tail height over time for groups of laboratory-conditioned newts injected on alternate days with 3.0 U of prolactin (PL), 0.2 mg of testosterone (Test), 3.0 U of prolactin + 0.2 mg of testosterone (PL+TEST) or amphibian saline (Saline) (Singhas et al., 1975).
In short, the growth of the tail fin is mostly stimulated by prolactin. Thus, it would be expected to see higher levels of prolactin in the animal’s bloodstream during periods where the tail’s height is highest. We can also conclude that hormones like prolactin can have their effects increased when combined with other hormones which have a synergistic relationship (Singhas et al., 1975).
The Distinct Smell of the Skunk’s Tail
If there is one thing the skunk is known for, it would have to be its characteristic foul smell. Interestingly, this smell and the fluids that cause it are a result of a biochemical production that takes place around the animal’s tail. More specifically, the skunk has 2 glands near its tail, on each side of the anus, that secrete a mixture of sulfur-containing thiols which drive off predators. The gland’s secretions comprise three major thiols, three major thioacetates, and a methylquinoline. Only two of these thiols, (E)-2-butene-1-thiol and 3-methyl-1-butanethiol are responsible for the repellent odor, making up 51% and 70% of the secretions. The thioacetates, although not initially odiferous, can be converted into more potent thiols when encountering water. Indeed, water helps the thiols isolate and makes them resistant to chemical neutralization (Kerns. 2013). The seventh and final component is a much less reactive alkaloid 2-methylquinoline. The overall chemical composition and percentages of the components differ amongst different skunk species (Smallidge et al., 2021).
After an observation of the tail region, (Fig. 16), the location of the gland is quickly revealed to be below the animal’s muscular layer and inside the muscular envelope. Furthermore, unlike for most carnivores with a similar physiology, it occupies a limited portion of the receptacle’s surface. The follicles are sizable, red-brown colored, and have numerous 0.55 mm diameter oval cavities. The fluid reservoir is lined with dense laminated tissue and comprises elastic fibers. As for their product, the fluids are not acidic but neutral. Their vapours are very inflammable, but, when burned, release sulphur dioxide. It is easily dissolved in alcohol, ether, chloroform and concentrated sulphuric acid. However, it is practically insoluble in water (Thomas. 1896).

Figure 16. Representation of the location and shape of the skunk’s sacs and glands (Williams et al.)
Now for the smell itself, which is already clearly known to be foul, there is some interesting key information to understand: what is it that makes its smell so persistent, diffusible, and penetrating? The persistence can be linked to certain substances such as allyl sulphide and lower thiols. The diffusibility and penetrating characteristics are also a result of the thiols, whereas the permanence of the odor is attributable to a mixture of liquids which, when separated from the thiols, has a less penetrating smell, is comprised of nitrogen and a large amount of sulphur.
In short, the skunk’s fluid production serves as a unique and interesting example of evolutionarily developed defense mechanisms in animals. Seeing how each component of the secretion may contribute to the potency and staying power of its smell is endlessly fascinating.
Conclusion
To summarize, this paper has dissected how animal tails have naturally developed biochemical mechanisms and functionalities. The lizard tail has developed the capacity to detach and regenerate in a manner with unique qualities when compared to embryonic generation. It is able to perform autotomy due to the now understood paradox of its fracture plane-generating microstructures. It then regenerates on a timeline of approximately 60 days undergoing a succession of steps: wound healing, early growth of ependyma, outgrowth of vascularized tissue, differentiation of central cartilage tube, formation an ependymal core and finally continual tail lengthening and additional differentiation. The understanding of this timeline was also expanded on through the lenses of gene differentiation and skeletal development through an endochondral ossification-like process. The scorpion tail produces and when threatened injects a cocktail of toxins capable of interfering and even shutting down a body’s nervous system. Most exceptionally, a few animals have subsequently developed an innate immunity to the toxins of their scorpion prey. The channel responsible for transmitting pain signals in their central nervous system appears to have amino acid variants or acid residues that inhibit channel current for the duration of the venom exposure. Lastly, the skunk tail secretes a signature smell made particularly persistent by substances such as allyl sulphide and lower thiols. Tails can even be passively affected by chemical compounds, like in the case of the newt’s tail, whose height is controlled by hormone production. These various properties highlight the many unique adaptations that vertebrae have developed as they relate to tail function, along with the complexity of the chemical processes that accompany these adaptations.
Citations
Araújo, T. H., Faria, F. P. D., Katchburian, E., & Haapalainen, E. F. (2010). Ultrastructural changes in skeletal muscle of the tail of the lizard Hemidactylus mabouia immediately following autotomy. Acta Zoologica, 91(4), 440-446. https://doi-org.proxy3.library.mcgill.ca/10.1111/j.1463-6395.2009.00432.x
Baban, N. S., Orozaliev, A., Kirchhof, S., Stubbs, C. J., & Song, Y. A. (2022). Biomimetic fracture model of lizard tail autotomy. Science, 375(6582), 770-774. https://doi-org.proxy3.library.mcgill.ca/10.1126/science.abh1614
Boghozian, A., Nazem, H., Fazilati, M., Hejazi, S. H., & Sheikh Sajjadieh, M. (2021). Toxicity and protein composition of venoms of Hottentotta saulcyi, Hottentotta schach and Androctonus crassicauda, three scorpion species collected in Iran. Veterinary Medicine and Science, 7(6), 2418–2426. https://doi.org/10.1002/vms3.593
Breeland, G., Sinkler, M. A., & Menezes, R. G. (2021). Embryology, bone ossification. In StatPearls [Internet]. StatPearls Publishing. https://www.ncbi.nlm.nih.gov/books/NBK539718/
Charles A. Singhas, James Norman Dent; Hormonal control of the tail fin and of the nuptial pads in the male red-spotted newt. General and Comparative Endocrinology. Volume 26, Issue 3, 1975, Pages 382-393. ISSN 0016-6480. https://doi.org/10.1016/0016-6480(75)90092-1
Evans, E. R. J., Northfield, T. D., Daly, N. L., & Wilson, D. T. (2019). Venom Costs and Optimization in Scorpions. Frontiers in Ecology and Evolution, 7. https://doi.org/10.3389/fevo.2019.00196
Hopp, B. H., Arvidson, R. S., Adams, M. E., & Razak, K. A. (2017). Arizona bark scorpion venom resistance in the pallid bat, Antrozous pallidus. PLOS ONE, 12(8), e0183215. https://doi.org/10.1371/journal.pone.0183215
Hutchins, E. D., Markov, G. J., Eckalbar, W. L., George, R. M., King, J. M., Tokuyama, M. A., … & Kusumi, K. (2014). Transcriptomic analysis of tail regeneration in the lizard Anolis carolinensis reveals activation of conserved vertebrate developmental and repair mechanisms. PloS one, 9(8), e105004. https://doi.org/10.1371/journal.pone.0105004
Kripena, K. (2022, March 16). Scorpion Predators: What Eats Scorpions? INSECTCOP. https://insectcop.net/what-eats-scorpions/
Lozito, T. P., & Tuan, R. S. (2016). Lizard tail skeletal regeneration combines aspects of fracture healing and blastema-based regeneration. Development, 143(16), 2946-2957. https://doi.org/10.1242/dev.129585
Lozito, T. P., & Tuan, R. S. (2015). Lizard tail regeneration: regulation of two distinct cartilage regions by Indian hedgehog. Developmental Biology, 399(2), 249-262. https://doi.org/10.1016/j.ydbio.2014.12.036
Petricevich, V. L. (2010). Scorpion Venom and the Inflammatory Response. Mediators of Inflammation, 2010, 1–16. https://doi.org/10.1155/2010/903295
Rowe, A. H., Xiao, Y., Rowe, M. P., Cummins, T. R., & Zakon, H. H. (2013). Voltage-Gated Sodium Channel in Grasshopper Mice Defends Against Bark Scorpion Toxin. Science, 342(6157), 441–446. https://doi.org/10.1126/science.1236451
Rowe, M., & Rowe, A. (2013, October 24). Photograph of Bark Scorpion And Grasshopper Mouse. National Geographic. https://i.natgeofe.com/n/6cdd9ef5-8c02-4d60-a17c-afc9c8cbd31b/72754.jpg
Sanggaard, K. W., Danielsen, C. C., Wogensen, L., Vinding, M. S., Rydtoft, L. M., Mortensen, M. B., … & Enghild, J. J. (2012). Unique structural features facilitate lizard tail autotomy. Plos one, 7(12), e51803. https://doi.org/10.1371/journal.pone.0051803
Smallidge, S. T. (2021). New Mexico Skunks: Identification, Ecology, and Damage Management. College of Agricultural, Consumer and Environmental Sciences. https://www.dvm360.com/view/skunk-spray-toxicosis-odiferous-tale
Thomas B. Aldrich; A CHEMICAL STUDY OF THE SECRETION OF THE ANAL GLANDS OF MEPHITIS MEPHITIGA (COMMON SKUNK), WITH REMARKS ON THE PHYSIOLOGICAL PROPERTIES OF THIS SECRETION. J Exp Med 1 April (1896); 1 (2): 323–340. doi: https://doi-org.proxy3.library.mcgill.ca/10.1084/jem.1.2.323
Van der Meijden, A., & Kleinteich, T. (2016, December 28). Morphological image of scorpion telson. Onlinelibrary. https://doi.org/10.1111/joa.12582
Venomous versus poisonous. Same thing, right? Wrong! – Cabrillo National Monument (U.S. National Park Service). (n.d.). Retrieved October 31, 2022, from https://www.nps.gov/cabr/blogs/venomous-versus-poisonous-same-thing-right-wrong.htm
Williams K, Yuill C; Skunk Spray and Your Dog. VCA Animal Hospitals. https://vcahospitals.com/know-your-pet/skunk-spray-and-your-dog
Yigit, N., & Benli, M. (2008). The venom gland of the scorpion species Euscorpius mingrelicus (Scorpiones: Euscorpiidae): morphological and ultrastructural characterization. Journal of Venomous Animals and Toxins Including Tropical Diseases, 14(3). https://doi.org/10.1590/s1678-91992008000300007
Kerns, Nancy. (2013) Dog Got Skunked? DON’T Use Water (At Least, Not at First). Whole Dog Jounral. https://www.whole-dog-journal.com/blog/dog-got-skunked-dont-use-water-at-least-not-at-first/
Wikimedia Foundation. (2022) Newt, Wikipedia. https://en.wikipedia.org/wiki/Newt