Abstract
Coral polyps have been studied from many scientific standpoints, but this paper will examine coral polyps from a physical standpoint. To begin, polyps were analyzed under the lens of kinetics, relating the specific movement of polyps to the benefits of this movement. Through their natural ability to manipulate water flow using kinetics, polyps can enhance their growth, energy usage, and feeding. Large-scale hydrodynamics in coral reef ecosystems influence nutrient distribution, larval dispersal, and environmental conditions. At a smaller scale, the intricate flow patterns created by coral polyps’ tentacles play a crucial role in capturing suspended particles, highlighting the interplay between large and small-scale hydrodynamics in the survival and feeding mechanisms of coral polyps. Secondly, the mechanical properties of the coral skeletons were investigated emphasizing their vital role in coral survival when faced with external hydraulic forces. To be precise, this paper studies how crystal orientation and crystal size can contribute to the coral skeleton’s anisotropic properties. This holistic approach to studying corals, in addition to polyps, is crucial for understanding how these intricate marine ecosystems function. Lastly, when looked at from an optics perspective coral polyps have revealed the ability to manipulate light as well as to respond to its fluctuations. Coral polyps can act as light gradients and thus affect scattering, as well as change their size in response to certain stimuli indicating that the different types of light have an affect behavior. The insights made throughout this paper will offer an overall view of the fluidics, kinetics, mechanics, and optics relating to coral polyps.
Introduction
Coral polyps are intricate and refined sea creatures that have been, and will continue to be, studied from various scientific standpoints to explain many of their natural mysteries. A polyp is one of the two most basic forms of the phylum Cnidaria. Related to jellyfish (medusa) and sea anemones, polyps are tiny, soft-bodied organisms with an aboral side for attachment to a limestone skeleton called a calicle and an oral side with tentacles for feeding. They often get mistaken for rocks because of their hard and lifeless-looking shells. They also often get mistaken for plants because they are attached to the sea floor. However, polyps are related to neither, and unlike rocks, they are living; unlike plants, they are heterotrophs and have a symbiotic relationship with photosynthesizing zooxanthellae. Polyp calicles connect to one another and create colonies and as colonies grow for thousands of years, they join other colonies and create reefs (US Department of Commerce, National Oceanic and Atmospheric Administration, 2014). Some of the world’s coral reefs began to form more than 50 million years ago and coral polyps first appeared in the fossil record as solitary species more than 400 million years ago (Corals | National Geographic, 2011). Coral polyps produce their gametes by meiosis when conditions are favorable and the gametes are released into the water where external fertilization occurs. The zygote then drifts with the current and goes through mitosis where an embryo forms. A larva, called a planula, is then formed and it is able to maneuver and swim in the water using its cilia which allows it to look for a substrate to bind to. After settling onto a solid substrate, the planula undergoes metamorphosis transitioning from larva to polyp. The polyp then begins to form its skeletal cup and it will reproduce asexually to produce new polyps, increasing the size of the coral colony and the number of coral polyps. When the adult polyps reach sexual maturity, the life cycle will restart. Corals are made up of colonies of polyps that secrete calcium carbonate to create an exoskeleton. Over time as individual polyps within the colony continue to grow and reproduce, they collectively contribute to the growth of the coral’s skeleton (KSLOF Coral Reef Education: Free Coral Life Cycle Course, n.d.). This paper will go over and explain the key aspects relating to the fluidics, kinetics, mechanics, and optics of coral polyps.
Kinetics and Fluidics: The Interaction of Coral Polyps and Water
Hydrodynamics and Polyps
Overview
Many of the basic functions carried out by coral polyps depend on the movement of water since mature polyps have limited to no mobility. There are significant patterns of water circulation in regional areas around coral reefs, caused by large-scale processes. These patterns determine how water masses with varying properties are transported to the reef. This, in turn, affects the environmental conditions of the reef and influences the dispersal of reef organisms, including larvae. At the other end of the spectrum, there is turbulence characterized by motions with length scales similar to the Kolmogorov scale, which is the smallest scale in the turbulent flow of fluids. This has an impact on how particles are captured and the rate of mass transfer on the scale of individual coral polyp structures (Reidenbach et al., 2014).
Understanding examples of large-scale hydrodynamic processes is fundamental to comprehending how coral polyps interact with their environment. Ocean currents play a pivotal role in transporting nutrients, larvae, and heat across vast distances. These currents deliver essential nutrients to coral polyps, supporting their growth and sustenance (Davis et al., 2020). Furthermore, ocean currents facilitate the spread and colonization of new reef areas by transporting coral larvae. Tides also introduce daily fluctuations in water levels, creating dynamic conditions for coral polyps. Tidal influxes provide essential feeding opportunities for polyps: polyps extend their tentacles to capture plankton carried by the tide (Davis et al., 2020). Conversely, during low tide, the polyps may retract their tentacles to avoid exposure to air or strong sunlight. Tidal currents can also suspend sediment particles. Sedimentation is the settling of fine particles from the water onto the coral surface, which can smother and stress the polyps (Lenihan et al., 2015).
While large-scale hydrodynamics shape the broader reef environment, small-scale turbulence, and fluid dynamics govern the microcosm around individual coral polyps. The survival of these small organisms depends on the movement of water at the smallest scales. Normally, natural surfaces exposed to the forces of breaking waves and strong currents would gradually become smoother over time. However, hard corals actively grow intricate structures that create a rough and uneven surface. These structures are like obstacles that stir up the water and boost the movement of turbulence near the seafloor (Davis et al., 2020). Coral polyps have numerous flexible tentacles covered in tiny, specialized structures called cnidocytes. These tentacles extend into the surrounding water to capture tiny food particles. The movement of these tentacles creates local water currents around the polyp. As the tentacles sweep through the water, they create vortices and eddies that help bring food particles closer to the polyp for capture (Price & Patterson, 2023). Turbulence around coral polyps enhances the exchange of nutrients, dissolved gasses, and waste products with the surrounding water (Reidenbach et al., 2014). Turbulence also helps prevent excessive sedimentation on coral surfaces by creating local water currents, ensuring that sediment particles do not smother or stress the polyps (Lenihan et al., 2015).
Importance of Coral Polyp Tentacles for Small-Scale Hydrodynamic Feeding
The size, shape, and arrangement of the tentacles determine how they interact with the surrounding water. The movement and positioning of the tentacles create localized flow patterns in the water. For example, as water flows past and around the tentacles, it can be redirected, accelerated, or decelerated, creating intricate micro-flow patterns. The tentacles can then trap particles through mechanisms such as direct impaction (particles colliding with the tentacles), inertial impaction (particles redirected by the tentacles’ motion), and gravitational deposition (particles settling onto the tentacles). The micro-flow patterns generated by the tentacles increase the chances of particles coming into contact with the tentacle surfaces. This interaction is essential for the coral polyp to capture and feed on suspended particles in the water (Price & Patterson, 2023).
A study was conducted to investigate the significance of tentacle characteristics in scleractinian coral polyps regarding the capture of particles from the surrounding water. Researchers took morphometric measurements of tentacles from 27 coral species residing in the Caribbean Sea and used these measurements to construct model tentacles. A uniform flow of water was employed in a flume to observe the fluid dynamics around these models. The study benefits from using a larger-scale artificial representation because polyp tentacles are photosensitive and contract during daylight hours which complicates direct observation of their role in feeding. In addition, the concept of dynamic similarity states that if two physically similar objects have an equal Reynolds number (Re), the flow patterns around them will be identical. The Reynolds number is simply a dimensionless number used in fluid dynamics to predict the flow regime of a fluid around an object. It helps determine whether the flow is laminar (smooth and predictable) or turbulent (chaotic and unpredictable). It is calculated using the following formula (Price & Patterson, 2023):
Re (dimensionless)=(1/η)(s/{cm}^2 )×characteristic ~length (cm)×flow ~speed (cm/s)
For water, η = 0.01s/cm2
The characteristic length is determined as an object’s maximum length in the direction of the flow. In this study, the characteristic length was specifically designated as the length of the model tentacle (7 cm) due to the tentacle’s interaction with the surrounding water flow. Flow speed is the velocity of the fluid in the vicinity of the object. A flow speed of 5 cm/s was set in the flume. Plugging these values into the equation, the calculated Re value for the polyp tentacle model was 3500, suggesting turbulent flow (Price & Patterson, 2023). This model generates flow characteristics that closely resemble those of medium to long tentacles typically found in moderate to high-flow environments, as the calculated value falls within the Reynolds number range associated with the tentacles found in nature (Price & Patterson, 2023).
The flow of water over a reef is oscillatory and occurs in all directions, and as a result, tentacles that initially curve upstream during one phase of the oscillation subsequently curve downstream in the following phase. The model therefore represents the tentacles in three distinct positions (shown in Figure 1): straight, facing upstream, and facing downstream. To study the movement of particles around the model tentacles, the researchers utilized 1 mm thick black dyed bean sprout disks to mimic the particles in nature. A detailed examination was conducted, analyzing the trajectories of 10 bean sprout disks (particles) as they interacted with different vertical sections (upper, middle, base) of the three tentacle postures. This analysis encompassed both horizontal and vertical planes, spanning from the moment just before the particles encountered the tentacles to several tentacle diameters after the encounter (Price & Patterson, 2023).
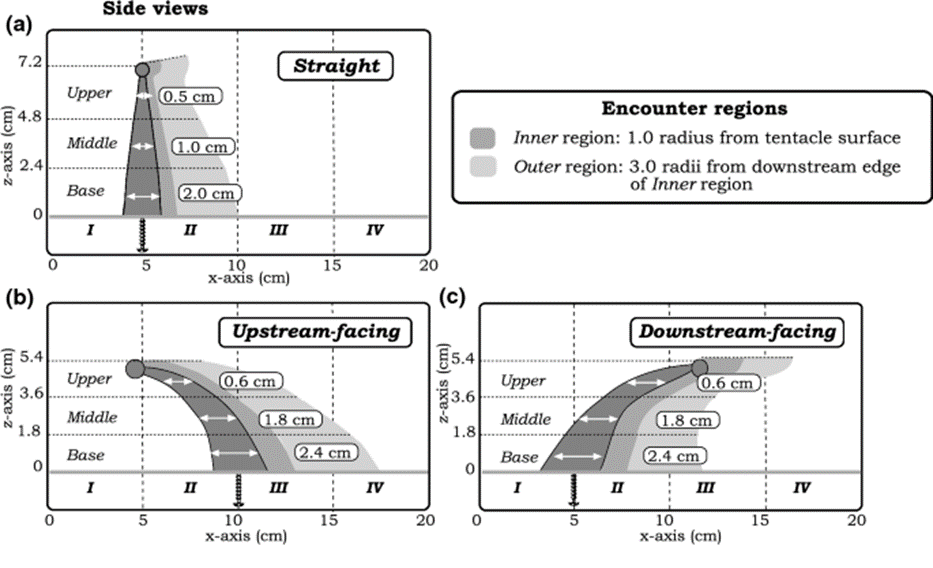
Fig. 1 Three postures of the coral tentacles (straight, upstream-facing, downstream-facing) shown with three vertical encounter zones (upper, middle, base), and two horizontal encounter zones (inner and outer region) (Price & Patterson, 2023).
An inner (dark grey) and outer (light grey) region are defined in the figure above. This is because the behaviour of particles in proximity to the tentacle plays a significant role in influencing the likelihood of their capture.
Vertical Deflection
The particles undergo distinct vertical deflections when they encounter the three separate orientations of the tentacle model with varying vertical zones (upper, middle, and base). Vertical deflection is quantifiable by calculating the difference between the inner region entry point, and the inner or outer region exit point on the z-axis. According to the results shown in Figure 2, all three vertical regions in the straight and upstream-facing tentacles deflect the particles downwards. The base zone of the downstream-facing tentacle effectively deflects particles upwards (Price & Patterson, 2023).
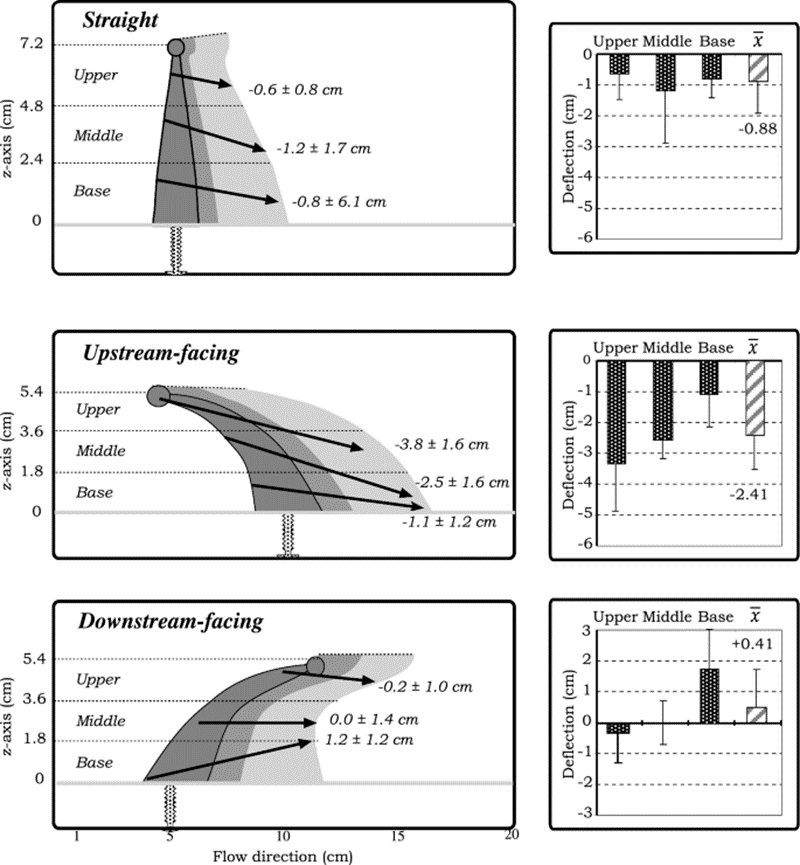
Fig. 2 Different tentacle postures causing the vertical deflection of particles across the horizontal regions. A positive number refers to an upward deflection, whereas a negative number refers to a downward deflection, while also including the standard deviations of the particles (Price & Patterson, 2023).
A feeding strategy that alters the microscale flow dynamics using varying orientations of the tentacles would be advantageous for many coral species according to these results. The downward deflection of particles by the straight tentacles increases the effective filtering height of the polyp because they can capture particles that are positioned higher in the water column above the coral. On the other hand, the upstream-facing tentacles can deflect particles towards the oral cavity of a simple polyp. The significant upward force created by the downstream-facing tentacles serves a dual purpose. Firstly, it helps in retaining particles within the zone where other tentacles can filter them, especially when multiple polyps are close together. Secondly, it plays a role in preventing the accumulation of particles below and between neighboring polyps (Price & Patterson, 2023).
Retention of Particles
In this study, the time particles spend within a specific region is known as the actual time ta. The encounter speed ve measures how quickly particles approach the tentacle. The researchers attempted to set this speed to 5 cm/s, but it ranged from 4.5-7.5 cm/s due to variations in the flume. To account for this possible variation in particle speed, the time each particle spent in a particular region is multiplied by the ratio of encounter speed to standardized speed vs of 5.0 cm/s (Price & Patterson, 2023).
t_t (s)=t_a (v_e/v_s )
When the physical model is not present, it is assumed that the particles would keep moving in a uniform direction at an adjusted freestream velocity. This allows the researchers to make comparisons and analyze the effects of the tentacle on particle behavior by contrasting it with how particles would move with no obstruction. As a result, the distance a particle covered horizontally would be associated with a theoretical freestream time ts. The retention value r is thus defined (Price & Patterson, 2023):
r (dimensionless)=(t_t (s))/(t_s (s))
If the r-value is less than 1, then this means that the particle was accelerated as it moved around the model. On the contrary, if the r-value is greater than 1, it does not necessarily equate to a decrease in particle speed. This is because the travel distance of a particle in a vortex could be much longer than the uniform freestream distance. A higher r-value means that the particle spends more time near the tentacle, which increases the likelihood of capture (Price & Patterson, 2023).
The retention of particles in the post-encounter regions was found for all three tentacle positions, most notably in region III found in Figure 3. The upstream-facing tentacle had the strongest retention in this region with a value of r = 2.5, whereas the straight and downstream-facing tentacles had values of r = 1.6 and 1.5 respectively. This decrease in retention is caused by the breakdown of the turbulent flows and structures created by the tentacle (Price & Patterson, 2023).
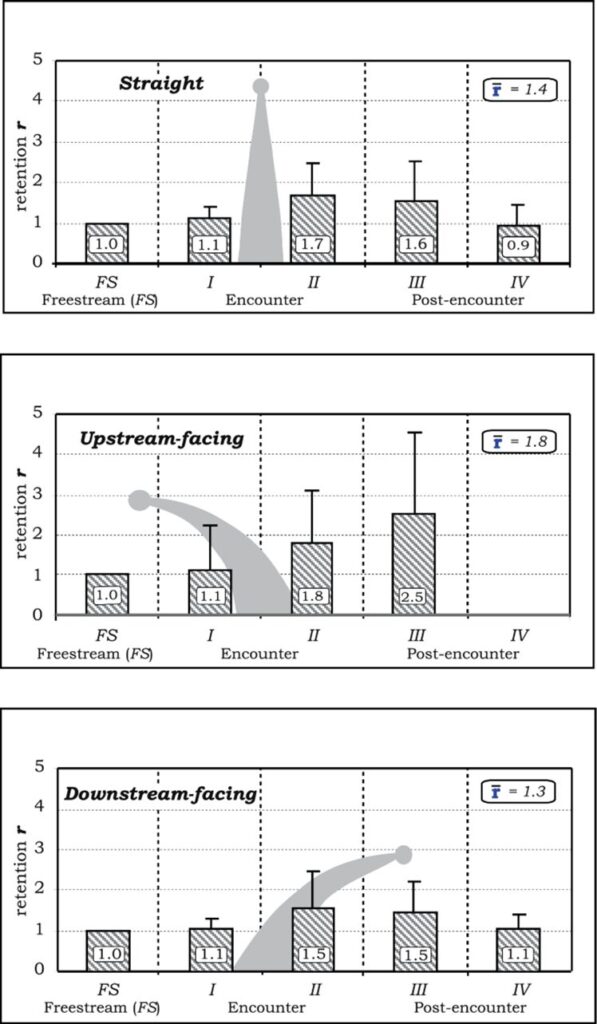
Fig. 3 Rentention r-values and standard deviations shown for particles in different horizontal sections, according to a specific tentacle posture (Price & Patterson, 2023).
The retention values observed are important to further understand particle settlement over oral surfaces. Instead of relying solely on direct impaction for feeding, the tentacles of the polyp can increase the time the particle spends around it. Leeward vortices can hold or keep the particle very close, providing additional opportunities for capture. It is also believed that some species contain mucus as a method of capturing particles over their oral surface. Increasing the time that the particles spend around the tentacles can allow for significant settlement (Price & Patterson, 2023).
The rotation of the particles caused by the tentacles also has implications with direct impaction feeding. When objects in fluid flow rotate or tumble, it effectively makes them seem large by extending the object beyond its freestream path, increasing the chances of them encountering other feeding elements (Price & Patterson, 2023).
Given that water movement is essential for the healthy growth of coral, it is highly probable that corals have developed these specific behaviors and physical characteristics to regulate the flow of water at different levels within their structure. These findings suggest that the secondary radial symmetry of tentacles in simpler coral polyps is an optimal strategy for filtering suspended particles, especially in dynamic and multidirectional water flow environments. This adaptation allows them to ensure optimal access to vital nutrients and other factors required for their growth and survival in their aquatic environment.
The Effect of Polyp Pulsation on Hydrodynamics
Overview
Being rooted to the bottom of the ocean floor, corals are very limited in their ability to benefit from physical movement as a means to feed and grow. However, a unique characteristic exhibited by the specific design of soft corals belonging to the Xeniidae family is the perpetual, rhythmic, non-synchronous, and vigorous pulsation of its coral polyps. The benefit of these kinetic pulsations can be explained by analyzing the flow of water directly next to the polyps as well as an analysis of the mitigation of water recirculation caused by neighboring polyps. The kinetic mechanism of pulsations used by a coral polyp is a way in which coral polyps interact with water and this mechanism will be explained through the analysis of a study of four different Heteroxenia fuscescens (Xeniidae family soft corals) colonies at a depth of 5 to 10 metres (Kremien et al., 2013). On a general note, from the information of these 4 colonies, it was observed that the polyps would pulsate more than 95% of the time and there would be short (15-20 minute) intervals without pulsation which can be considered rest. It was also recorded that resting intervals occurred when the intensity of solar radiation was less than 50% of the daily maximum which again highlights the connection between these pulsations and feeding as the pulsation activity has a dependence on the efficiency of photosynthesis (Kremien et al., 2013).
The Mechanism of Pulsations
To start, the specific design allowing these pulsations will be explained. Throughout the pulsatory phase, the tentacles of the actively engaged polyps consistently maintain extension, and their tentacles exhibit a rhythmic alteration in orientation, transitioning from a fully extended configuration in the open state (Fig. 4 A) to a tightly compacted configuration in the closed state (Fig. 4 B). It is also essential to observe the distinct and specific tentacle arrangements among the pulsating polyps, indicating a lack of synchronized movement among colony polyps. Each polyp acts independently of one another but there is still an overall positive impact on the colony as a whole. During pulsations, the average exposed surface area to incident downward light was 2.69 times larger compared to that observed during the closed state (Kremien et al., 2013).
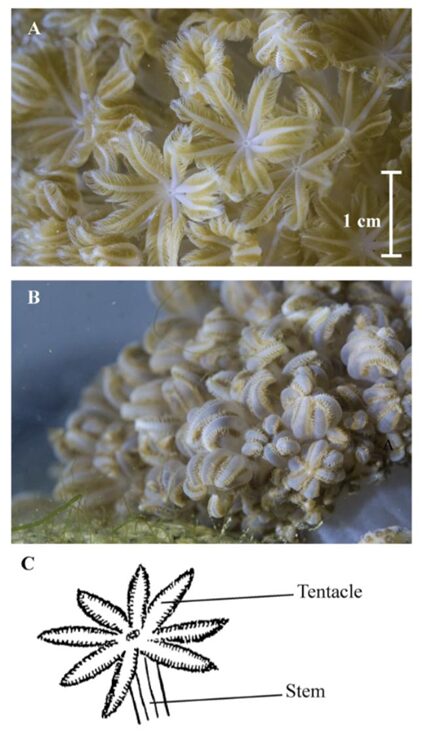
Fig. 4 The Xeniid coral Heteroxenia fuscescens exhibiting pulsation (A) and a resting state (B). A diagram (C) provides a visual representation of a single polyp’s stem and tentacles (Kremien et al., 2013).
The Effect of Pulsations on Water Flow and Nutrient Filtration
As a result of this pulsation mechanism, there are hydrodynamic effects on the flow field that is generated by the pulsations. Due to the vigorous and upward nature of these pulsations, there was a noticeable difference in the vertical flow which was caused by the vertical thrust of the coral polyp. The vertical velocity at a height of 1.8 cm above the coral was measured to be about 2 times greater during pulsating periods (Fig. 5). On the other hand, the horizontal flow was not altered as much as the vertical flow (Fig. 6) since the pulsations are mostly an upward action and therefore do not affect the horizontal flow as much (Kremien et al., 2013).
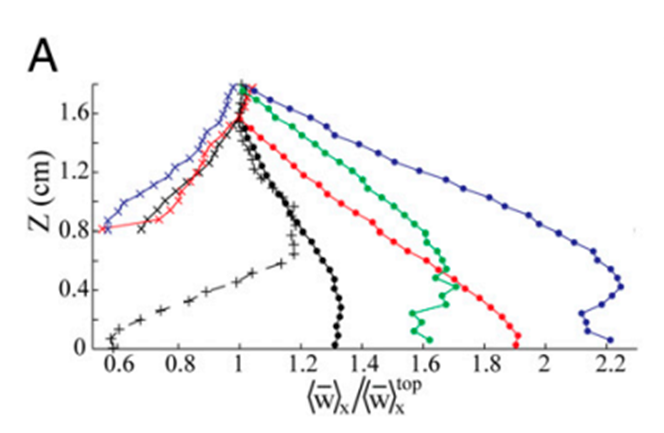
Fig. 5 Graph depicting the normalized vertical mean velocity components (x-axis) measured 0-1.8 cm above the polyp. Extended and pulsating (represented by full circles), contracted and inactive (indicated by × symbols), extended but inactive (denoted by + symbols). The vertical axis (Z) is defined with Z = 0 at the uppermost tip of the visible polyp (Kremien et al., 2013).
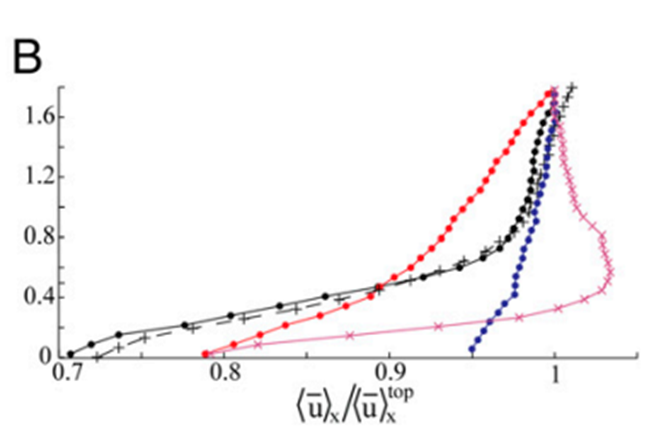
Fig. 6 Graph depicting the normalized horizontal mean velocity components (x-axis) measured 0-1.8 cm above the polyp. Extended and pulsating (represented by full circles), contracted and inactive (indicated by × symbols), extended but inactive (denoted by + symbols). The vertical axis (Z) is defined with Z = 0 at the uppermost tip of the visible polyp (Kremien et al., 2013).
However, this ever so slightly increased horizontal flow of water effectively prevents water refiltration by removing post-filtered water away from neighboring polyps and the colony. Lagrangian simulations (a type of computational modeling where individual particles are tracked as they move through a fluid or dynamic environment) can be used to track this process of the removal of postfiltered water. Results from a Lagrangian simulation show that this pulsation process substantially reduced the probability of water refiltration in polyp colonies. In corals with resting polyps, greater than 50% of the particles were refiltered by a neighboring polyp (Fig. 7 B, 7 D) whereas less than 20% of the particles were refiltered by a neighboring polyp in corals with pulsating polyps (Fig. 7 A, 7 C) (Kremien et al., 2013). This reduction in re-filtration enhances both the absorption of dissolved substances from the water and the removal of excreted waste materials. This is particularly beneficial for colonial organisms like corals, where all polyps collectively share resources. By reducing re-filtration, corals can efficiently distribute vital nutrients and optimize the health and growth of the entire colony, contributing to their overall resilience and vitality.
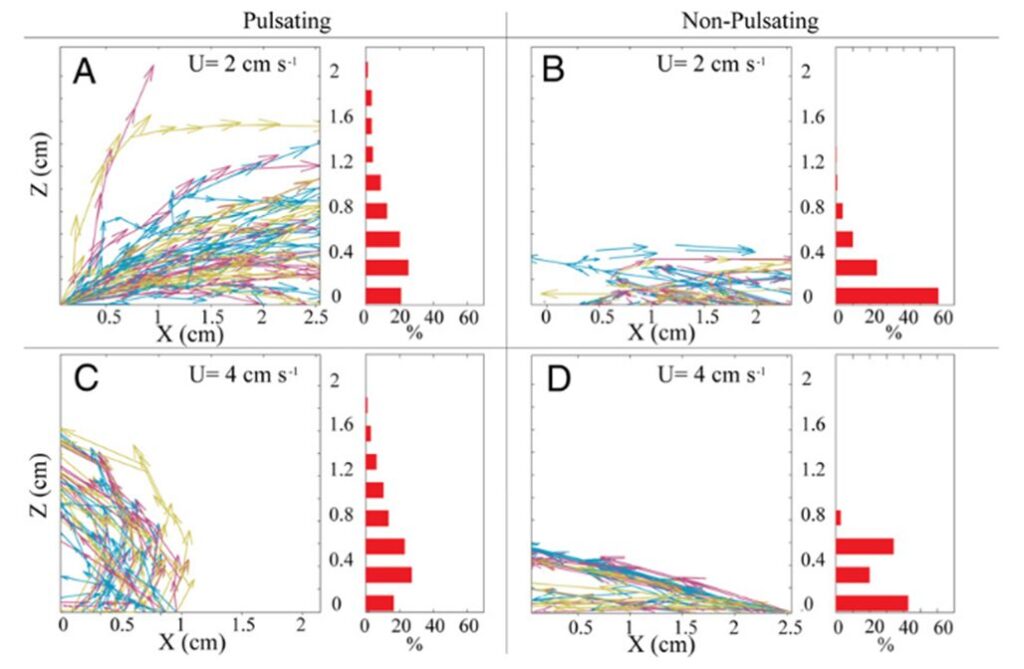
Fig. 7 Trajectories followed by hypothetical waterborne particles originating from the coral surface. The left portion of the panel displays approximately 50 typical trajectories. The right part of the panel consists of histograms indicating the proportion of particles (among all trajectories) departing the measurement area at varying elevations above the coral. “U” denotes the ambient horizontal velocity (Kremien et al., 2013).
While the influence of water flow on the uptake of dissolved and particulate substances in corals has been extensively studied, the added benefit of reduced re-filtration appears to be a unique feature of pulsating corals. Being immobile and attached to the ocean floor, corals have a limited means of feeding and thus must resort to feeding using external factors such as water flow. To overcome this problem, some coral polyps (specifically those of the Xeniidae family) have gone through evolutionary processes that allow the coral polyps to pulsate. This specific design of the Xeniidae coral family that grants the coral polyps the ability to pulsate allows for an enhanced control over the water flow near the coral allowing the corals to benefit from these pulsating movements of their polyps. Furthermore, the impact of pulsations in the presence of a current needs to be determined. Strong currents may perform a similar function to pulsations, potentially making pulsations unnecessary and redundant. An examination of turbulence intensity at the coral-water boundary layer can address this question. An experiment compared pulsating coral polyps in a 3 cm/s ambient current with resting coral in an 8 cm/s strong current (Fig. 8), which exceeds the typical current velocity experienced by corals by nearly threefold (Kremien et al., 2013).
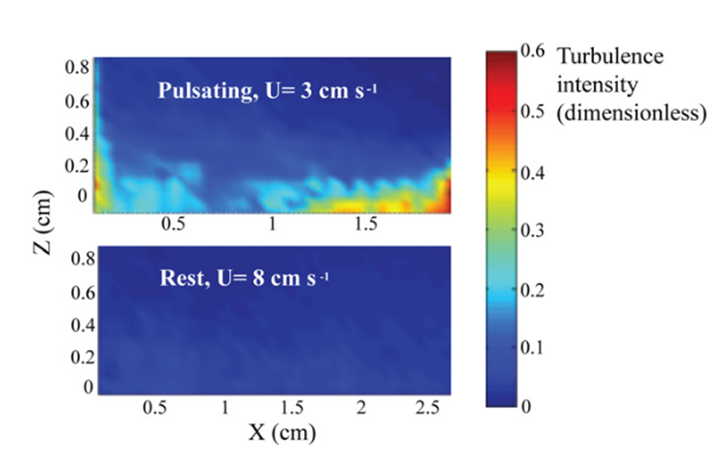
Fig. 8 Turbulence intensity (color bar) at the coral-water boundary layer (Kremien et al., 2013).
As seen in Figure 8, the findings illustrate the pronounced impact of pulsation, where the turbulence intensity is observed to be at least an order of magnitude more potent when pulsations are present. This allows polyps to overcome their dependence on an external factor such as the natural flow of water when it comes to feeding. Instead of relying on the external and natural flow of water to filter nutrients, certain polyps are designed in a way that allows them to filter nutrients through pulsations which are essentially independent of the natural water flow as seen in Figure 8.
Another element related to the pulsation of a polyp is the change in surface area between the pulsating state and the resting state. The surface area of a coral polyp that was exposed to light during pulsations was 2.69 times larger (Fig. 4 A) than the surface area exposed when the polyps were resting (Fig. 4 B). Similarly, photosynthesis by zooxanthellae was also 2.68 times higher during pulsating periods than when the polyp was at rest. The kinetic pulsations and movements of polyps also have a large effect on the photosynthetic output and chemical processes of corals. This connection between photosynthetic efficiency and polyp pulsations is a way in which corals enhance their feeding in nutrient-deficient environments and will be further developed in the second essay relating to the chemical analysis of polyps (Kremien et al., 2013).
Mechanical Adaptations of Coral Skeletons: Anisotropic Properties and Factors Contributing to their Formation
Overview
Coral polyps are exposed to a variety of physical threats while living at the bottom of the sea. These physical threats can be waves, currents, and other hydraulic forces (Chamberlain, 1978). Coral polyps’ soft tissue bodies are vulnerable to these hydraulic forces. In order to protect themselves, they create an exoskeleton through biomineralization. This process involves specialized cells within the coral polyp that combine calcium ions (Ca²⁺) and carbonate ions (CO₃²⁻) from the seawater to form calcium carbonate (CaCO₃) crystals. Then, these crystals are transported to the surface of the polyps and are secreted as a thin layer of calcium carbonate. This layer becomes part of the growing exoskeleton. Over time, as individual polyps within the colony continue to grow and reproduce, they collectively contribute to the growth of the colony’s exoskeleton. This layer-by-layer growth process is what forms the sturdy and protective skeletal structure of the coral colony which serves as the foundation for coral reefs (Drake et al., 2019).
Anisotropic Properties
The coral skeleton has evolved to best withstand mechanical loads. Its anisotropic qualities can provide it with a structural advantage (Moynihan et al., 2022). Anisotropy is described as the characteristic of having properties that vary when measured along different axes or directions (Britannica, n.d.). Precisely, the microstructures of the coral skeleton are arranged to make it stronger in the direction parallel to corallite (exoskeleton of individual polyps) growth. This direction is also where it is more likely to face higher forces as illustrated in Fig.9 (Moynihan et al., 2022). This has been experimentally measured through multiple studies. Notably, using in-depth microindentation on WLT Porites coral samples, it was revealed that both Young’s modulus (a measure of material stiffness) and hardness (a measure of material resistance to deformation) varied significantly between surfaces. In particular, Young’s modulus and hardness were higher in surface 1 (Fig.10). This surface corresponds to the primary vertical growth direction of the coral. (Moynihan et al., 2022).
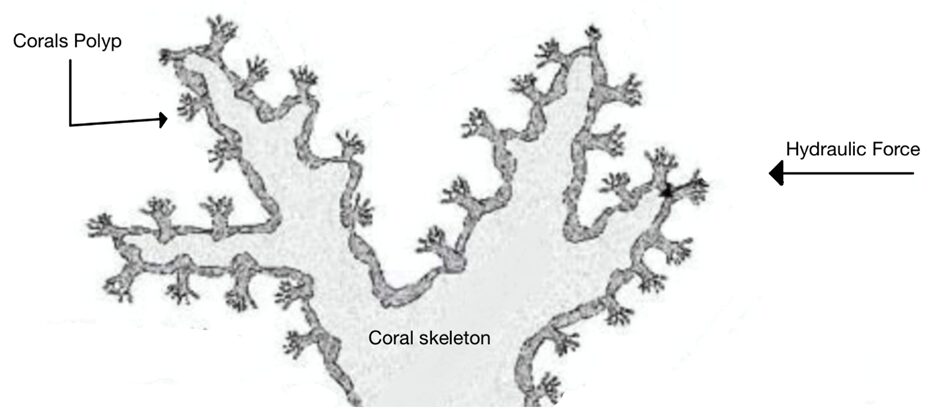
Fig. 9 Cross section of a Live Stony Coral. Polyp and corallite growth is shown to be perpendicular to the coral skeleton and mainly parallel to the hydraulic forces (Adapted from About Corals, Anemones, and Their Kin, n.d.).
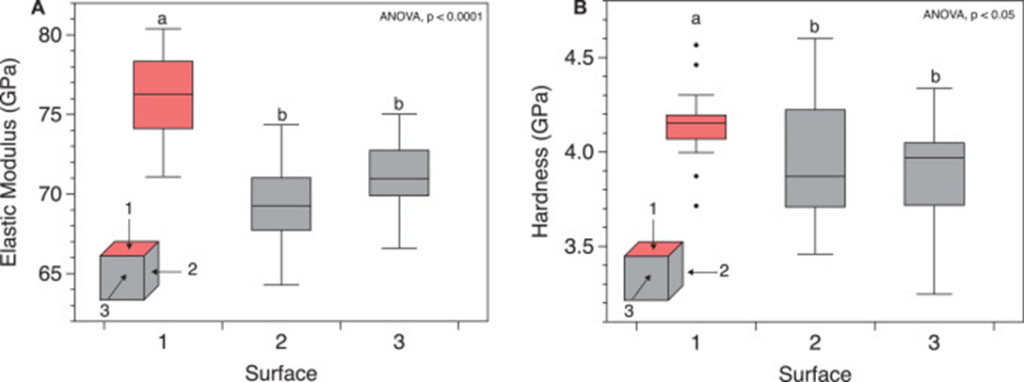
Fig. 10 Micromechanical anisotropy in WLT sample. (A) Young’s modulus (GPa) and (B) hardness (GPa) measured using depth-sensing microindentation in surfaces 1 (coral growth direction), 2, and 3 of the WLT Porites sample. Statistically different groupings are indicated by a and b (Moynihan et al., 2022).
Factors Contributing to Anisotropic Properties
The anisotropic properties of coral skeletons are a product of how calcium carbonate crystals are arranged and oriented as the skeletons develop. Specifically, at the microscale, slight differences in the preferred crystal orientation and grain size can explain the coral’s skeleton’s anisotropic properties (Moynihan et al., 2022).
At the microscale level, the orientation of calcium carbonate crystals is a main contributor to the anisotropic properties of the coral skeleton. A material’s mechanical properties become direction-dependent when the crystals are pre-eminently aligned along specific axes. If a material’s crystals are oriented to align with the direction of an applied force, it will display a higher resistance to deformation and greater strength (Liu et al., 2020). It has been shown that the individual crystals tend to orient themselves parallel to the direction of growth of the corallites (Fig.11). Since corallites’ growth is parallel to the direction of the hydraulic forces, the coral skeleton is ultimately better equipped to resist the environmental forces (Moynihan et al., 2022).
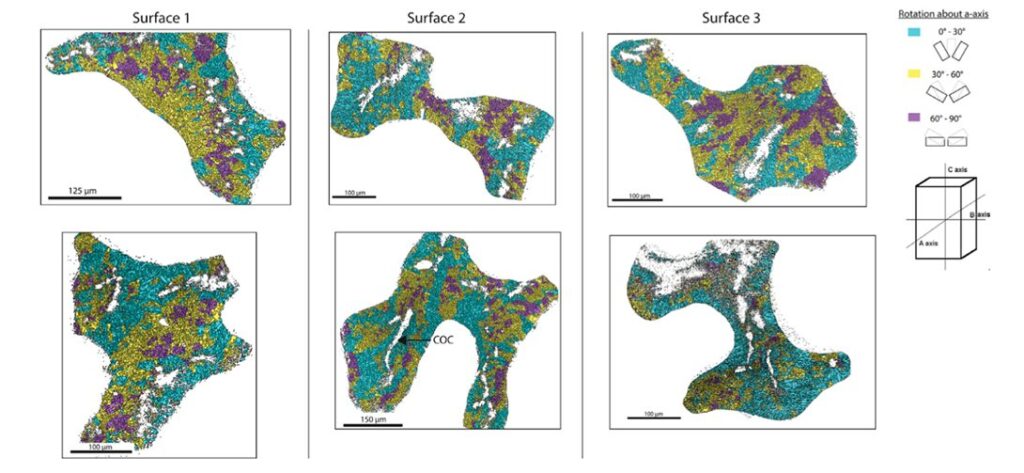
Fig. 11 Trichomic mapping of crystal orientation data from electron backscatter diffraction (EBSD) analysis of surfaces 1 – 3 of the WLT Porites sample. Two locations within each surface are displayed in columns. Crystallographic directions were clustered based on their orientation about the a-axis (cyan = 0 – 30◦; yellow = 30 – 60◦; magenta = 60 – 90◦). Centers of calcification (COCs) are non-indexed regions surrounded by indexed crystals, and an example of it is demarcated in Surface 2 (Moynihan et al., 2022).
The anisotropic properties of the coral skeleton are also attributed to the varying size of grains (crystals). The term “grains” refers to the individual crystalline structures that compose the calcium carbonate framework of the skeleton. Smaller grains cause more dislocations (defects in a crystal that affect a material’s property) and grain boundaries (interface between grains), resulting in greater ductility and deformability. This increased density of grain boundaries offers more pathways for dislocations to navigate through the material. In contrast, larger grains can contribute to higher strength and stiffness (Brush Wellman, 2010). These differences in grain size can manifest as anisotropy in the material’s response to mechanical loads. Surface 1 (direction of the growth of corallites) was experimentally shown to have significantly higher grain size than surfaces 2 or 3 (Fig. 12) (Moynihan et al., 2022).
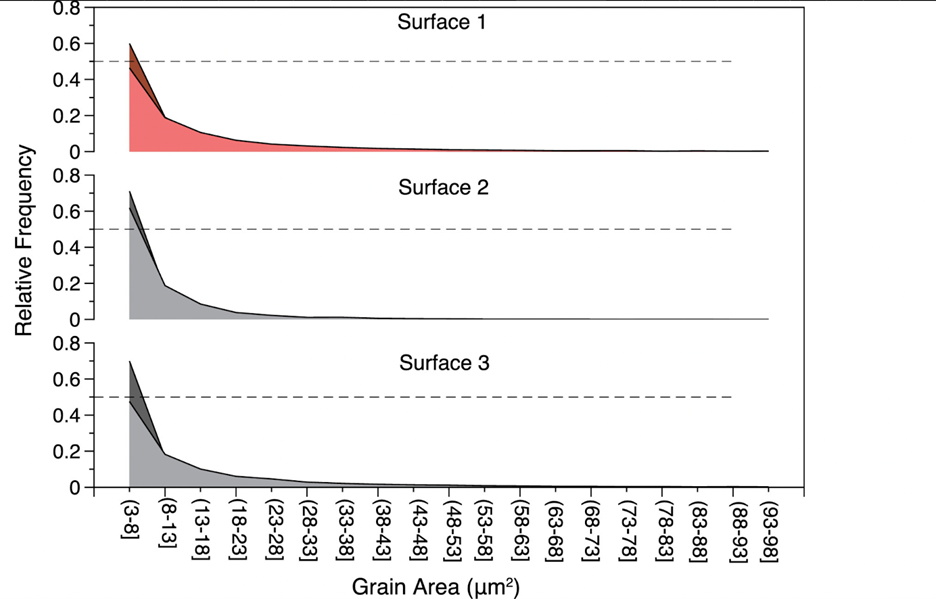
Fig. 12 Grain size (µm) relative frequency distribution from surfaces 1, 2, and 3 of the WLT Porites sample. Grain size was determined from electron backscatter diffraction (EBSD) data. All three surfaces had significantly different grain size distributions, with surface 1 having the largest average grain size (Moynihan et al., 2022).
To recap, coral polyps are soft-bodied organisms, and they can build themselves an exoskeleton through biomineralization. The exoskeleton needs to find a way to adequately protect the polyp and maintain the structure of the coral reef. Its solution is to orient calcium carbonate crystals parallel to the direction of corallite growth and, to vary the size of the crystals. This solution allows the exoskeleton to illustrate anisotropic properties. Precisely, it exhibits higher Young’s modulus and hardness in the direction parallel to corallite growth. Since the exoskeleton undergoes hydraulic forces in the direction parallel to growth, the exoskeleton can ensure the protection of the coral polyps and can serve as the foundation of the coral reef.
Optics: The Manipulation of Light by Coral Polyps
Overview
Corals are reef-building organisms and hence are in need of extra nutrients and energy sources to maintain their massive colonies. This is achieved through the photosynthetic cells, called zooxanthellae, which have a mutualistic relationship with coral polyps. Coral polyps are usually clear structures, but the Zooxanthellae growing on its body provides coloration. This relationship is very important as up to 90% of the organically synthesized material through photosynthesis by zooxanthellae is effectively transferred to the host coral tissue. This photosynthetic phenomenon serves as the primary driving force propelling the expansion and fecundity of coral reefs. On the other hand, corals live in low-nutrient tropical environments, are exposed to many different arrays of light depending on the time of day and year, and are subject to lower levels of light intensity than land plants as they live underwater. To overcome these problems, corals have developed specific features such as a mutualistic relationship between polyps and zooxanthellae as well as the unique design of zooxanthellae that allows them to manipulate light and enhance photosynthesis. The coral polyps produce carbon dioxide and water through cellular respiration and the zooxanthellae use the carbon dioxide and water to carry out photosynthesis. The symbiotic relationship between zooxanthellae and coral polyps enables an efficient nutrient-recycling process within oligotrophic tropical waters (US Department of Commerce, n.d.). Corals are heavily reliant on the symbiont production of energy and must therefore be good absorbers of light that enables photosynthesis. Thus, corals display several optical properties and phenomena.
Monte Carlo Simulations
The Monte Carlo Simulations are a series of experiments and models aimed to model the photon propagation in coral tissue to reveal its scattering properties. These scattering properties entail the scattering coefficient which quantifies the probability of light being scattered, the absorption coefficient which quantifies the probability of light absorption, and the reduced scattering coefficient which shows the light scattering in a scattering-rich medium which forces the photon to move down its concentration gradient. In these experiments, Daniel Wangpraseurt et al. (2016) worked on solving the reverse of the radiative transfer equation to find the optical properties of coral polyps. They performed these experiments on the Faviid corals and found an estimate of the optical properties of these corals. Their results are shown in Fig. 11. It was assumed that the incident laser light beam had scattering coefficients of 0.06, 3.2, and 17.8 cm-1 and the absorption coefficient was found to be µa = 1.8 cm-1 and the scattering coefficient to be µs=10 cm-1 (Fig. 13).
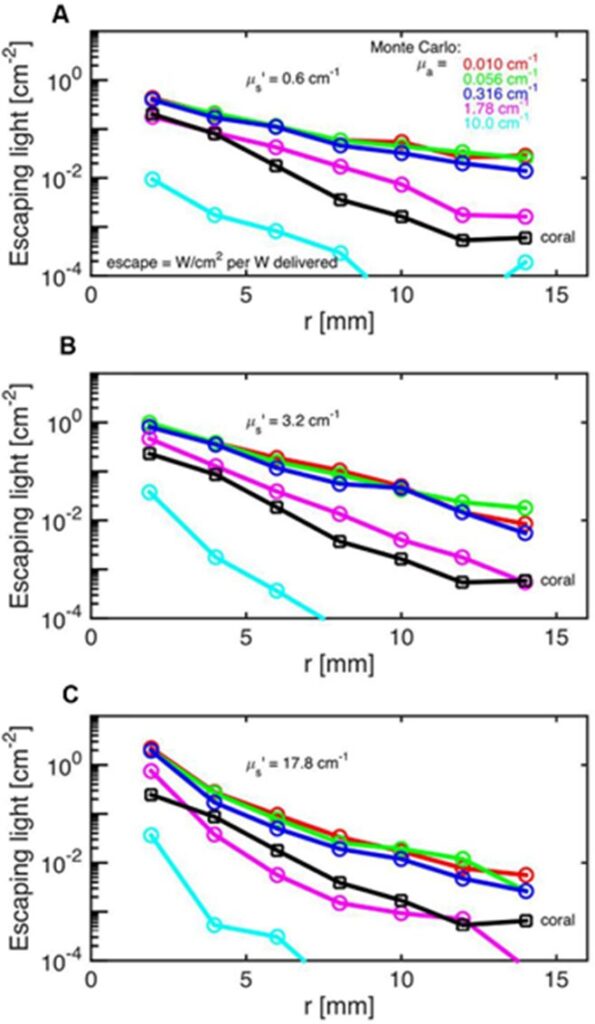
Fig. 13 Monte Carlo simulations to estimate coral tissue optical properties. Escaping light (ϕ) as a function of radial distance (r) from incident laser light beam as measured over intact coral tissue surface. Monte Carlo simulations were run for 5 μa values, assuming μs’ = 0.6 (A), 3.2 (B), and 17.8 cm-1 (C). The experimental data are black square (mean ± SD, n = 13 repetitions). The match between the experiment and simulation was based on both the slope and absolute values of the ϕ(r) curves. The best choice was μa = 1.8 cm-1 and μs’ = 10 cm-1
In addition, they showed that the fluence rate at a wavelength of 636 nm during midday under vertical sunlight can reach up to twice the incident irradiance at a depth of 50-100 µm (Wangpraseurt et al., 2016).
Polyps as Light Gradients
In another study by Wangpraseurt et al. (2012) used scalar irradiance microprobes to characterize the light field and light penetration at the level of the coral tissue. Their results showed that scalar irradiance levels on the surface of the polyp reached about 200% of the downwelling photon irradiance, which is mainly due to multiple scattering of the tissue. In addition, they showed that scalar irradiance levels were consistently higher over polyp tissue rather than coenosarc tissue (the living tissue surrounding the coral skeleton) for photosynthetically active radiation (PAR) (400-700 nm) and near-infrared radiation (NIR) (700-800 nm) where scalar irradiance levels were 1.3 to 1.4 times higher over polyp tissue (Fig. 14).
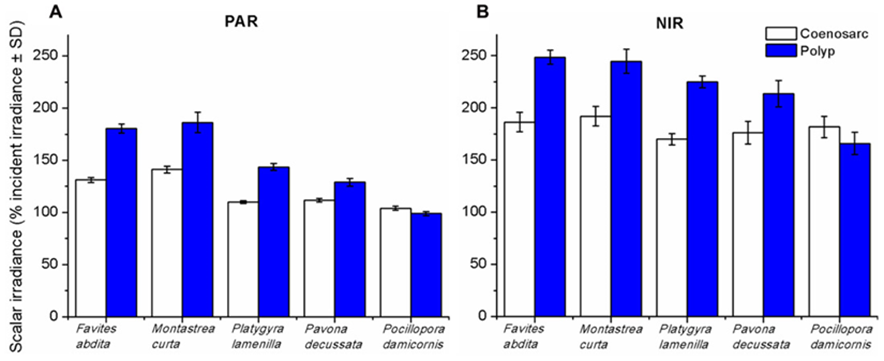
Fig. 14 Integrated scalar irradiance (in % of downwelling irradiance) at the tissue surface of corals(A) PAR (photosynthetically available radiation, 400–700 nm) and (B) NIR (near-infrared radiation, 700–800 nm). Data are means ± SD (n = 9). Measurements were done at the surface of the coenosarc (white bars) and polyp (blue bars) tissue, respectively (Wangpraseurt et al., 2012).
Moreover, micro profiles revealed the presence of strong optical gradients: detailed horizontal micro measurements revealed heterogeneity in irradiance levels, and further measurements showed that irradiance levels dropped to 74% and even 16% of downwelling irradiance levels at the coenosarc cells layers. That drop was determined by the thickness of the polyp tissue where the thicker the tissue was, the more the irradiance levels decreased with respect to the downwelling irradiance (Fig 15).
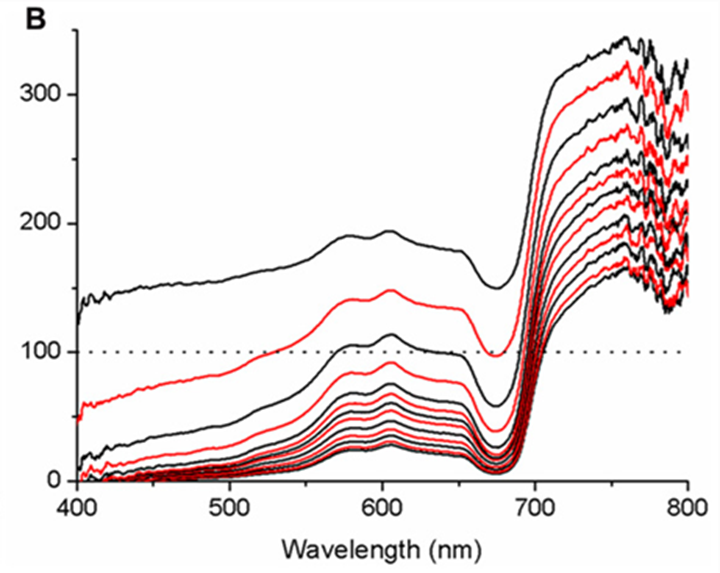
Fig. 15 Micro profiles of spectral scalar irradiance within coral tissue. Representative profiles were measured in the coral Montastrea curta within (A) coenosarc (tissue thickness ~400 μm) and (B) polyp tissue (tissue thickness ~1200 μm). Scalar irradiance was normalized to the incident downwelling spectral irradiance, Ed; the dotted line represents 100% Ed. The uppermost spectrum (black) represents measurements taken at the coral surface and subsequent spectra correspond to increments of 100 μm with the lowermost spectrum equaling measurements over the coral skeleton. Spectra are colored in an alternating fashion (black–red) for clarity (Wangpraseurt et al., 2012).
Thus, these results show the existence of an optical gradient in the polyp tissue, as well as proving that the polyp scatters light laterally and vertically along its body. Then, polyps have a clear spatial stratification where the lower layer cells have more light-limiting conditions due to the scattering, photon trapping, and mismatch of the refractive indices of the water and surface layer of polyp cells with water. (Wangpraseurt et al., 2012). In this way, the polyp can maximize the algal exposure to light and amplify photosynthesis and its products.
Implications of the Nature of the Polyp Tissue
Due to this heavy reliance on photosynthesis, polyps must utilize all the light that falls upon them. Thus, as an evolutionary mechanism, coral polyps scatter light in a way to decrease self-shading and increase the algal exposure to light, in addition to forming a gradient of light and scattering it laterally and vertically to the shaded parts of the coral body. This is achieved by a translucent lamina on the polyps that enhances the polyp tissue’s ability to scatter, absorb as well as reflect light. Consequently, coral polyps can absorb 85% more solar radiation, with two orders of magnitude less pigment density than terrain plants. It was found that the zooxanthellae on the coral polyps absorb about 2 to 5 times more light than individual algae, showing the coral polyps’ ability to manipulate light (Enríquez et al., 2005).
Fluorescent proteins
The coral Polyps contain a special type of proteins called fluorescent protein that play a role in the optical properties of the polyp. These fluorescent proteins (FPs) can occur in four main colors, of which three are fluorescent (cyan, green, and red) and one that is non-fluorescent (blue or purple). These fluorescent proteins function in the process of photoluminescence. They will act as photoprotection where they will absorb harmful UV light and re-emit it as red-shifted nonharmful light as well as act as a scatterer of light spreading irradiance to low light regions of the polyp body.
In corals, the electrons in the chromophores become excited when exposed to blue-green light with wavelengths between 450 nm and 495 nm. They then release this absorbed energy by emitting light in the form of fluorescence, which has a longer wavelength and falls within the red-shifted range of 495 nm to 570 nm.
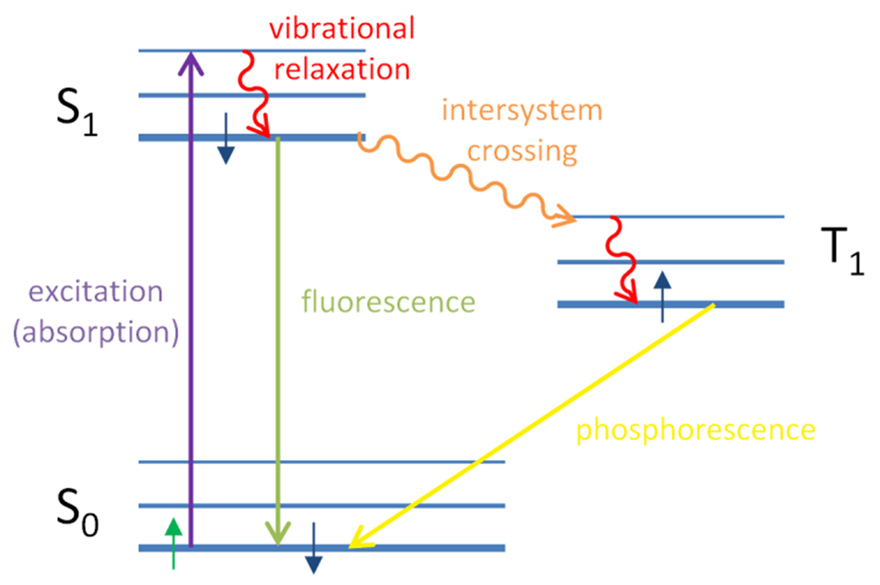
Fig 16. Jablonski diagram explaining the occurrence of fluorescence and phosphorescence. In fluorescence, the electron gets excited by the absorption of a photon. The electron then returns to its ground state by emitting a photon of lower energy where some energy was due to vibration and relaxation (Eck, 2014).
Hyperspectral fluorescence and reflectance imaging revealed that fluorescent proteins were distributed in granular with the highest density in the polyp tissue. In fact, the hyperspectral reflectance in visible light of the highly fluorescent protein of polyp tissue was 5.5 times higher in polyp tissue than in coenosarc tissue, and the highly fluorescent protein was about 75% higher than that of tissues with normal fluorescence (Lyndby et al., n.d.).
The thermal action spectrum of surface heating vs. spectral irradiance revealed that heating was directly proportional to the wavelength, meaning that the shorter wavelength led to less heating, where the heating was the highest in the red (largest wavelength) and lowest in the blue. Figure 15 shows the variation of temperature as a function of fluorescent protein fluorescence. The graph clearly illustrates a positive relation between the two. Proving that the FPs regulate the heat and irradiance of the polyp surface where higher FP concentrations lead to higher temperatures (Lyndby et al., n.d.).
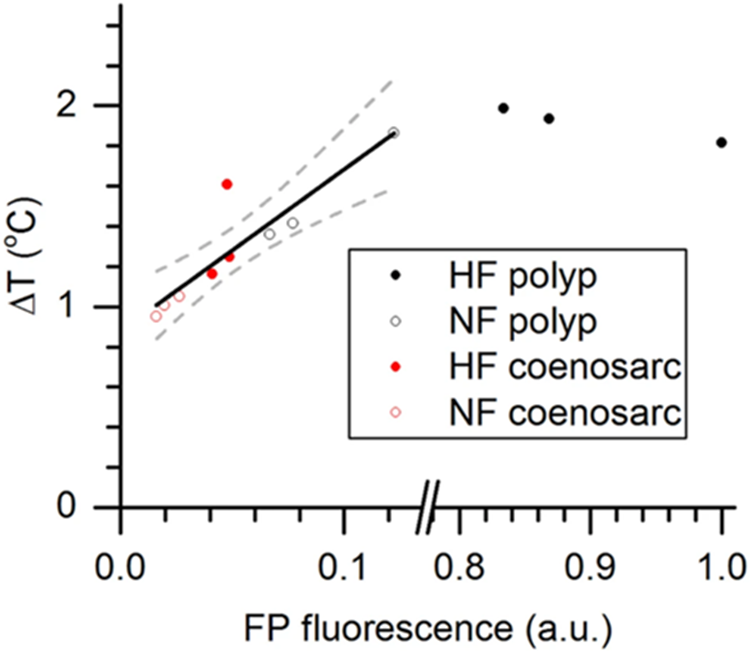
Fig. 17 Relationship between green fluorescence and coral tissue surface heating. Coral tissue surface heating is expressed as ΔT (°C), i.e., the difference between coral surface temperature and the temperature in the ambient water, and was integrated for excitation between 400–700 nm (PAR) and expressed for a total irradiance delivery of 500 W m−2. FP fluorescence (a.u.) was normalized to the maximally measured fluorescence (=1). The black line shows the positive correlation between heating and green fluorescence (R2 = 0.89). Dashed lines are 95% confidence intervals (Lyndby et al., n.d.).
Moreover, fluorescent proteins are also involved in decreasing photoinhibition which is the reduction of the photosynthetic ability of algae due to exposure to high light. As seen in Fig 13, FPs are photoprotective where the polyps associated with fluorescent proteins had lower photoinhibition levels than the ones associated with non-fluorescent ones.
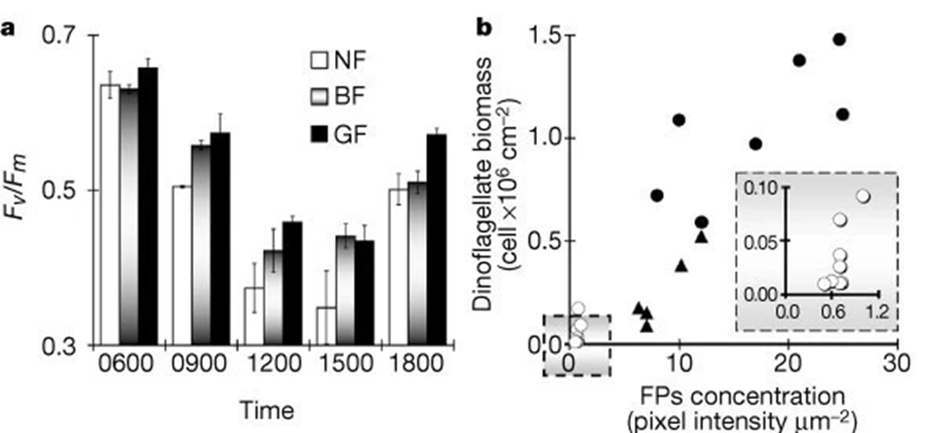
Fig. 18 Photoinhibition and bleaching responses of corals. a. Maximal potential quantum yield (Fv / Fm) of dinoflagellates in green highly fluorescent (GF), brown medium fluorescent (BF), and non-fluorescent (NF) Acropora palifera. Results are means ± s.e. for 3 sub-colonies × morph × 2 tanks × time interval (that is, the samples (270) taken from 3 colonies for each of the 3 morphs, divided randomly between 2 tanks, and killed for PAM fluorescence measurement at 5 given times). b. Dinoflagellates per cm2 and relative concentration of fluorescent pigments per µm2 of sampled corals: open circles, bleached; triangles, part-bleached; filled circles; unbleached. Inset. Enlarged section of graph marked in square (Salih et al., 2000).
Furthermore, it was found that scalar irradiance and temperature were enhanced in areas in polyp tissue where highly fluorescent protein was present. However, these results were not consistent at high irradiance, and this is theorized to be due to the high scattering of the fluorescent proteins. This strong scattering of light decreases the light penetration, cooling the coral down and reducing the temperature levels. Effectively, the fluorescent proteins, found in the polyp tissue, are responsible for regulating irradiance and temperature levels of the coral (Salih et al., 2000). Hence, fluorescent proteins act as a sunscreen for the polyp protecting it from high irradiance levels and temperatures through emission and scattering.
Coral Bleaching is a phenomenon that has long threatened the survival of corals. Coral bleaching is the process that leads to the demise of where the coral host and the symbiont algae dissociate: the coral expels its symbiont algae, or the algae loses its photosynthetic pigments. This interrupts the symbiosis between them, leading to the death of both the coral and the algae. Bleaching can be triggered by several factors: extreme temperatures that are either too cold or too hot, high irradiance, prolonged darkness, and several others. Corals are usually exposed to a different array of temperatures and irradiances and light exposure. Thus, to prevent corals from coral bleaching the polyps must have an effective mechanism of light acclimating and adaptation to the different light and temperature conditions. Therefore, the coral utilizes the properties of its fluorescent proteins to adapt to the changing conditions surrounding them. These fluorescent proteins regulate the temperature, reduce photoinhibition, and regulate the irradiance levels at the level of the polyp tissue.
Light Induced Contraction or Expansion
Observations by Salih et al. (2000) noted that coral polyps actively vary the areal density of pigment chromatophores by contraction and expansion. Under high light, the polyps contract, making the pigment-dense layer of FPs that effectively acts as a sunscreen to protect the polyp from high irradiance, while in low light environment, the coral expands, allowing more light to interact with the coral tissue, leading to an increased absorption of light (Wangpraseurt et al., 2019). Most reef coral polyps extend their tentacles at night and retract them by dawn, but any mechanical or light stimulation may cause the polyps to contract. In fact, the contraction and expansion of the coral polyps is a combination of three factors: water flow, light, and availability of prey. It was found that the two main deciding factors were water flow and light intensity levels. Wangpraseurt et al. (2019) defined a compensation intensity, which is calculated from coral respiration and photosynthesis. If the intensity of light was greater than the compensation intensity (Icom), the coral polyp would not expand but remain contracted; however, when the light intensity is lower than the compensation intensity, the expansion would depend on water flow and availability of prey (Fig. 13). This shows that the intensity of light plays a role in determining the state of the polyp.
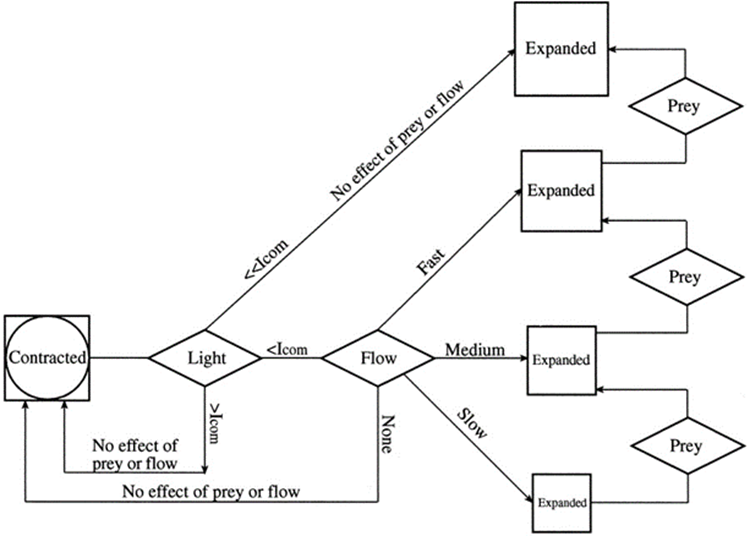
Fig. 19 Conceptual diagram of tentacle expansion behavior in the stony coral Favia favus in response to different levels of light intensity, flow speed, and the addition of prey (Artemia nauplii). At low irradiance, under the compensation point (Icom), corals will expand with correlation to the flow rate. At high irradiance level, above Icom the tentacles remain contracted regardless of the presence of neither flow nor prey. In still water the corals remain contracted (Levy et al., 2001).
Therefore, in response to varying intensities of light, the polyp will expand or contract increasing and decreasing the density of fluorescent proteins, hence either increasing absorption under low irradiance or forming a protective layer under high-intensity light.
Blue Light Photoreceptors:
As mentioned previously, coral polyps can expand and contract depending on the ambient light: it is expanded in the dark phase when there is low light intensity and irradiance, and it is contracted in the photo phase, when there is high intensity and irradiance. It was noticed that lunar radiation also influenced this motion of polyps, and it was found that the coral polyps had a very low threshold of photoreception sensitivity of a range of 1.2 * 1015 quanta m-2 s-1 to 2.5* 1015 quanta m-2 s-1, which matches the photon flux density levels of moonlight (Gorbunov & Falkowski, 2002).
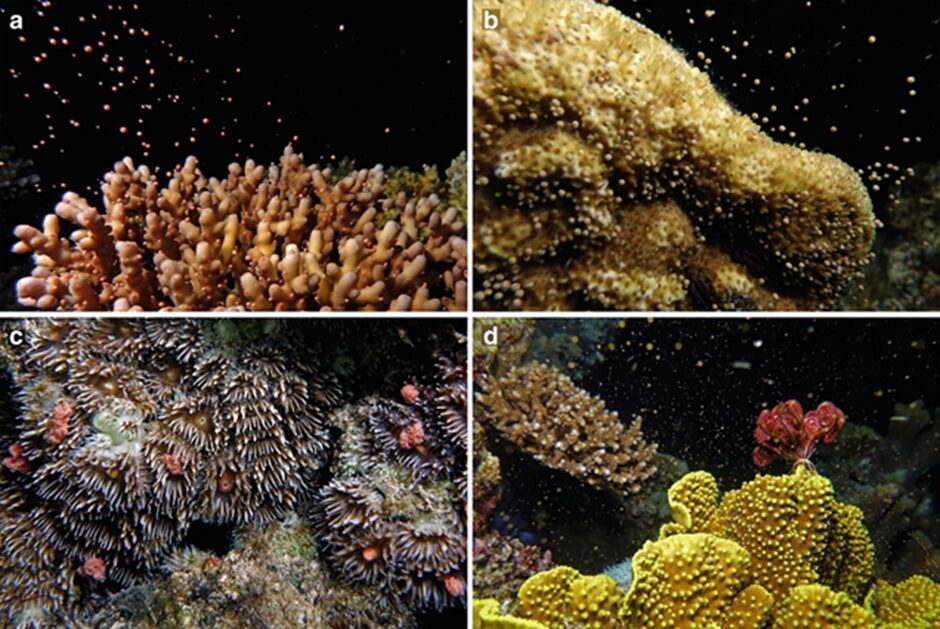
Fig. 20 Image showing synchronous ejection of the coral polyps’ gametes into the water.
It was found that full moonlight stimulated minute contraction in the coral polyps. Figure 21 shows detailed measurements of the moonlight and its corresponding scattering signals in coral polyps (Gorbunov & Falkowski, 2002). These minute contractions are believed to cause the ejection of the gametes causing synchronous reproduction witnessed in corals. Therefore, the polyp can absorb light and differentiate between the different intensities and types of light (sun or moon) and utilize these aspects to trigger different reactions in the polyp to ensure the survival and continuation of its species.
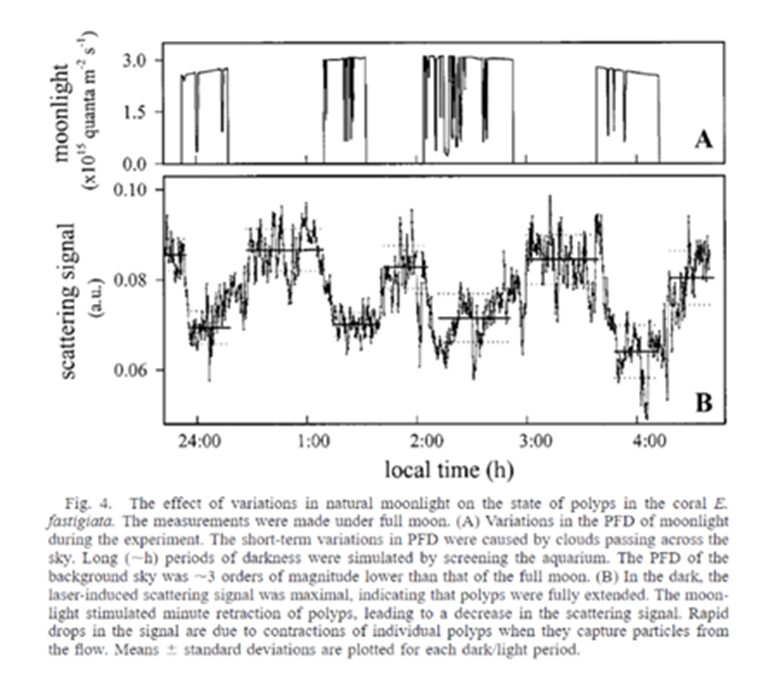
Fig. 21 E. fastigiata. The measurements were made under full moon. (A) Variations in the PFD of moonlight during the experiment. The short-term variations in PFD were caused by clouds passing across the sky. Long (~h) periods of darkness were stimulated by screening the aquarium. The PFD of the background sky was ~3 magnitudes lower than that of the full moon. (B) In the dark, the laser-induced scattering signal was maximal, indicating that polyps were fully extended. The moonlight stimulated minute retraction of polyps, leading to a decrease in the scattering signal. Rapid drops in the signal are due to contractions of the individual polyps when they capture particles from the flow. Mean ± standard deviations are plotted for each dark/light period (Gorbunov & Falkowski, 2002).
Therefore, this explains the mysterious process of polyp reproduction. Since polyps are immobile, the only way to do so is by ejecting their gametes into the water and relying on external fertilization. It is fascinating to note that the polyps, male and female, with no form of communication, release their oocytes and sperms synchronously (Fig. 20). This process occurs 7 to 10 nights after a full moon, the specific period determined by the type of coral.
Conclusion
In conclusion, coral polyps make use of many intricate mechanical, optical, kinetic, and fluidic mechanisms to overcome problems that impede their survival and enhance their multitude of functions in the marine ecosystem. One of the main obstacles that limit coral polyps is their lack of mobility on the ocean floor. In addition, the flow of the surrounding water is dynamic and multidirectional. To respond to these environmental limitations, polyps have evolved specific strategies to maximize their nutrient intake. One design solution focuses on enhancing the particle filtration efficiency of simple coral polyps. Despite the dynamic and multidirectional water flow environments, the orientation of polyp tentacles can vertically deflect particles, and increase particle retention time to improve nutrient intake. Furthermore, the specific design of some polyp tentacles allows for the polyps to pulsate which alters the fluidics of the water in close proximity. This altering of the flow of water allows for polyps to overcome their sedentary nature and reliance on the natural flow of water. This transformation occurs because the polyp’s pulsations allow corals to generate a significant, self-sustaining, and independent water current. This allows polyps to have increased control over their environment which enhances their feeding and growth. Next, to ensure the protection of the polyp and to serve as the foundation of the coral reef, the coral skeleton needs to find a way to optimize its resistance to external hydraulic forces such as waves and currents. The coral skeleton creates anisotropic properties by orienting its crystals parallel to the direction of growth. Additionally, it creates larger crystals on the surface perpendicular to the direction of growth. Together, they contribute to a higher Young’s modulus and hardness in the direction parallel to the growth of corallite. This is also the direction in which the coral skeleton undergoes most of the hydraulic forces. Thus, its anisotropic properties enhance the coral’s ability to withstand external hydraulic forces. Lastly, polyps face the challenge of limited sunlight availability at the ocean’s bottom which hinders their access to sufficient light for photosynthesis and energy production. Therefore, polyps need to find a way to maximize their light absorption. To optimize photosynthesis, polyp tissue has a distinct refractive index compared to water, features a translucent lamina, and employs fluorescent proteins that scatter and reflect light while reducing self-shading. Furthermore, polyps survive in a very delicate environment where extreme temperatures and irradiances can lead to their demise. Their solution is to utilize fluorescent proteins to regulate irradiance and temperature levels. They also actively expand and contract in response to incident light. They expand in low-light conditions to maximize light absorption and contract in high-light conditions to protect themselves. Moreover, polyps cannot communicate. To reproduce, they use blue-light photoreceptors that detect irradiance from the moon which signals them to secrete their gametes. Thus, what may seem like an immobile plant is an organism that operates on the most delicate of scales to survive and thrive in its environment. When analyzed from a physics standpoint, these minuscule sea plants grant valuable observations in kinetics, optics, mechanics, and fluidics. The complexity of such a small natural system highlights the need to preserve these biodiverse beings and ecosystems in the face of ongoing environmental threats. Coral polyps highlight the many complex ideas and even mysteries present in the natural world.
References
About corals, anemones, and their kin. (n.d.). Web.augsburg.edu. https://web.augsburg.edu/~capman/aquaria/SpecialTopicsFiles/AboutCoralsAnemonesAndKin.html
Britannica. (n.d.). Anisotropy | Definition, Examples, & Facts | Britannica. Www.britannica.com. https://www.britannica.com/science/anisotropy#:~:text=anisotropy%2C%20in%20physics%2C%20the%20quality
Brush Wellman. (2010). Grain Size and Material Strength. https://materion.com/-/media/files/alloy/newsletters/technical-tidbits/issue-no-15—grain-size-and-material-strength.pdf
Chamberlain, J. A. (1978). Mechanical properties of coral skeleton: compressive strength and its adaptive significance. Paleobiology, 4(4), 419–435. https://doi.org/10.1017/s0094837300006163
Corals | National Geographic. (2011, June 10). Animals. https://www.nationalgeographic.com/animals/invertebrates/facts/corals-1#:~:text=Coral%20polyps%20are%20tiny%2C%20soft
Davis, K., Pawlak, G., & Monismith, S. (2020). Turbulence and Coral Reefs. https://doi.org/10.1146/annurev-marine-042120-
Drake, J. L., Mass, T., Stolarski, J., Von Euw, S., van de Schootbrugge, B., & Falkowski, P. G. (2019). How corals made rocks through the ages. Global Change Biology, 26(1), 31–53. https://doi.org/10.1111/gcb.14912
Enríquez, S., Méndez, E. R., & Prieto, R. I. (2005). Multiple scattering on coral skeletons enhances light absorption by symbiotic algae. Limnology and Oceanography, 50(4), 1025–1032. https://doi.org/10.4319/lo.2005.50.4.1025
France, I.-I. de B. S. – G. /. (2023, February 13). fluorescent proteins. IBS – Institut de Biologie Structurale – Grenoble / France. https://www.ibs.fr/fr/recherche/assemblage-dynamique-et-reactivite/groupe-imagerie-integree-de-la-reponse-au-stress/equipe-pixel/techniques-utilisees/photophysics-of-fluorescent-proteins?lang=fr
Gorbunov, M. Y., & Falkowski, P. G. (2002). Photoreceptors in the cnidarian hosts allow symbiotic corals to sense blue moonlight. Limnology and Oceanography, 47(1), 309–315. https://doi.org/10.4319/lo.2002.47.1.0309
Guillemin, G., Patat, J. L., Fournie, J., & Chetail, M. (1987). The use of coral as a bone graft substitute. Journal of biomedical materials research, 21(5), 557–567. https://doi.org/10.1002/jbm.820210503
Kernan, M. J. (2007). Mechanotransduction and auditory transduction in Drosophila. Pflügers Archiv – European Journal of Physiology, 454(5), 703–720. https://doi.org/10.1007/s00424-007-0263-x
Kremien, M., Shavit, U., Mass, T., & Genin, A. (2013). Benefit of pulsation in soft corals. Proceedings of the National Academy of Sciences, 110(22), 8978–8983. https://doi.org/10.1073/pnas.1301826110
KSLOF Coral Reef Education: Free Coral Life Cycle Course. (n.d.). Living Oceans Foundation. https://www.livingoceansfoundation.org/education/portal/course/life-cycle/#:~:text=CORAL%20CYCLEs
Lenihan, H. S., Hench, J. L., Holbrook, S. J., Schmitt, R. J., & Potoski, M. (2015). Hydrodynamics influence coral performance through simultaneous direct and indirect effects. Ecology, 96(6), 1540–1549. https://doi.org/10.1890/14-1115.1
Levy, O., Mizrahi, L., E, C. N., & Achituv, Y. (2001). Factors Controlling the Expansion Behavior of Favia favus (Cnidaria: Scleractinia): Effects of Light, Flow, and Planktonic Prey. The Biological Bulletin, 200(2), 118126. https://doi.org/10.2307/1543305
Liu, Z., Zhang, Z., & Ritchie, R. O. (2020). Structural Orientation and Anisotropy in Biological Materials: Functional Designs and Mechanics. Advanced Functional Materials, 30(10). https://doi.org/10.1002/adfm.201908121
Lyndby, N. H., Kühl, M., & Wangpraseurt, D. (2016). Heat generation and light scattering of green fluorescent protein-like pigments in coral tissue. Scientific Reports, 6(1). https://doi.org/10.1038/srep26599
Moynihan, M. A., Amini, S., Oalmann, J., Chua, J. Q. I., Tanzil, J. T. I., Fan, T. Y., Miserez, A., & Goodkin, N. F. (2022). Crystal orientation mapping and microindentation reveal anisotropy in Porites skeletons. Acta Biomaterialia, 151, 446–456. https://doi.org/10.1016/j.actbio.2022.08.012
Price, Wm. S., & Patterson, M. R. (2023). Microscale flow dynamics and particle capture in scleractinian corals: I. Role of the tentacles. Coral Reefs, 42(3), 761–783. https://doi.org/10.1007/s00338-023-02385-5
Reidenbach, M., Koseff, J., & Yahel, G. (2014). Boundary Layer Turbulence and Flow Structure over a Fringing Coral Reef RIME -restoration of Israeli micro estuaries View project Revisiting the role of dissolved organic matter, the largest pool of exchangeable carbon in the ocean, as a nutritional source for aquatic metazoans View project. https://doi.org/10.2307/3841037
Risalat, B. (2022, April 20). Symbiotic Relationship Between Coral And Zooxanthellae. Reefcraze.com. https://reefcraze.com/relationship-between-coral-and-zooxanthellae/
Salih, A., Larkum, A., Cox, G., Kühl, M., & Hoegh-Guldberg, O. (2000). Fluorescent pigments in corals are photoprotective. Nature, 408(6814), Article 6814. https://doi.org/10.1038/35048564Wangpraseurt, D., Jacques, S. L., Petrie, T., & Kühl, M. (2016). Monte Carlo Modeling of Photon Propagation Reveals Highly Scattering Coral Tissue. Frontiers in Plant Science, 7. https://www.frontiersin.org/articles/10.3389/fpls.2016.01404
Samson, J. E., Miller, L. A., Ray, D., Holzman, R., Shavit, U., & Khatri, S. (2019). A novel mechanism of mixing by pulsing corals. J Exp Biol, 222(15), jeb192518. https://doi.org/10.1242/jeb.192518
US Department of Commerce, National Oceanic and Atmospheric Administration. (2014). NOAA CoRIS – What are Coral Reefs. Noaa.gov. https://www.coris.noaa.gov/about/what_are/
US Department of Commerce, N. O. and A. A. (n.d.-a). Corals: NOAA’s National Ocean Service Education. Oceanservice.noaa.gov. https://oceanservice.noaa.gov/education/tutorial_corals/media/supp_coral02bc.html
US Department of Commerce, N. O. and A. A. (n.d.-b). Zooxanthellae…What’s That – Corals: NOAA’s National Ocean Service Education. Oceanservice.noaa.gov. https://oceanservice.noaa.gov/education/tutorial_corals/coral02_zooxanthellae.html#:~:text=Coral%20polyps%2C%20which%20are%20animals Wangpraseurt, D., Jacques, S., Lyndby, N., Holm, J. B., Pages, C. F., & Kühl, M. (2019). Microscale light management and inherent optical properties of intact corals studied with optical coherence tomography. Journal of The Royal Society Interface, 16(151), 20180567. https://doi.org/10.1098/rsif.2018.0567