Abstract
There are many microorganisms in the universe, and they can generally be sorted into three types: prokaryotic, eukaryotic, and acellular. The subject of this paper is a member of the eukaryotic family, and they are commonly present in nature: the diatom. The diatomite which is formed by the death of diatoms has a complex miniature geometric structure. The microstructure of diatomite is mainly composed of diatom’s exoskeleton structure which is composed of siloxite tetrahedron. It possesses a special porous structure that it uses to adsorb particles or bacteria from 0.1 to 1.0 μm. As a result, they are widely used in many industries for purification or filtering. Scientists were surprised when they first realized that diatoms could still move with heavy shells through osmotic pressure and with a cytoplasmic foot extended from a slit on their frustules. The structures and properties of diatoms are reviewed and connections between microstructure and mechanics are drawn. In addition to mechanical properties, the glass-like shells of diatoms help turn light into energy in dim conditions due to the special shell structure. By consolidating available information about how the diatom works during their life cycle and the microstructure of their cell body then hypothesizing it from a materials science point of view, design principles for the development of advanced biomimetic materials with strong adsorption property, elasticity, and stiffness in addition to converging light can be formulated.
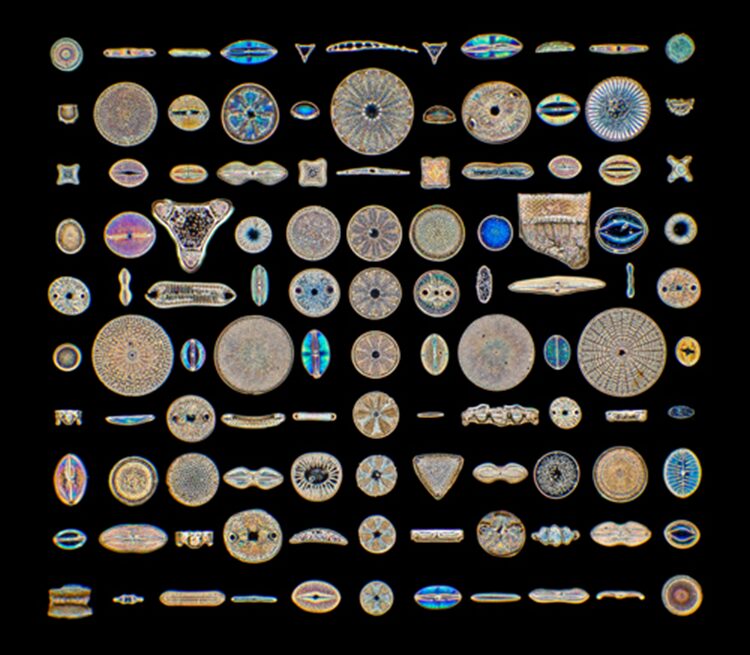
Figure 1: Taken from ‘Science and science’/Alamy Stock Photo 2021. The picture shows different diatoms in the world with different shapes but similar body components.
Introduction
The ancestor of the diatom has existed since the Stenian period. They had no function of photosynthesis at first and were huge, with many cilia allowing them to move. Some ancestors of modern diatoms conducted endosymbiosis of green algae and incorporated this system into their own. For example, the urea metabolism gene was gained to ensure the nitrogen-containing compounds that are not used to form urea are stored in the cell; the urea in the cell which increased the concentration of their cytoplasm could help them to control the osmotic pressure and buoyancy. Additionally, diatoms could elongate their cell bodies to increase buoyancy, so that the diatom could diffuse to larger spaces. This was the earliest mode of motion for diatoms. Furthermore, nitrogen monoxide as the complementary product of synthesizing urea could be used to attack pathogens (Benoiston et al., 2017). To scientists’ surprise, as time went by, the ancestor of the diatom gradually gave up the symbiosis with green algae but sometime later they began to engulf red alga and conduct endosymbiosis again.
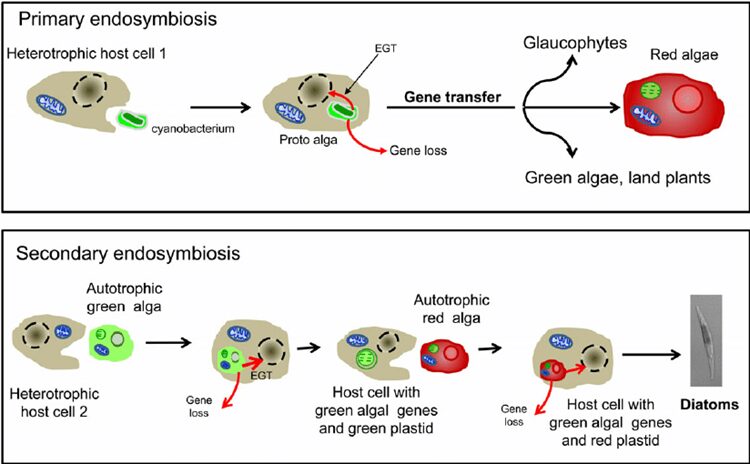
Figure 2: Schematic representation of the secondary endosymbiosis hypothesis of diatom evolution. (Moustafa et al.,2009). EGT, endosymbiotic gene transfer graph showing the progress of evolution in diatom and gene taken from green algae inserted in red algae.
This seems strange, why would nature put photosynthesis aside and then incorporate it again? They captured the gene of the green alga and inserted it into the cell body of the red alga which allowed them to proceed with the function of the green alga in the background of the red alga (Nisbet et al., 2004). Thanks to these features, the group of diatoms began to boom 300 million years ago. However, at that point when the diatom picked up photosynthesis again, it was the Permian, and even though the diatom had such capabilities, there were thousands of algae that survived the extreme environment. But it did not matter, the earth was struck by the explosion from Siberia, and the earth came to the Permian-Triassic extinction event. In an instant, the earth is almost back to before the Cambrian explosion, everything in the ocean is physically pulled back to the same starting line. There are still millions of unicellular creatures in the ocean, and the lawed (Chen et al., 2019). In addition, unicellular algae, which was at the bottom of the oceanic food chain, had as many enemies as the amount of sand in the Ganges River. In estimation, the plankton in the ocean was eaten up once in an average of 6-8 days. Moreover, there are billions of pathogens in the water that will attack the monocellular organisms. But then we thought, in a field like this, if the diatom improved a little in the ability to protect them from pathogens or microorganisms, multiplied by the enormous death rate, that would correspond to the survival of billions of offspring. The survival rate will cause a large difference for the plankton thanks to the fast reproductive rate (Unkown, 2011). To exist, the ancestor of diatom chose the most common way – making thicker and harder shells. But it is not a good method in the long run, because viruses and pathogens could also degrade the cell walls even though the shells were hard. Also, they can make the cell wall much thicker, but the energy cost is huge, so it is not worth it. To solve the problem, the ancestor of the diatom tried many paths and found a way to deal with it: the ‘diatoms’ chose to grow larger and larger, and gradually they evolved into a more complex multicellular creature. In the end, the branch of the diatom evolved into the largest Kelp— Phaeophycean. The kelp we eat in our common life is a kind of Phaeophycean despite the difference in appearance (Morrow & Algiers). Another branch of the ‘diatom’, which is more similar to the current diatom, chooses to form cell walls using silicon dioxide. To be more precise, they use hydrated silica, which is relatively hard to decompose (Hildebrand et al., 2018). Although this compound is commonly used by grass living in the sea to the grass living on the land, due to the insolubility of silicon dioxide in water, most organisms only compose a little for protection from predators. In comparison with them, the diatom uses a lot more silica, and they use complementary products like urea to increase the solubility of the silicon dioxide in the cell (Allen et al., 2011). As a result, the diatom forms the frustule made from silica. In addition, the diatom’s frustules with the microstructure including small hexagonal micropores which are arranged in a mesh structure on the frustule helps them to converge light so that it results in higher efficiency of photosynthesis especially for the diatom living under deep sea water (De Tommasi, 2016). The first diatom in the modern sense appeared in the Triassic period which is about 240 million years ago (Benoiston et al., 2017). In the following essay, we will delve into more details about the current diatom and the biomimetic material arising from them; we focus on the principle behind the microorganisms.
Mechanics of the frustule and diatom chains
Diatoms are characterized by their frustules, the rigid cell walls that make these organisms so identifiable, despite the incredible diversity in form and size between species of diatom. These frustules are composed nearly entirely of silica, with some naturally occurring impurities of hydrogen (H) and hydroxide (OH–). Their silica walls are hydrated and amorphous; they do not present any clear organization on the structural level, unlike crystals, and they are porous, as seen in Figure 3.
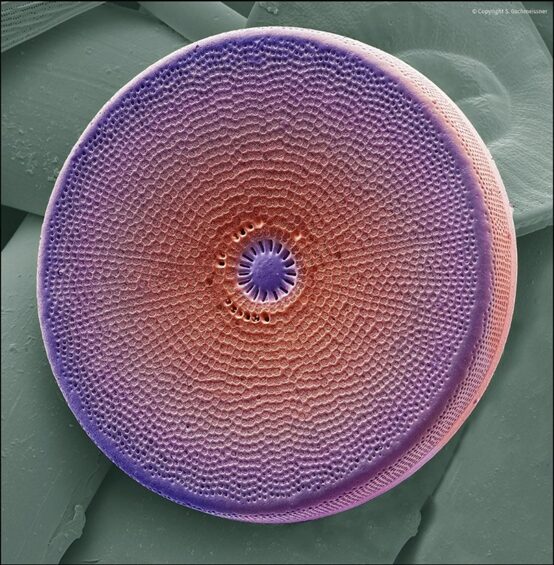
Figure 3: Electron micrograph of a diatom (Gschmeissner).
The frustules also contain organic elements, as found by Krammer et al., and are coated by a layer of polymers serving various purposes in the diatom’s interactions with the world around them, as well as other diatoms (De Tommasi et al., 2017). The shape of the frustule is commonly compared to that of a petri dish: it has two thecae, or valves, which are identical in shape but vary slightly in size, so that one may fit into the other. The smaller, or bottom hypotheca fits into the top or larger epitheca (Black and white “V” in Fig. 3) (De Tommasi et al., 2017). The valves are the main component of the frustule, and they exist primarily to protect the inner parts of the diatom and to allow for nutrient and gas exchange with the environment. The major role of the diatom frustule is to act as protection from predators (Hamm et al., 2003). Although diatoms are easily eaten and digested by many sizable predators, they have a very powerful protective barrier against predators on their scale. When reproducing asexually, even the slightest advantage in population size will ultimately create a very large disparity, and so this is an advantage that allows the diatoms to dominate the world of phytoplankton. Two of the main predators of diatoms and other phytoplankton are copepods and krill, seen in Figures 4 and 5 (Hamm et al., 2003).
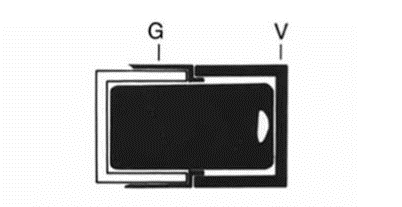
Figure 4: Schema of the diatom frustule (Gebushuber & Crawford 2006).
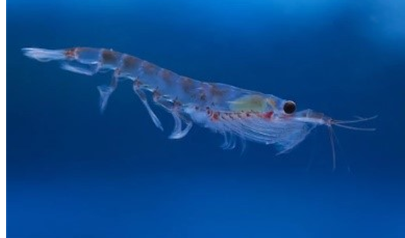
Figure 5: Krill (Source: Antarctic Krill).
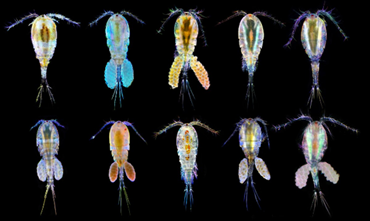
Figure 6: Copepods (Source: Copepods).
Both of these organisms have specialized cutting or crushing systems which are evidence of an evolutionary arms race between diatoms and their predators (Hamm et al., 2003). In loading tests done with glass needles, it was found that frustules of the genera F. kerguelensis required around 750 micro newtons to be broken, which would require their predators to have teeth or tools with a diameter of at least 50 microns, around twice the size of F. kerguelensis, at around 30 microns (Hamm et al., 2003)! This greatly reduces the number of predators that can prey on these diatoms, as they must be the largest of their species, while the smaller krill or copepods prey on other phytoplankton, further increasing diatom’s relative presence in their ecosystems. Interestingly, there is an inverse relationship between diatom size and stress resistance. The F. kerguelensis diatom, with a diameter of around 30 microns, has a stress limit of around 750 micronewtons, as stated before, while the genus C. granii, with a diameter of around 130 microns, only resisted stress up to 90 micronewtons before breaking (Hamm et al., 2003). Even in the case of C. granii, however, this is still a considerable amount of stress to resist considering the scale of the diatoms. This capacity for high-stress resistance is due to the design of the frustule, which allows it to distribute stress uniformly and over much of its surface. One of the most important features which contribute to this effect is the costae or ribs.
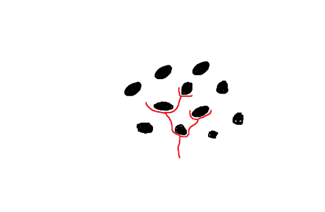
Figure 7: Path of a fracture (red) around the silicon beads (black).
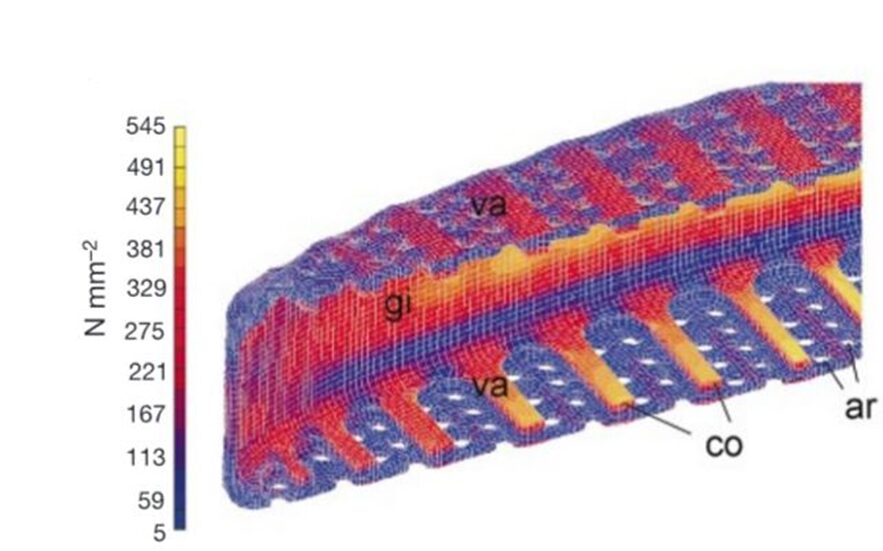
Figure 8: FEA of Frustule under stress (Hamm et al., 2003).
The costae, “co” in Figure 8, are parallel thick sections of the frustule, in between which are the porous parts of the valve. These porous sections are much weaker under stress, and so when a force is applied to the frustule, the stress is distributed as much as possible to the costume. As seen in Figure 8, this almost entirely removes the stress from the porous sections. If these ribs were not present, and the same amount of material was evenly distributed in the structure of the valve, the resistance to stress and deformation decreases by a huge 70%, and in some cases, even more (Hamm et al., 2003). Another remarkable feature of diatoms which allows them to resist such high amounts of stress stems from the fact that the silica composing the frustules is in the form of beads around 40 nanometers in diameter. When a fracture travels through the frustule, it does not pass through these beads and rather must pass around each one, as illustrated.
This massively increases the amount of force required to create a fracture in the structure (Hamm et al., 2003). Although these advantages create a structure that is highly resistant to mechanical pressures, other predators of the diatoms have developed systems that allow them to prey on and digest diatoms without the ability to fracture the frustule.
Girdles and reproduction
The valves of the frustule fit in place with the girdle bands (“G” in Fig. 4), whose purpose lies in the reproduction of the diatoms. Diatoms reproduce asexually, through mitosis. When they do so, the inner matter of the diatom, called the protoplasm, begins to expand, and the two valves begin to separate (Gebeshuber & Crawford, 2006). To avoid the protoplasm from being exposed to the environment, a second girdle band protrudes to fully contain and protect the protoplasm, as seen in Figure 9 below:
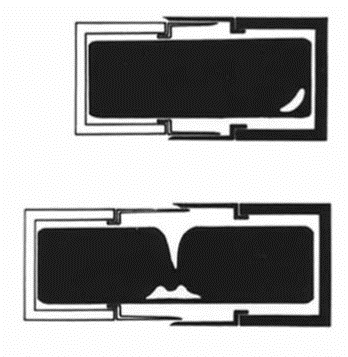
Figure 9: Schema of Girdle Bands During Diatom Reproduction.
The girdle bands are thus essential; their absence would doom the diatom’s protoplasm to “fall out” of their frustule or possibly be consumed by a predator. Finally, in the center of the parent cell, two new valves begin to form, one for each of the daughter cells. The organelle responsible for the formation of the new valves is the silica deposition vesicle, or SDV (Karp-Boss et al., 2014). The silica that composes the frustules is biomineralized, meaning that the diatom is entirely responsible for the production of its silica, and does not draw it in directly from its surroundings (Gebeshuber & Crawford, 2006). When the daughter cell approaches maturity, the membrane of the SDV, as it is a membranous organelle, and the membrane of the daughter cell fuse, the valves take their places, and the two new daughter cells are formed, as seen in Figure 10 (Karp-Boss et al., 2014). This model of valves and girdle bands is repeated in all species of diatoms, in many different forms.
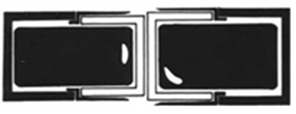
Figure 10: Result of Diatom Mitosis.
Elasticity of frustules
A natural question that arises from a physical point of view is whether the frustules that have just been formed are just as stiff, or rather elastic, depending on how one sees it, as the frustules mature. This happens to be fairly straightforward to compare since each daughter cell resulting from diatom mitosis has one mature and one young frustule. To quantify elasticity, Young’s modulus (E) is used, which is defined in the following way:
E=σ/ε=(F/A)/(dl/l)
Where 𝜎 is the tensile stress, the amount of force applied per unit area, and 𝜀 is the tensile strain, the change in length per unit length. A very high Young’s modulus is characteristic of a brittle material. This means that when very high stress is put on the material, it does not bend, but when the amount of stress applied reaches a certain threshold, it will break. A very low Young’s modulus is characteristic of so-called plastic materials. Highly plastic materials begin to bend when even a very small force is applied to them, but they do not have a threshold at which they will break, or that threshold is extremely high. Boss et al. tested the elasticity of newly formed frustules, mature frustules in divided cells, and undivided cells, and found that Young’s modulus of newly formed frustules was significantly lower than that of mature frustules of the same diatom and mature frustules in undivided cells. The Young’s moduli of newly formed frustules tended to be in the range of 0.25-1 MPa (1 MPa = 106 Pa, 1 Pa = 1 N/m2), while mature frustules in both divided and undivided cells tended to be in the range of 3.1-9.0 MPa (Karp-Boss et al., 2014). A difference of a factor around 9 is very important, and so a hypothesis is required to explain it. The current base of diatom knowledge indicates that no silica deposition occurs once the valves have left the SDVs, it is not thought that this disparity in elastic modulus is indicative of gradual silica deposition in the frustule as it matures. To explain this change in elasticity, Boss et al. propose the hypothesis that organic polymers are combined into the valve structure gradually as the diatom matures. They suggest the existence of regulatory genes controlling the synthesis of these polymers, such as polysaccharides and proteins, and that by varying the regulation of these genes, an organic matrix is constructed over time in the valve. This hypothesis is supported by the findings of Tesson and Hildebrand, who detected multiple distinct layers of polysaccharides in the frustule cell walls (Karp-Boss et al., 2014).
Diatom chain formation
One of the characteristics of diatom colonies is that they organize themselves into chains. The purposes of chain formation are not all known, but two of the most important are to prevent dispersion and to maintain the diatoms at the correct depth in the water. In more plentiful environments, diatom chains are longer, while in environments with limited nutrients, chains shorten, and their growth rate decreases (Young et al., 2012). A diatom chain is organized in such a way that the girdle bands are not impeded or mechanically fastened, allowing cell reproduction to occur unimpeded for the on average 30-50 cells composing the colony (Gebeshuber & Crawford, 2006). To hold together, chains may feature organic adhesive between valves, which forms a much more condensed chain, or mechanical interlocking features on each valve which allow them to form a connection with their neighbor. The mechanically fastened diatom chains are particularly impressive, and as this is a physics paper, we will keep our focus on these. We will study the examples of Syndectocystis and Ellerbeckia, pictured in Figures 9 and 10 respectively. Due to the nature of their environments, diatom chains must be able to withstand many different forces, including shear or torsion forces, as well as forces that would threaten to pull diatoms apart from their neighbours. A huge variety of solutions to this problem have been experimented with over the millions of years of evolution of diatoms. The first example is the genus Syndetocystis. Each valve in the chain has a stem that extrudes from it, towards its neighbour. At the end of the stem is a clasp with two sections, and when the stems meet, the clasps lock themselves around the stem of the other diatom, as seen in Figure 11.
There is an asymmetry present in the clasps which prevents them from rotating around each other, thus preventing any torsion from occurring, as well as not being able to be pulled apart due to the clasps interfering with each other (Young et al., 2012). Another example, found in general with radial symmetry, such as Ellerbeckia, is that of interlocking splines preventing rotational movement.
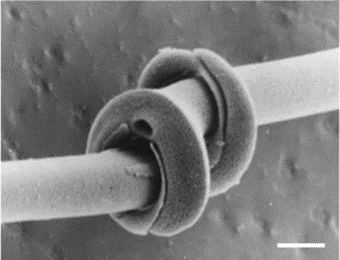
Figure 11: Syndectocystis clasps interlocked (Young et al., 2012).
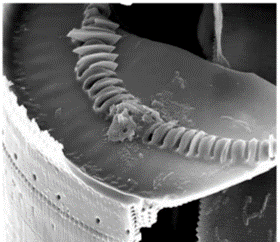
Figure 12: One end of an Ellerbeckia diatom, with protruding cavities to receive the splines of the neighbouring diatom (Young et al., 2012).
As seen in Figure 12, one valve presents openings for the extruded linking structure of the neighboring valve. Due to the formation of valves in the silicon deposition vesicle together, very high precision is attained with the interlocking of these structures (Young et al., 2012). In cases such as these, in which only the fossil is available, and the mechanism has no clear method of preventing neighbouring diatoms from being pulled apart, it is hypothesized that organic adhesive of some kind was used to reinforce the joint between the diatoms (Young et al., 2012). These are only a few examples of diatom designs, but tribology, the study of friction and surfaces in relative motion, can gain lots of insight and inspiration by further investigation of diatom chain formation.
The optics of Diatoms
Since Diatoms are photosynthetic cells, light plays an essential role in their survival. Without light, photosynthesis becomes impossible, and the diatom cell will die due to a lack of nutrients due to the light-dependent reactions in the chloroplasts. However, these cells often live in a deep underwater environment where light becomes scarce as its intensity decreases the deeper you go. This is important as light intensity is proportional to the metabolic rate of the algae and its biomass production (MacLeod & Barton, 1998). This presents an evolutionary challenge to diatoms as they must evolve a way to increase the amount of light (that is in the right range for photosynthesis) that arrives at the chloroplasts. However, they must also limit the amount of light that enters the cell since light radiation increases the concentration of oxygen radical (non-bonded oxygen species that is highly reactive and will try to react to stabilize itself) (Goessling et al., 2018). These reactive oxygen species cause a problem for the cell since they disrupt cell activities and interrupt various crucial integral biochemical reactions. This presents yet another evolutionary problem: how to increase the light intake without disrupting the cell’s functioning. Nature’s solution is a not-so-simple yet elegant solution: the frustules.
Diffraction in Diatoms
Diffraction phenomena are the basis for most of the other optical phenomena that will be discussed in this section. Diffraction is the bending of light around objects or an opening (“Diffraction,”). This was first explained by Christian Huygens in a principle that is now called the Huygens-Fresnel principle. Put simply, Huygens discovered that every point on the wavefront (the edge of the waves) can be considered a source of semi-circular waves and that the new wavefront at a certain distance in time is tangent to all the new secondary waves (“Diffraction,”). What happens around small openings is that the light will bend to the outside of the opening as can be seen in Figure 13.
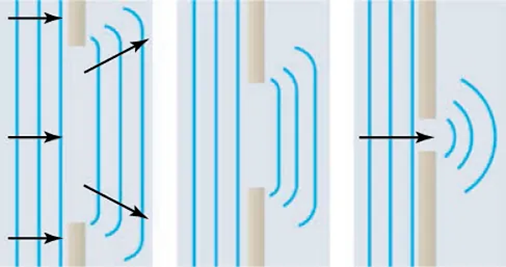
Figure 13: Diffraction around a small opening. Due to the Huygens principle, light will be observed at a dimmer intensity off the central axis of the opening (Paul Peter Urone, 2020).
Diffraction can be quantified and expressed using the following formula:
asinθ=mλ
Where a is the aperture of the diffracting slit, θ is the angle at which a diffraction maximum will be observed from the central axis, m is the order of the diffraction maximum and λ is the wavelength of the diffracted light. This has 2 main implications. First, the diffraction pattern will vary depending on the wavelength of the incoming light and the width of the aperture can be measured using the diffraction pattern.
Diatoms utilize this phenomenon with the many pores that they exhibit on the valves of their frustule (Figure 14). These pores have a very small aperture that varies around 1.4 micrometres (De Tommasi, 2016). The light that enters one of the many pores diffracts as was observed by research conducted at Cambridge University (Noyes et al., 2008). They observed that diatoms diffract light very strongly in air as well as in water. Using measurements of the angular separation of the diffraction pattern, they were able to confirm the size of the pores and the distance between them (due to interference effects that will be explained later).
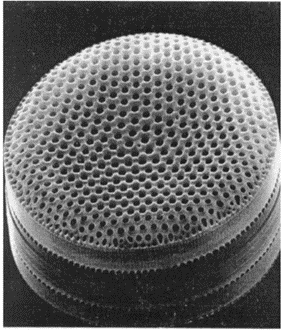
Figure 14: Pores on the frustule of a C. Walesii diatom. The picture was taken with an electron microscope (Longuet-Higgins, 2001).
Another similar optical phenomenon to diffraction that occurs in diatoms is interference. Interference occurs when two separate sources of light occupy the same space. Since diatoms have many pores that diffract, they will also have plenty of interference in the cytoplasm of the cell. Waves are mathematically described as trigonometric functions (sine or cosine) and when they occupy the same space, they follow the superposition principle. The superposition principle simply states that when two waves are in the same space, they do not interact with each other, they simply pass through one another. However, in the space where they do overlap the resulting wave is simply the sum of the amplitude of both waves (Raymond, 2016). This means that two diffracted waves can completely cancel each other out or have very low (or high) amplitude over a certain distance.
Research by Dr. De Tomassi at the Institute for Microelectronics and Microsystems in Naples shows that diatoms are cleverly able to manipulate this phenomenon to their advantage. The diffraction across the many pores of the valve structure causes hotspots where light reaches high intensities due to interference between the waves that are diffracted in different pores (De Tommasi, 2016). Research was also able to show that the location of the hotspots was directly correlated with the wavelength of the diffracted light. Longer wavelengths of light have hotspots that are close to the rim of the diatom whereas shorter wavelengths may reflect further away from the shell or even as far outside the cell of the diatom completely. This provides a mechanism for diatoms to in a way block off the nefarious ultra-violet radiation and safeguard its biochemical equilibrium from reactive oxygen species (De Tommasi, 2016).
Photonic crystal properties of diatoms
A photonic crystal is a material with a crystal structure (meaning that they have a regular repeating lattice), which interacts with light in a different way than regular crystals do. The repeating microscopic pattern in a photonic crystal is a regular structure made from either dielectric material or metallic material (Gangwar et al., 2023). A dielectric material is a material that is an electrical insulator (that does not allow current to pass through), but that can be polarized to induce an electric field inside the material that will oppose the external electric field (Poplavko, 2019). A common dielectric material that is used for many applications is silicon dioxide (silica) which is the same material that the frustule of diatoms is composed of. As can be seen in Figure 14, the surface of the frustule has a semi-repeating pattern (it is an imperfect honeycomb pattern, not all pores are of the perfect aperture and distance apart from the others (Goessling et al., 2018)) of pores that are made of a dielectric material. The surface of the diatom is therefore a two-dimensional photonic crystal, (Fuhrmann et al., 2004) and this modifies the way light interacts with the diatom cells. These are the electrical properties of a photonic crystal. The photonic crystal also possesses optical properties which refers to the refractive index of the dielectric material. The repeating pattern of dielectric material will also be a repeating pattern of a high refractive index material (it will be 1.43 in the diatom case, as measured with the index-matching liquids method (Fuhrmann et al., 2004)).
The major optical property of a photonic crystal is its photonic band gap. The photonic band gap refers to a range of electromagnetic wave frequencies that cannot propagate in the crystal structure will be completely reflected off the surface and will not be transmitted through the material. While experimental measurements of the photonic bandgap are scarce for now, theoretical using the size, shape, positioning, and patterning of the pores have been able to approximate the photonic bandgap and help investigate their optical properties. The results were that the photonic bandgap highly depends on the periodicity of the lattice, the diameter of the pore, and the depth of the pore (Mishler, 2015). The photonic bandgap for a periodicity of 1840 nm the bandgap is centred around 4319.6 nm which corresponds to the microwave range of electromagnetic radiation.
Waveguide properties of diatoms
Waveguide refers to physical objects that can confine a wave in three dimensional and limit the propagation of their energy. The waves are therefore “guided” in a certain direction (Pfeiffer, 1963). In the case of electromagnetic, the waveguide will limit the propagation of the electric and magnetic fields not only in space but also in orientation. The orientation of electric and magnetic fields that are allowed to propagate inside the waveguide are referred to as a mode of the waveguide (more precisely the modes are the solutions of the set of equations governing the propagation of waves inside the waveguide). A simple example of a waveguide with modes is the formation of standing waves on a string.
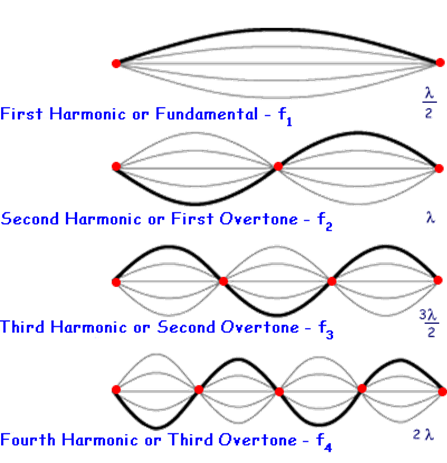
Figure 15: Harmonics of a standing wave system. This is a simple example of a waveguide system with modes (Unkown, 2023).
In the example of the standing waves, the waveguide is the nodes at both ends of the string (think of both ends of a guitar as an example) and the modes are the allowed wavelengths of the standing waves. What can be noticed from this example is that at low frequencies (below the ones for the fundamental, also recall that standing waves are formed by the superposition of a wave and its reflection), there will be no standing wave and thus it will not be propagated through the system. This is also the case for electromagnetic waveguides. Photonic crystals are an example of a waveguide and are also where diatoms get their waveguide properties.
The frustules of the diatom have been observed to exhibit waveguide properties in many different ways. First, it has been observed that due to the hexagonal pore structure of the frustules, diatoms can essentially couple (transferring light from one waveguide to another) light directly to the chloroplasts for an effective light collection in photosynthesis (Fuhrmann et al., 2004). Theoretical models have predicted that the waveguiding properties of the diatom shell are highly wavelength-dependent and only occur at very small and specific wavelength bands (Noyes et al., 2008). This range has been determined to be in the near-infrared range. This result has also been observed recently experimentally in research conducted at the University of Copenhagen (Goessling et al., 2018). Other research has observed butt-coupling properties of diatoms. In the N. filiformis case, the diatom has an elongated cell structure. It has waveguided properties that allow for light propagation along the length of its structure, but it has also been observed that it can couple light from its end into another optical waveguide (possibly another diatom). While this has not been confirmed experimentally, being able to share light with members of the same species is an important evolutionary advantage and might have led to the development of waveguiding along the length of the cell (D’Mello et al., 2022).
Focusing properties of diatoms
Lenses are commonly used to focus light rays onto a particular point or area such as in glasses. They operate on the refraction principle. As light changes mediums between air and glass (common material in lenses), its speed will decrease due to the differing properties of the medium. Light will therefore want to optimize the time it takes to travel between 2 points rather than the shortest distance following Fermat’s principle (Adam & Urbach, 2021). This will cause light to change its direction of travel as it goes through the lens as described by Snell’s Law (Figure 16).
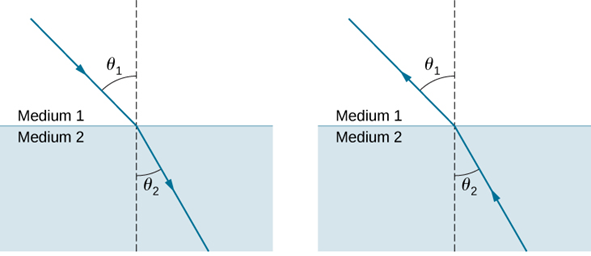
Figure 16: Refraction of light as it changes medium. The relationship between the 2 angles is described in Snell’s Law: n1sin(Θ1) = n2sin(Θ2) where n is the refractive index of the medium (Refraction, 2016).
Diatoms have been observed to exhibit lens-like properties. Unlike traditional lenses, however, diatoms do not focus light using refraction. They use diffraction instead. In a very interesting study conducted by Dr. De Stefano, they analyzed the lens-like properties of the C. Walesii diatom. What they discovered is that even though the diatom has no lens, it was still able to focus a laser beam of 100-110 micrometres down to around 8.1 micrometres (Stefano et al., 2007). The diatom essentially acted as a microlens. The way the diatom accomplishes this is by utilizing once again the pores on their valve. The light that goes through the pores is diffracted and only interacts constructively at certain spatial positions (Stefano et al., 2007). This was confirmed by measuring the focal length of the system at different wavelengths of light as if the focusing effect was due to interference, the focal will be inversely proportional to the wavelength of the light being focused. This relationship has been plotted and confirmed by Dr. De Stefano’s team as can be seen in Figure 17 (Stefano et al., 2007). So, the consequence is the observed lens focusing effect without ever having a lens. It is an example of structural optics.
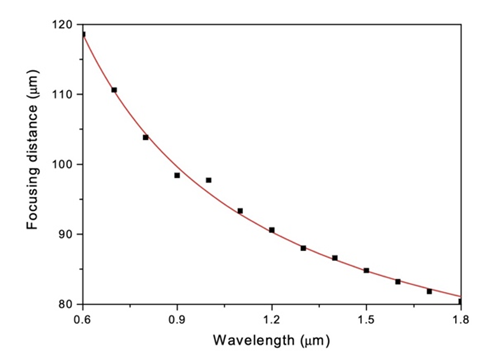
Figure 17: Relationship between focusing distance and wavelength in the C. Walesii diatom. In red are the expected (calculated) values and the black points are the experimental values (Stefano et al., 2007).
Passive transport
The absence of flagella or cilia makes diatoms unable to move substantial distances in water (Sabuncu et al., 2021). To have continual access to nutrients and sunlight, diatoms mainly rely on the turbulence of a body of water. It keeps diatoms near the surface of the water, maintains a nutrient-rich environment, and distorts the local chemical gradient around the cell wall to allow more nutrient absorption (Amato et al., 2017). While turbulence is an important factor in diatom survival, they have their hidden mechanisms of transportation.
Buoyancy control
Some species of diatoms can travel within a water column by controlling and changing their buoyancy in response to different factors (Arrieta et al., 2020; Gross et al., 1948). Diatoms reside in the euphotic zone, close to the surface of the water (Gross et al., 1948). A healthy vegetative cell has a specific gravity equal to sea water allowing it to stay near the surface, which is due to the cell sap having a lower specific gravity than water which counterbalances the heavier components of a diatom. While diatom cell sap is most likely isotonic with seawater, the lower specific gravity of cell sap correlates with its lower concentrations of divalent ions.
In the case of colony overcrowding, high temperatures (>20°C), or colony contamination, diatom cells can adjust their buoyancy to increase their sinking rate, which is primarily done by expelling the cell sap as the cell undergoes plasmolysis (Gross et al., 1948). Vegetative cells will retract their plasma membrane from the siliceous cell wall and the protoplast will condense into a compact cell known as a resting spore which can be seen in Figure 18, suited to withstand adverse environmental conditions. This higher-density cell will sink rapidly and can be found in lower depths. When conditions improve, the resting spore can expand its protoplast until it fills out the cell wall, re-establishing turgidity, and in the case that the cell wall was removed or destroyed, the resting spore can expand into an auxospore which creates a new cell wall.
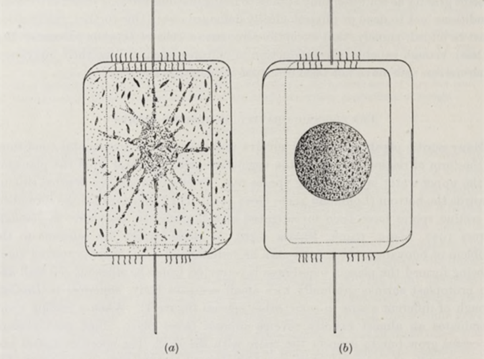
Figure 18: Diagram of Ditylum Brightwelli. (a) vegetative cell; (b) resting spore (Gross et al., 1948).
Another trigger of buoyancy regulation is the mechanical stress of turbulence (Amato et al., 2017; Gross et al., 1948). While diatoms were initially thought to be completely passive regarding the turbulent waters that transport them, they have been shown to change their gene expression in response to turbulence (Amato et al., 2017). Recent experiments show that they regulate metabolic pathways in turbulent currents. In the presence of turbulence, fatty acid biosynthesis as well as other metabolic pathways were heavily upregulated, lowering the cell density and keeping the diatom near the surface (Amato et al., 2017; Gross et al., 1948). This is still not fully understood as turbulence should not be a stressor for diatoms, but it gives another insight into why diatoms thrive in turbulent environments rather than still water (Amato et al., 2017).
Diatom Gliding
Diatoms possess seemingly no locomotory organelles, yet somehow, they can move small distances across a solid substrate (Sabuncu et al., 2021). Some species of diatom have been observed to be able to glide on a substrate, which is achieved using one of the diatom’s key features, the raphe (Edgar & Pickett-Heaps, 1983; Gebeshuber et al., 2021; Ruck & Theriot, 2011). The raphe is a slit through the siliceous cell wall of a diatom running from the top of the diatom to the bottom, separated by a central nodule (Edgar & Pickett-Heaps, 1983). At the end of each raphe, there is a small circular opening (Edgar & Pickett-Heaps, 1983; Ruck & Theriot, 2011). The diatom can only move when the raphe is adjacent to the substrate, and the raphe secretes a glue-like substance called mucilage that the diatom moves against (Edgar & Pickett-Heaps, 1983; Gebeshuber et al., 2021; Sabuncu et al., 2021). Each raphe of a diatom functions autonomously from the other (Hopkins & Drum, 1966).
The motion of diatoms along a substrate has neither been observed to be uniform nor unidirectional, meaning the diatom will accelerate quickly and then stop in a jerky motion (Sabuncu et al., 2021). While it is not fully understood how diatoms move along a substrate, it is generally accepted that mucilaginous polysaccharide fibrils and actin microfilaments are at play (Edgar & Pickett-Heaps, 1983; Gebeshuber et al., 2021). One proposed theory is that vesicles transport mucilaginous polysaccharide fibrils which are discharged into the raphe (Edgar & Pickett-Heaps, 1983). Once in the raphe, they hydrate and swell, and then the distal end of the fibril projects through the raphe to bind to a substrate. On the proximal end, actin microfilaments bind to the mucilaginous fibrils and can displace them along the hydrophobic lipid-coated raphe until the fibril reaches the other end of the slit, detaches, and quickly dissolves from the substrate which is seen illustrated in figure 19.
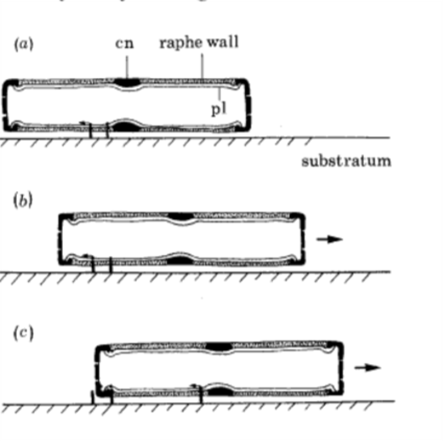
Figure 19: The proposed movement of the diatom Navicula Cuspidata. (a) mucilaginous polysaccharide fibrils are secreted near the central nodule and distally bind to the substrate while the proximal end binds to actin microfilaments within the cell. (b) The actin microfilaments move the mucilage distally to the left relative to the cell, which pushes the cell toward the right. (c) Mucilage fibrils are removed from the raphe at the apical ending, while new fibrils are secreted near the central nodule. The mucilage trail rapidly dissolves in the surrounding medium. (Edgar & Pickett-Heaps, 1983).
There are a few proposed reasons why motile diatoms move along substrates. Depending on the species, some motile diatoms show phototaxis, either moving towards or away from sources of light, which could be done to optimize light conditions (Gebeshuber et al., 2021). Similarly, some species show chemotaxis, moving towards chemical conditions that are well suited for them. Another possible benefit of locomotion concerns diatoms that inhabit sediment which may need to move upwards to remain at the surface of the sediment. Most importantly, the ability to move around can greatly aid diatoms in contacting mates for sexual reproduction.
Colony formation and movement
Bacillaria Paxillifer is a species of diatom that is both motile and colonial, meaning that multiple cells associate in a chain and the colony moves rhythmically (Gebeshuber et al., 2021; Kapinga & Gordon, 1992). By initial observations, it was hypothesized that the uneven protrusions on the side of the raphe fissures that are unique to this species allow them to connect in the chain formation by “hooking” to each other (Kapinga & Gordon, 1992). Further observations that showcase Bacillaira Paxillifer’s ability to bend while maintaining cell-to-cell attachment disprove this theory, although it is still possible that protrusions play a role in cell-to-cell attachment. Due to the elasticity of the observed attachment, mucilage is a contender for maintaining the valve-to-valve connection between adjacent cells. While mucilage is elastic enough to allow for the observed range of bending from a diatom chain, it dissolves too quickly in the aquatic environment to be a long-lasting bond. Hypothetically, if the uneven silica protrusions could fit together to form a tube around the mucilage, it could protect the fibrils from being dissolved into the surrounding medium.
When Bacillaria Paxillifer travel, they move together as a colony (Gebeshuber et al., 2021; Kapinga & Gordon, 1992). While the linkage does allow cells to shift forward concerning other cells, there is always at least 15% of cell body overlap with an adjacent cell (Kapinga & Gordon, 1992). By further analyzing mucilage fibrils, it can be determined whether they have anomalous viscosity (viscosity that is extremely high at low shear rates and low at high sheer rates), which could explain how the bonds between cells are strong enough to keep them together while non-motile, and weak enough to allow the cells to extend when they generate sufficient force. To move as a colony, diatoms in the chain must glide nearly simultaneously. Furthermore, when a colony experiences environmental stressors such as a large change in light exposure, a large change in temperature, or vigorous shaking, each cell will nearly perfectly align and become non-motile. Although many details of intercellular communication are unknown since there is no cell-to-cell contact with adjacent plasmalemmas, cells likely need to communicate through the mucilaginous polysaccharide fibrils and actin microfilaments to synchronize movement.
Conclusions
Overall, the diatom is an ancient creature with many incredible physical mechanisms including controlling self-buoyancy by expelling the cell sap as the cell undergoes plasmolysis. the frustule of the diatom has many functions like protection, mechanical pressure tolerance, light focusing abilities, photonic crystal properties, photonic band gap, and waveguiding which can confine a wave in three dimensional and limit the propagation of their energy in addition to wave shielding. In the different sections outlined above, the researchers have explored the structures and related functions in addition to how they harvest light using the nano-scale lattice structure. The diatom needs light, but it also needs to be protected from harmful frequencies of light, so the diatom frustules and valves present the most impressive resemblance with artificial photonic crystals and exhibit a photonic bandgap. They converge light in hot spots where photosynthesis is conducted while filtering and reflecting harmful UV light away from the host at the same time. Diatoms are also sometimes trapped in dim space or must live in dark environments for extended periods. As a result, the frustule of diatom develops waveguiding properties. Thanks to the hexagonal pore structure of their frustules, diatoms can couple light directly to their chloroplasts which allows them to conduct photosynthesis effectively when the surrounding light is scarce. Diatoms also need to float and sink depending on the circumstances, in general they have gravity equal to seawater allowing them to stay near the surface, to float they lower the concentrations of divalent ions in their cell sap and increase the concentrations for sinking. In addition, diatoms have slits on their frustules so that they could move by extending cytoplasm out of shells which also allows them to stick to the rocks for them to stay at fixed regions if there are enough nutrients. The silica shell of the diatom protects them from predators but also makes their reproduction difficult. To solve this problem, the valves of the frustule fit in place with the girdle bands so that they could conduct mitosis without protoplasm being exposed to the outside environment. To sum up, the frustule of diatom possesses many physical properties such as optics, stress, and osmotic pressure (resulting in buoyancy) and structural features such as pores and a raphe for gliding. The diatom can move with such a heavy shell relative to themselves. Nature has found an elegant solution to many of the diatom’s problems using one simple highly optimized structure: the frustule.
References
Adam, S. K. A. J. L., & Paul Urbach, H. (2021, March 13) Principle of Fermat. LibreTexts Physics. https://phys.libretexts.org/@go/page/57071
Allen, A. E., Dupont, C. L., Oborník, M., Horák, A., Nunes-Nesi, A., McCrow, J. P., Zheng, H., Johnson, D. A., Hu, H., Fernie, A. R., & Bowler, C. (2011). Evolution and metabolic significance of the urea cycle in photosynthetic diatoms. Nature, 473(7346), 203-207. https://doi.org/10.1038/nature10074
Amato, A., Dell’Aquila, G., Musacchia, F., Annunziata, R., Ugarte, A., Maillet, N., Carbone, A., Ribera d’Alcalà, M., Sanges, R., Iudicone, D., & Ferrante, M. I. (2017). Marine diatoms change their gene expression profile when exposed to microscale turbulence under nutrient replete conditions. Scientific Reports, 7(1), 3826. https://doi.org/10.1038/s41598-017-03741-6
Antarctic Krill. (2023, November 8). Australian Antarctic Program. Retrieved October 1, 2023 from https://www.antarctica.gov.au/about-antarctica/animals/krill/
Arrieta, J., Jeanneret, R., Roig, P., & Tuval, I. (2020). On the fate of sinking diatoms: the transport of active buoyancy-regulating cells in the ocean. Philosophical Transactions of the Royal Society A: Mathematical, Physical and Engineering Sciences, 378(2179), 20190529. https://doi.org/doi:10.1098/rsta.2019.0529
Benoiston, A.-S., Ibarbalz, F. M., Bittner, L., Guidi, L., Jahn, O., Dutkiewicz, S., & Bowler, C. (2017). The evolution of diatoms and their biogeochemical functions. Philosophical Transactions of the Royal Society B: Biological Sciences, 372(1728), 20160397. https://doi.org/doi:10.1098/rstb.2016.0397
Copepod. (2023, September 21) In Wikipedia. https://en.wikipedia.org/wiki/Copepod
Chen, Y., Richoz, S., Krystyn, L., & Zhang, Z. (2019). Quantitative stratigraphic correlation of Tethyan conodonts across the Smithian-Spathian (Early Triassic) extinction event. Earth-Science Reviews, 195, 37-51. https://doi.org/https://doi.org/10.1016/j.earscirev.2019.03.004
D’Mello, Y., Bernal, S., Petrescu, D., Skoric, J., Andrews, M., & Plant, D. V. (2022). Solar energy harvesting mechanisms of the frustules of Nitzschia filiformis diatoms. Optical Materials Express, 12(12), 4665-4681. https://doi.org/10.1364/OME.473109
De Tommasi, E., Gielis, J., & Rogato, A. (2017). Diatom Frustule Morphogenesis and Function: a Multidisciplinary Survey. Marine Genomics, 35, 1-18. https://doi.org/10.1016/j.margen.2017.07.001
De Tommasi, E. (2016). Light Manipulation by Single Cells: The Case of Diatoms. Journal of Spectroscopy, 2016, 2490128. https://doi.org/10.1155/2016/2490128
Diffraction. https://phys.libretexts.org/@go/page/16195
Edgar, L. A., & Pickett-Heaps, J. D. (1983). The Mechanism of Diatom Locomotion. I. An Ultrastructural Study of the Motility Apparatus. Proceedings of the Royal Society of London. Series B, Biological Sciences, 218(1212), 331-343. http://www.jstor.org/stable/35706
Fuhrmann, T., Landwehr, S., El Rharbi-Kucki, M., & Sumper, M. (2004). Diatoms as living photonic crystals. Applied Physics B, 78(3), 257-260. https://doi.org/10.1007/s00340-004-1419-4
Gangwar, R. K., Pathak, A. K., Qin, J., & Wang, X. (2023). 10 – Physics of photonic crystals and applications. In S. K. Sharma, C. Jacinto da Silva, D. J. Garcia, & N. Shrivastava (Eds.), Modern Luminescence from Fundamental Concepts to Materials and Applications (pp. 313-327). Woodhead Publishing. https://doi.org/https://doi.org/10.1016/B978-0-323-89954-3.00011-9
Gebeshuber, I. C., Zischka, F., Kratochvil, H., Noll, A., Gordon, R., & Harbich, T. (2021). Diatom Triboacoustics. In Diatom Gliding Motility (pp. 249-282). https://doi.org/https://doi.org/10.1002/9781119526483.ch11
Gebeshuber, I. C., & Crawford, R. M. (2006). Micromechanics in biogenic hydrated silica: Hinges and interlocking devices in diatoms. Proceedings of the Institution of Mechanical Engineers, Part J: Journal of Engineering Tribology, 220(8), 787-796. https://doi.org/10.1243/13506501jet163
Goessling, J. W., Su, Y., Cartaxana, P., Maibohm, C., Rickelt, L. F., Trampe, E. C. L., Walby, S. L., Wangpraseurt, D., Wu, X., Ellegaard, M., & Kühl, M. (2018). Structure-based optics of centric diatom frustules: modulation of the in vivo light field for efficient diatom photosynthesis. New Phytologist, 219(1), 122-134. https://doi.org/https://doi.org/10.1111/nph.15149
Gross, F., Zeuthen, E., & Yonge, M. (1948). The buoyancy of plankton diatoms: a problem of cell physiology. Proceedings of the Royal Society of London. Series B – Biological Sciences, 135(880), 382-389. https://doi.org/doi:10.1098/rspb.1948.0017
Hamm, C. E., Merkel, R., Springer, O., Jurkojc, P., Maier, C., Prechtel, K., & Smetacek, V. (2003). Architecture and material properties of diatom shells provide effective mechanical protection. Nature, 421(6925), 841-843. https://doi.org/10.1038/nature01416
Hildebrand, M., Lerch, S. J. L., & Shrestha, R. P. (2018). Understanding Diatom Cell Wall Silicification—Moving Forward [Review]. Frontiers in Marine Science, 5. https://doi.org/10.3389/fmars.2018.00125
Hopkins, J. T., & Drum, R. W. (1966). Diatom motility: An explanation and a problem. British Phycological Bulletin, 3(1), 63-67. https://doi.org/10.1080/00071616600650081
Kapinga, M. R. M., & Gordon, R. (1992). CELL ATTACHMENT IN THE MOTILE COLONIAL DIATOM BACILLARIA PAXILLIFER. Diatom Research, 7(2), 215-220. https://doi.org/10.1080/0269249X.1992.9705214
Karp-Boss, L., Gueta, R., & Rousso, I. (2014). Judging Diatoms by Their Cover: Variability in Local Elasticity of Lithodesmium undulatum Undergoing Cell Division. PloS one, 9(10), e109089. https://doi.org/10.1371/journal.pone.0109089
Longuet-Higgins, M. S. (2001). Geometrical Constraints on the Development of a Diatom. Journal of Theoretical Biology, 210(1), 101-105. https://doi.org/https://doi.org/10.1006/jtbi.2001.2301
MacLeod, N. A., & Barton, D. R. (1998). Effects of light intensity, water velocity, and species composition on carbon and nitrogen stable isotope ratios in periphyton. Canadian Journal of Fisheries and Aquatic Sciences, 55(8), 1919-1925. https://doi.org/10.1139/f98-075
Mishler, J. H. (2015). Modeling Diatom Frustules as Photonic Crystals [Honors Thesis, University of Arkansas]. http://www.jbherzog.com/pubs/MishlerThesis.pdf
Morrow, M. H. M., & Algiers, K. Brown Algae and Diatoms. https://bio.libretexts.org/@go/page/31928
Nisbet, R. E. R., Kilian, O., & McFadden, G. I. (2004). Diatom Genomics: Genetic Acquisitions and Mergers. Current biology, 14(24), R1048-R1050. https://doi.org/10.1016/j.cub.2004.11.043
Noyes, J., Sumper, M., & Vukusic, P. (2008). Light manipulation in a marine diatom. Journal of Materials Research, 23(12), 3229-3235. https://doi.org/10.1557/JMR.2008.0381
Paul Peter Urone, R. H. Physics. (2020, March 26) Understanding Diffraction and Interference. OpenStax. https://openstax.org/books/physics/pages/17-1-understanding-diffraction-and-interference
Pfeiffer, M. A. G. a. R. (1963). Feynman’s Tips On Physics -24 Waveguides. Retrieved October 1, 2023 from https://www.feynmanlectures.caltech.edu/II_24.html
Poplavko, Y. M. (2019). Chapter 7 – Dielectrics. In Y. M. Poplavko (Ed.), Electronic Materials (pp. 287-408). Elsevier. https://doi.org/https://doi.org/10.1016/B978-0-12-815780-0.00007-4
Refraction. (2016). https://phys.libretexts.org/@go/page/4487
Ruck, E. C., & Theriot, E. C. (2011). Origin and Evolution of the Canal Raphe System in Diatoms. Protist, 162(5), 723-737. https://doi.org/https://doi.org/10.1016/j.protis.2011.02.003
Sabuncu, A. C., Gordon, R., Richer, E., Manoylov, K. M., & Beskok, A. (2021). The Kinematics of Explosively Jerky Diatom Motility: A Natural Example of Active Nanofluidics. In Diatom Gliding Motility (pp. 33-63). https://doi.org/https://doi.org/10.1002/9781119526483.ch2
Stefano, L. D., Rea, I., Rendina, I., Stefano, M. D., & Moretti, L. (2007). Lensless light focusing with the centric marine diatom Coscinodiscus walesii. Optics Express, 15(26), 18082-18088. https://doi.org/10.1364/OE.15.018082
Raymond, D. J. (2016, May 17).Superposition Principle. https://phys.libretexts.org/@go/page/32921
Unknown. (2011). Microbiology by numbers. Nature Reviews Microbiology, 9(9), 628-628. https://doi.org/10.1038/nrmicro2644 Young, A. M., Karp-Boss, L., Jumars, P. A., & Landis, E. N. (2012). Quantifying diatom aspirations: Mechanical properties of chain-forming species. Limnology and Oceanography, 57(6), 1789-1801. https://doi.org/10.4319/lo.2012.57.6.1789