Abstract
Evolution and the adaptation of different species to different ecological niches has resulted in a vast array of differences between the sensory systems of those animals, with some animals gaining new adaptive sensory capabilities and others losing the ones they no longer need for survival. This paper will discuss four examples of animals that, through evolution, have undergone a mutation to one of their sensory systems or gained a new sensory ability altogether, which promotes the survival of their species. First, the paper will explore how vertebrates perceive taste and how hummingbirds have undergone a mutation in their taste perception system, which has allowed them to respond to sweet tastes, even though most other birds can only respond to savoury tastes. Then the essay explains the function and structure of pit organs and their importance to snakes that use heat vision to search for and identify their prey. After that, the paper discusses how whip spiders have evolved to form a pair of antenniform legs composed of numerous mechano- and chemosensory sensilla that allow the nocturnal species to navigate the world around them. Finally, the paper will investigate the canines’ unique and powerful olfactory system that allows for one of the strongest and most sensitive noses.
Introduction
Animals can perceive and respond to their surroundings using the common five senses: vision, olfaction, taste, hearing, and tactile perception, as well as other sensory capabilities. Since different animal species face unique environmental pressures, caused by the ecological niches in which they live, their sensory systems and capabilities have evolved and adapted to best suit their living conditions and promote their survival by aiding hunting, finding food, and other necessary actions. This paper will focus on a few such cases of animals who have undergone evolutionary changes to one of their sensory systems to better suit their needs. Examples of these evolved senses include taste perception in hummingbirds, heat vision in snakes, antenniform legs in whip spiders, and hypersensitive olfaction in canines.
Taste Perception in Hummingbirds
The sense of taste is a chemosensory system with the purpose of evaluating all foods and drinks that enter an animal’s body. Taste stimuli are generally categorized into five groups: sweet, bitter, sour, salty, and savoury (or umami) tastes (Yarmolinsky et al., 2009). For animals, their sense of taste acts as a sensor to detect essential nutrients and as an early-warning system for potentially poisonous foods. For example, sweet and umami tastes are primarily considered ‘good’ tastes because they are associated with the consumption of nutrients like carbohydrates and proteins. Meanwhile, sour and bitter tastes are ‘bad’ because they alert the organism of potential toxins or harmful substances, categorized by low pH. Thus, each species’ capacity for taste perception has uniquely evolved to not only fit their preferences but also to promote their survival (Yarmolinsky et al., 2009).
Chemistry of Taste Perception
Taste perception is mediated by taste receptor cells (TRCs) that are grouped into taste buds, distributed across the epithelial tissue of the tongue and palate. When the TRCs are activated by tastants, which are the stimulating chemicals in food, they transmit information via sensory afferent fibres to the gustatory cortex in the brain (Ahmad & Dalziel, 2020). These taste receptor cells are able to sense different tastes because they express G protein-coupled receptors (GPCRs) on their surface.
GCPRs are signalling proteins responsible for mediating a variety of cellular responses (Basith et al., 2018). Class-C GPCRs are called metabotropic glutamate receptors (mGluRs) and modulate synaptic transmission and neuronal excitability throughout the central nervous system (Basith et al., 2018). In vertebrates, tastes are sensed by a family of class-C GPCRs called T1Rs (Rowland et al., 2015). The structure of a GPCR consists of seven transmembrane (7TM) alpha-helices connected by three intracellular and three extracellular loops, along with an extracellular N-terminus and an intracellular C-terminus. For class-C GPCRs, there are unique ligand-binding pockets in the extracellular domain, which contain a venus flytrap (VFT) domain, named for the way in which it switches between an open and closed conformation to bind agonists (Figure 1) (Antinucci & Risso, 2017). Since these receptors must accommodate a broad range of tastants, like carbohydrates, proteins, and lipids, T1R receptor complexes contain many active sites on their surfaces that participate in ligand binding (Yarmolinsky et al., 2009).
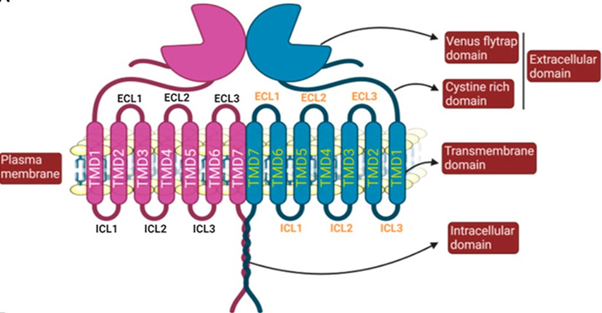
T1R Taste Receptors
The T1R family consists of three proteins: T1R1, T1R2, and T1R3, encoded by the genes Tas1r1, Tas1r2, and Tas1r3, respectively. The heterodimer formed by T1R1 and T1R3 mediates savoury, or ‘umami’ tastes by binding with L-amino acids. In contrast, the heterodimer formed by T1R2-T1R3 is responsible for mediating sweet tastes by binding with carbohydrates and sweeteners (Figure 1) (Treesukosol et al., 2011). Different animal species have, through evolution, lost one of these receptors, often because their diet did not require it. For example, the diets of different bird species vary widely, from fruits and nectars to seeds, insects and other animals. However, when a study was conducted, looking at the genome sequences of 10 birds with different diets, researchers found that the genes that encode for T1R1 and T1R3 were always present, but they failed to detect the T1R2-encoding gene in any of the species (Baldwin et al., 2015). This result would suggest that all observed bird species could only have functioning T1R1-T1R3 receptors and would therefore have diets consisting mainly of protein-rich foods. Closely related non-avian reptiles, such as the Chinese alligator, were found to have retained this gene, suggesting that the loss of the T1R2-encoding gene occurred in the age of the dinosaurs (Baldwin et al., 2015). This adaptation is reasonable since dinosaurs had diets rich in amino acids but lacking sugars, meaning that the T1R2 receptor served no purpose (Baldwin et al., 2015). However, if birds no longer possess the T1R2 protein and therefore cannot form a functioning T1R2-T1R3 heterodimer, how does this result explain how species that primarily feed on carbohydrate-rich nectar, like hummingbirds, detect sweet tastes?
T1R Receptor Mutations in Hummingbirds
It turns out that, in hummingbirds, the T1R1-T1R3 receptor, usually responsible for detecting savoury tastes, has mutated to respond to sweet tastes instead (Baldwin et al., 2015). When testing the hummingbird taste receptors in response to sugars and amino acids, the T1R1-T1R3 heterodimer responded to several carbohydrates, including sucrose, fructose, and glucose. Although, neither subunit responded individually, suggesting that receptor function is dependent on the formation of the heterodimer. In contrast, the T1R1-T1R3 receptors of chickens and chimney swifts, the hummingbird’s closest relative, detected alanine and serine proteins but failed to detect carbohydrates (Figure 2) (Baldwin et al., 2015).
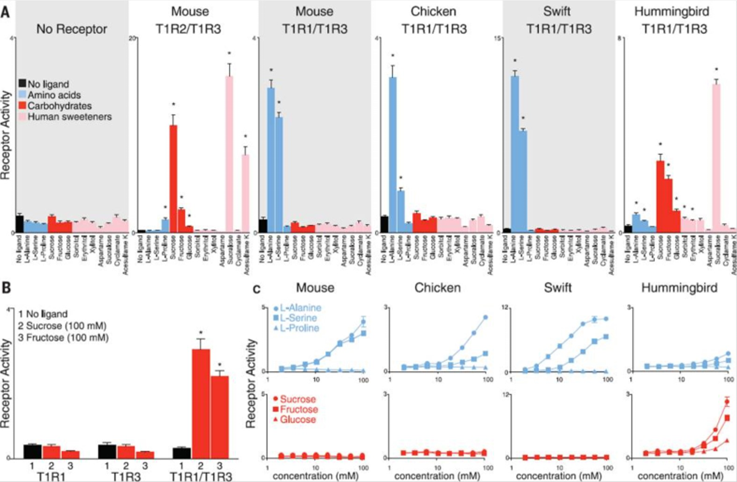
To further understand the specific mutations in the hummingbird T1R1-T1R3 receptor, which allowed for sugar detection, researchers created protein chimeras with parts from the hummingbird receptors and parts from the chicken receptors (Figure 3). Focusing on the effects of changing the ligand-binding domain, they introduced the venus flytrap domain of chicken T1R3 into hummingbird T1R3. This modification resulted in the hummingbird heterodimer being receptive to amino acids instead of sugars, as in the case of chickens (Baldwin et al., 2015). A second protein chimera consisted of a 109 amino acid sequence from hummingbird T1R3 being introduced into the chicken T1R3 venus flytrap domain, resulting in restored sucrose responses in the chicken receptor (Baldwin et al., 2015). When further analyzing this region, 19-non-consecutive amino acids were identified, which, when all introduced together in a third chimera, were sufficient to restore sugar sensitivity in the chicken T1R3, suggesting that mutation of these 19 amino acids is responsible for the hummingbird’s ability to perceive sweet tastes (Baldwin et al., 2015).
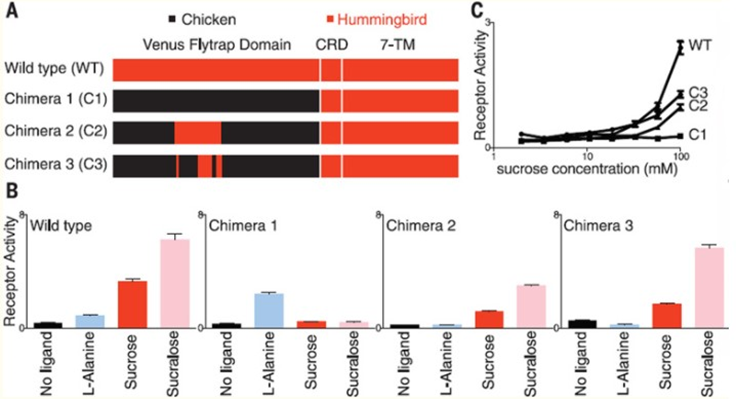
In addition to finding mutations in the hummingbird T1R3 subunit, researchers concluded that mutations must have also occurred in the T1R1 subunit. This is because a heterodimer made from chicken T1R1 and hummingbird T1R3 resulted in a preference for amino acids, meaning that it is not the T1R3 subunit acting alone to produce sweet taste responsiveness (Baldwin et al., 2015). Overall, this finding proposes that the mutations resulting in the evolution of carbohydrate detection occurred in both subunits of the hummingbird T1R1-T1R3 receptor. Additionally, sites on both subunits that experienced mutations were found to be under positive selection, meaning that they have proven to be evolutionary beneficial for hummingbirds (Antinucci & Risso, 2017). This discovery would make sense because while a great majority of birds are competing for insects and other protein-rich foods, hummingbirds are part of a small group, which can feed on nectar and sweets with little competition, thereby increasing their chances of survival.
Hummingbirds are just one example of a species whose sensory capabilities deviated from the norm to grant an additional evolutionary benefit. In this case, their unique sense of taste allows them to avoid competing with closely related species with which they would have shared similar diets if not for the occurrence of the mutation.
Heat Vision in Snakes
While snakes are infamously known to have very poor eyesight, as they can generally see shapes but not detail, they can still successfully track down and hunt their prey. Typically, snakes search for prey late at night or very early in the morning before sunrise, which leaves them with little exposure to sunlight, thereby inhibiting their vision. Consequently, snakes must rely on their unique somatosensory system to detect the presence and motion of other creatures.
Pit Organs
A snake’s ability to sense the radiant heat of its surroundings depends on its anatomical structure, which is why only certain species of snakes use this technique in their search for prey. Precisely, most boa constrictors, many pythons, and all pit vipers have evolved, alongside each other (parallel evolution), to form unique holes in their faces, called pit organs, which allow them to detect infrared thermal radiation (700 nm – 1 mm) from warm bodies up to a meter away (Fang, 2010). The placement and structure of this heat sensory organ, however, differ between species of snakes. Indeed, pit vipers possess a loreal pit organ situated between the lateral eye and the nostrils on either side of their face (Figure 4. A), while boas and pythons have multiple labial pit organs located on the upper or lower lip (Figure 4. B).
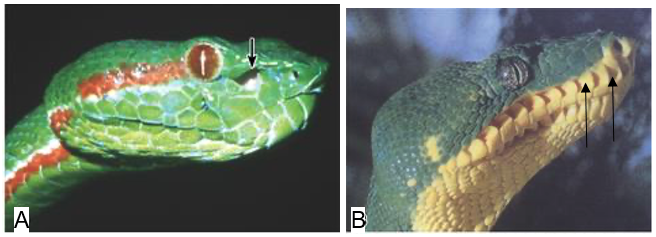
Furthermore, the morphology of the pit organs varies significantly between the pit vipers on the one hand and the boas and pythons on the other. In fact, a pit viper’s pit organ is composed of an inner chamber and an outer chamber separated by a thin membrane, which is responsible for detecting infrared thermal radiation entering the pit (Figure 5. A) (Goris, 2011). Although the inner chamber cannot be seen outside the snake’s body, it communicates with the exterior air through a pore between the pit organ and the eye (Figure 5. A). Conversely, the pit organs in boas and pythons are composed of a single cavity centred at a rostral (toward the head), infralabial (below the lip) or supralabial (above the lip) scale. Contrary to the pit viper’s pit organ, boas and pythons lack an inner chamber and pit membrane. Instead, boas and pythons contain infrared receptors in the bottom of their cavity, called the pit fundus, which is exposed to the exterior and serves as an infrared retina in these species of snakes (Figure 5. B) (Goris, 2011). Thus, while vipers have a heat-sensitive pit membrane that stretches across the opening of their pit organ, boas and pythons possess heat-sensitive receptors that line the inside of their pits. In both cases, however, the member responsible for sensing infrared radiation is covered with blood vessels, which transfer oxygen to the heat receptors to help cool them down (Goris, 2011). The absence of these blood vessels would limit the accuracy of a serpent’s infrared vision, as the heat receptors would take longer to cool down, leaving behind a trace of heat even after a creature has left their sight.
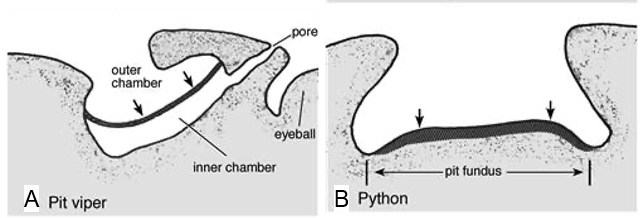
TRPA-1 Calcium Ion Channels
Multidisciplinary research suggests a strong relationship between the presence of transient receptor potential ankyrin 1 (TRPA-1) calcium (Ca2+) ion channels in snakes’ pit organs and infrared receptibility. Specifically, snakes lacking pit organs, and consequently showing no disparity in TRPA-1 expression at different conditions, cannot sense infrared radiation. On the other hand, snake species which possess pit organs, such as, pit vipers, pythons, and boas can use heat vision when pursuing their prey and identifying other creatures. Moreover, studies show that snakes’ TRPA-1 shows 63% identity with mammalian TRPA-1, which is activated by allyl isothiocyanate (AITC) (Gracheva et al., 2010). Nevertheless, the serpent calcium ion channel was found to be more sensitive to heat than AITC and is therefore activated at higher temperatures (Figure 6. A). For instance, in the case of pit vipers, the activation threshold is 27.6 ± 0.9 ℃ (Figure 6. B), indicating that any creature with a body temperature higher than the threshold will be bright in the eyes of the pit vipers (Gracheva et al., 2010). It follows that as an animal’s body temperature increases, the activation of the TRPA-1 calcium ion channels in a snake’s pit organs increases, causing the creature to appear more vivid to the snake.

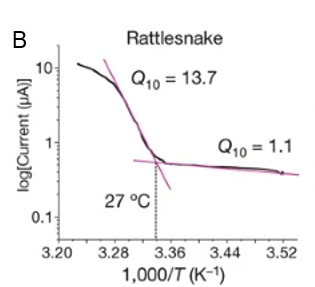
The Pursuit of Prey
As heat-sensing snakes have specialized pit organs that are sensitive to changes in heat in their environments, they use this sixth sense to accurately identify and track down their prey before eating them. Indeed, while pit vipers, boas, and pythons are cold-blooded while their prey, typically rodents, small mammals, or birds, are warm-blooded (Kelber, 2019). Therefore, snakes that possess pit organs can analyze the body temperature of different creatures in the form of infrared thermal radiation, allowing them to correctly identify their prey. In particular, if an unknown creature’s body temperature surpasses the 27.6 ± 0.9 ℃ threshold temperature and is small enough, the snake will perceive the emitted infrared radiation and begin its systematic attack. To further ensure accurate readings, snakes hunt their prey when it is cold late at night and thermoregulate their body through specific breathing patterns, which makes the difference in temperature between themselves and their prey more distinct (Kelber, 2019).
Moreover, the attacking process is different between species of heat-sensing snakes, as pit vipers are venomous, but boas and pythons are not. In the case of pit vipers, the serpents first use camouflaging tactics to hide and blend in with their surrounding environments (Newman & Hartline, 1982). The venomous snake then searches for a bright creature, indicating the presence of prey. Once the prey approaches the pit viper and is within striking range, the snake bites it but does not follow it immediately, as the prey will soon be immobilized. To track the animal down, vipers use their heat vision to follow the trail of venom, which emits a significant amount of infrared radiation. Additionally, the venom injection makes the prey dribble urine, leaving behind a strong scent that the pit viper can follow, using their sense of taste and smell, if the venom traces cool down (Newman & Hartline, 1982). Finally, when the snake finds its dead prey, it can effortlessly consume it. Similarly, boas and pythons camouflage in their environment and use their heat vision to identify and locate potential prey before attacking. However, since these snake species are not venomous, they must rely on different hunting tactics, such as constricting and suffocating their prey. This quick and effective attack method is performed as snakes coil around their prey with either their underside or dorsal side against the prey animal (Newman & Hartline, 1982). These serpents hold the tight position until their prey cannot breathe or lacks blood flow to the heart. Ultimately, although the techniques used to kill their prey may differ between heat-sensing snakes, the method used to identify and locate their prey is identical.
Antenniform Legs of Whip Spiders
Whip spiders (or Amblypygi) are a nocturnal species sharing morphological characteristics with other arachnids like spiders, scorpions and Opiliones (Sinakevitch et al., 2021). These spiders live in the dense rainforests in tropical and subtropical regions (Bingman et al., 2017). Much like other arachnids, whip spiders have eight legs. However, their first pair of legs is not used for movement; instead, their front legs are used as “feelers” (or antenniform legs) that help them navigate the world around them and aid them with prey detection and courtship (Sinakevitch et al., 2021).
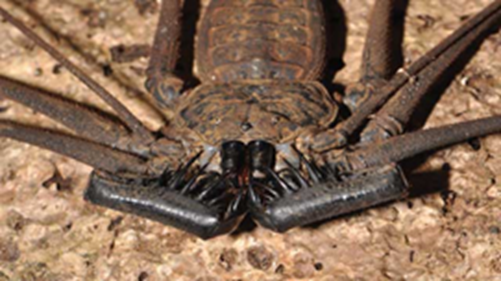
The large “whips” on the spiders can grow to be up to three to six times their body size (Rayor & Taylor, 2006). These front appendages can move in a complete circle around them, detecting everything ahead, below and above them. Due to their environment and nocturnal nature, navigation via visual cues becomes difficult. Their structurally complex environment consists of uneven surfaces and dense vegetation, depriving them of terrestrial stimuli and celestial cues (Bingman et al., 2017). Furthermore, amblypygids have relatively small eyes, and their visual pathways are not advanced compared to other arachnids, making them not likely to rely on visual cues for navigation (Bingman et al., 2017; Sinakevitch et al., 2021).
In 1974, Beck and Görke were the first to report that these creatures had an outstanding ability to navigate their way back home, shortly before dawn, after hunting on vertical surfaces of tree trunks (Bingman et al., 2017). A similar experiment performed by Hebets et al. built upon Beck and Görke’s findings where they displaced the spiders 4.5 meters away from their trees and found that they routinely returned home (Bingman et al., 2017). The researchers also found that the test subjects returned home consistently up to a displacement of eight metres (the journeys often taking more than one night to complete). The question that remains unanswered is how exactly their front legs have evolved to become such intricate perceptional tools.
Mechano- and Chemoreceptors
A whip spider’s antenniform legs are covered with a rich array of sensory organs composed of mechanoreceptors and chemoreceptors (Figure 8) (Foelix et al., 2002). These sensilla allow them to detect odours and the objects surrounding them (Sinakevitch et al., 2021). Studies of the sensilla on their legs indicate that their chemoreceptors are similar to olfactory sensilla present on the antennae of different insects (Sinakevitch et al., 2021). Other experiments have also shown that the chemosensory sensilla houses 20 – 45 olfactory neurons, and together with their connection to sensory axons, supply the spider’s central nervous system (Sinakevitch et al., 2021). Their legs also contain several large interneurons that allow them to receive mechanosensory input from 700 – 1500 tarsal bristles (found on the legs and feet of many insects) (Foelix et al., 2002). The combination of these sensory organs allows them to receive information about their surroundings to help them navigate.
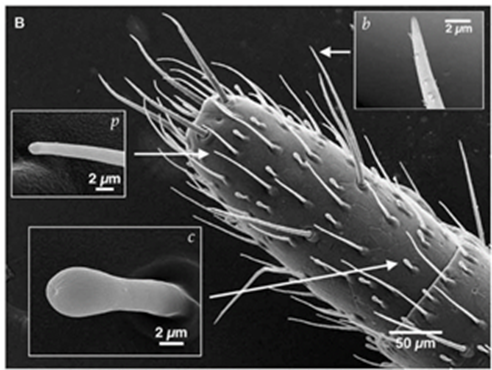
Olfaction in Whip Spiders
In 2000, Hebets and Chapman showed that the antenniform leg nerve showed neuronal responses to more than 40 volatile organic compounds, indicating that the species has an advanced sense of smell (Sinakevitch et al., 2021). Olfactory neurons send axons through the leg nerve into the neuromere of the central nervous system (Sinakevitch et al., 2021). These neurons then terminate in the primary olfactory glomeruli (cluster of nerve endings), ascend to the brain, and terminate in the mushroom body on a secondary set of glomeruli (which is unique when compared to typical olfactory perception in other animals) (Sinakevitch et al., 2021). The compounds they perceive indicate their position and enable them to relocate and find their way back to their shelter.
A previous experiment performed displayed their dependence on olfactory cues. To do so, they identified and sampled a scent near the location of their shelters and altered the position of the spiders (Wiegmann et al., 2019). The subjects were then able to reorientate their position within a two-week testing period. Upon repeating the experiment, they decided to perform a different test by amputating the front legs of the spiders. The group of researchers found that the number of spiders that found their way to shelter significantly decreased. The inferred results demonstrated how odour cues support near-distance relocation for whip spiders specifically in relation to their antenniform legs.
This ability to sense olfactory cues, however, is not commonplace or well known in spiders (Sinakevitch et al., 2021). For example, some researchers suggest that spiders can perceive pheromones, but no olfactory sensilla has been found in spiders due to their lack of glomeruli (which is how vertebrates perceive smell) (Sinakevitch et al., 2021). This unique sensory system of chemosensation is shared with scorpions who also have chemosensory organs that appear fan-like and allow them to probe substrates and send chemical identifying signals to clusters of glomeruli (Sinakevitch et al., 2021). These olfactory cues allow them to find food and also defend themselves against different dangers.
Canine Olfaction
Canines have an exceptional and unique sense of smell. They are equipped with an olfactory system that is far more capable than most land mammals. Not only is it more sensitive, but it can also pinpoint the desired odour amid the constantly incoming stimuli. Their olfactory system’s heightened and unique abilities are enabled by its two central parts: the main olfactory epithelium and the vomeronasal organ.
The Olfactory Epithelium
The central olfactory epithelium’s function is supported by: the nasal cavity, the olfactory receptors, and the olfactory bulb (Figure 9) (Kokocińska-Kusiak et al., 2021). When a canine sniffs, air enters through the nares, where the nasal cavity has two chambers containing three turbinates each: nasoturbinate, maxilloturbinate, and ethmoturbinate (Figure 10) (Jenkins et al., 2018). Found in all mammals (Hillenius, 1992), turbinates are nasal bones that cleanse and humidify the air entering the nostrils (Stanford Health Care, 2017). Following inhalation, air first flows to the maxillotrubinate, also known as the respiratory epithelium (Kokocińska-Kusiak et al., 2021), where some olfactory sensors are present (Jenkins et al., 2018). Then, air flows into the ethmoturbinate, otherwise called the olfactory epithelium, where the detection of scent occurs and where the air is also set on a path to the lungs. Odorants are captured in a mucous layer maintained by the Bowman’s glands which are embedded in the olfactory epithelium (Jenkins et. al, 2018).
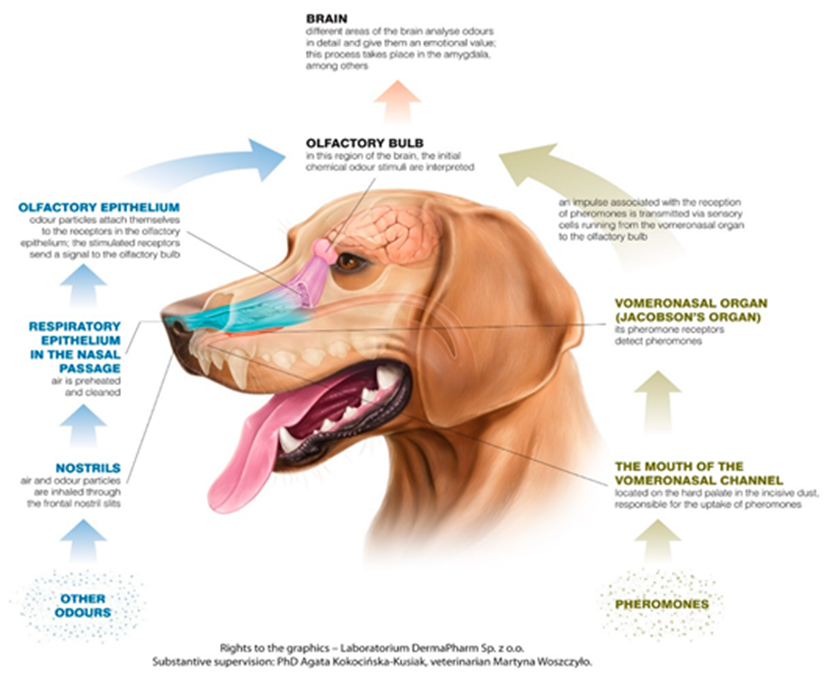
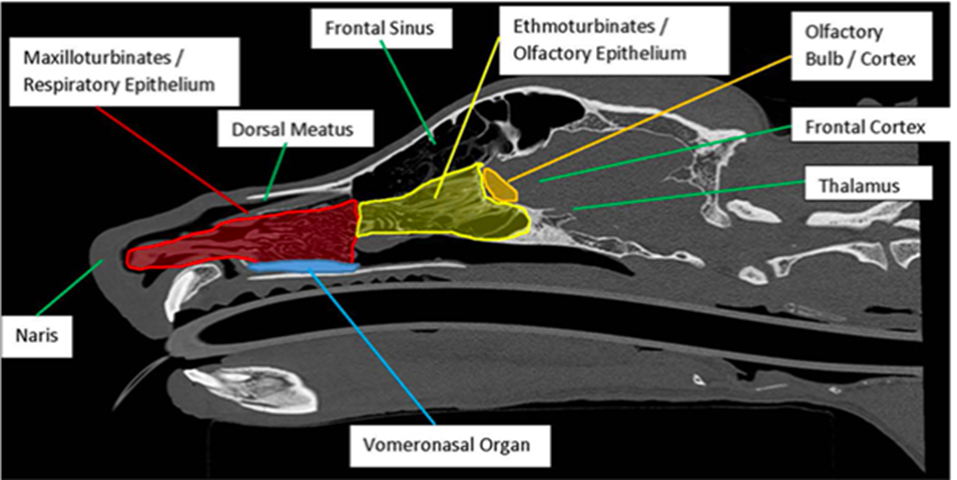
The olfactory epithelium contains the bulk of the olfactory receptor cells (ORCs) required for odour detection. These cells extend directly to the part of the brain, referred to as the olfactory bulb, where the initial chemical stimuli are interpreted (Kokocińska-Kusiak et al., 2021). Varying by breed and genetics, canine nasal cavities contain hundreds of millions of olfactory receptors (OR). However, each ORC only has one type of OR for hormones or neurotransmitters (Jenkins et al., 2018). Furthermore, olfactory receptor cells have cilia embedded with projections that bind to odorants entering the extracellular matrix post-inhalation. The canine olfactory receptor cells have more than four times the number of cilia per ORC as in humans, contributing to their heightened sense (Jenkins et al., 2018). Additionally, ORC cilia also have intracellular projections, which are coupled to a guanine nucleotide-binding protein (G-protein), composed of alpha, beta and gamma subunits (Jia et al., 2014). When the appropriate odorant binds to the extracellular G-protein receptor, a conformational change occurs, activating the intracellular G-protein (InterPro, n.d.). This activation, in turn, promotes the release of the G-protein alpha-subunit, which activates adenylyl cyclase, an enzyme that helps convert ATP to cyclic-AMP [Bowen, n.d.]. The cAMP then stimulates protein kinase A, amplifying the stimuli signal and activating the sodium gated channels (Yan et al., 2016). The resultant action potential propagates the signal to the olfactory bulb where different stimuli are recognized by the unique combination of activated ORs (Jenkins et al., 2018).
The Vomeronasal Organ
The vomeronasal organ (VNO) is located between the nasal and oral cavities, directly above the roof of the canine’s mouth (Figure 10) (Kokocińska-Kusiak et al., 2021). The VNO detects non-volatile odorants, such as pheromones, which evoke reproductive and social behavioural or physiological reactions (Dennis et al., 2003). However, the VNO’s distinction from the olfactory epithelium (nose) is debated and not yet clear enough (Kokocińska-Kusiak et al., 2021). It is believed that both parts receive pheromones but the VNO also receives low-volatility liquid-borne stimuli (Adams & Wiekamp, 1984). Moreover, it is also suggested that unique/individual behavioural responses could be the result of the activation of both parts of the olfactory system (Dennis et al., 2003).
As discussed, the canine’s olfactory system is far more superior to that of most mammals. Canine’s olfactory epithelium’s greater surface area and the greater number of olfactory receptors allow for the recognition of a broader spectrum of stimuli (Jenkins et al., 2018). Additionally, the more sensitive ORs make it possible to precisely target and distinguish between stereoisomeric structures of chemical stimuli (Jenkins et al., 2018).
Discussion
Through mutation, the taste system of hummingbirds has deviated from closely-related bird species, gaining the ability to sense sweet rather than savoury tastes, allowing them to adopt a diet mainly consisting of nectar. This mutation promotes an evolutionary advantage, allowing them to avoid competition for resources with most other bird species that feed on insects, seeds, and plants. Further understanding mutations to GPCRs, such as this one, can have implications in the pharmaceutical industry, where GPCRs are important drug targets because of their vast array of functions in the body. Moreover, in the case of pit vipers, boas, and pythons, the ability to perceive the environment in complete darkness by detecting emitted infrared thermal radiation provides fascinating information for future engineering technologies. Researchers have discovered how to mimic such technology by analyzing a snake’s pit organs, which are responsible for their heat-sensing capabilities. The acquired knowledge can have significant implications in the robotics industry and the field of cameras, telescopes, and night-vision goggles. Furthermore, due to the need to navigate deeply wooded and uneven terrains, the nocturnal whip spiders developed long antenniform legs that circle their bodies, providing mechanosensory and chemosensory information about their surroundings. By analyzing their methods of sensation, researchers can better understand chemosensation, specifically olfaction, by identifying the processes that these systems undergo. This data can then be applied to the treatment and better understanding of the molecular events that underlie taste and smell disorders (Spielman, 1998). Finally, canine’s extraordinary olfactory sensitivity gives them the ability to sniff out specific target chemical signals. They are able to track odours in numerous crowded environments, detecting the faintest signals. Studies have demonstrated the various capabilities of these hypersensitive scanners, including the detection of drugs, explosives, and diseases. Their ability to identify and distinguish the presence of volatile organic molecules equips them with a nose capable of sniffing out diseases like lung cancer and covid-19 (Jendrny et al., 2021; Feil et al., 2021). Beyond that, canines can not only be used for biomedical detection, but their olfactory system can be mimicked to produce “disease-sniffing” devices (Chandler, 2021).
Conclusion
To conclude, as different species face unique environmental pressures due to the ecological niches in which they live, many animals have developed new sensory capabilities, through evolution, to adapt to their particular living conditions. For instance, hummingbirds have undergone mutations that allow them to sense sweet tastes instead of savoury ones, pit vipers, boas, and pythons have developed pit organs that enable them to sense infrared thermal radiation to facilitate the search for prey in the absence of sunlight, whip spiders have evolved ways to navigate their terrain using mechano- and chemosensation, and canines developed a hyperselective nose caple of sniffing out target molecules. Ultimately, closely analyzing these exceptional senses found in the animal kingdom has led to the development of cutting-edge technology in various industries and innovative research in several science disciplines.
References
Adams, D. R., & Wiekamp, M. D. (1984). The canine vomeronasal organ. J Anat, 138 ( Pt 4)(Pt 4), 771-787.
Ahmad, R., & Dalziel, J. E. (2020). G Protein-Coupled Receptors in Taste Physiology and Pharmacology [Review]. Frontiers in Pharmacology, 11. https://doi.org/10.3389/fphar.2020.587664
Antinucci, M., & Risso, D. (2017). A Matter of Taste: Lineage-Specific Loss of Function of Taste Receptor Genes in Vertebrates [Mini Review]. Frontiers in Molecular Biosciences, 4. https://doi.org/10.3389/fmolb.2017.00081
Baldwin, M. W., Toda, Y., Nakagita, T., O’Connell, M. J., Klasing, K. C., Misaka, T., Edwards, S. V., & Liberles, S. D. (2014). Evolution of sweet taste perception in hummingbirds by transformation of the ancestral umami receptor. Science, 345(6199), 929-933. https://doi.org/doi:10.1126/science.1255097
Basith, S., Cui, M., Macalino, S. J. Y., Park, J., Clavio, N. A. B., Kang, S., & Choi, S. (2018). Exploring G Protein-Coupled Receptors (GPCRs) Ligand Space via Cheminformatics Approaches: Impact on Rational Drug Design [Review]. Frontiers in Pharmacology, 9. https://doi.org/10.3389/fphar.2018.00128
Bates, M. (2017, December 1). Meet the Arachnid That May Add a New Chapter to the Book on Sensory Biology. TheScientist. https://www.the-scientist.com/notebook/meet-the-arachnid-that-may-add-a-new-chapter-to-the-book-on-sensory-biology-30173
Bingman, V. P., Graving, J. M., Hebets, E. A., & Wiegmann, D. D. (2017). Importance of the antenniform legs, but not vision, for homing by the neotropical whip spider Paraphrynus laevifrons. Journal of Experimental Biology, 220(5), 885-890. https://doi.org/10.1242/jeb.149823
Bowen, R. (n.d.). Adenylyl Cyclase. Colorado State University. Retrieved November 5, 2021, from https://rabowen.org/hbooks/pathphys/topics/cyclase.html
Chandler, D. L. (2021, February 17). Toward a disease-sniffing device that rivals a dog’s nose. MIT News. Retrieved November 5, 2021, from https://news.mit.edu/2021/disease-detection-device-dogs-0217
Dennis, J. C., Allgier, J. G., Desouza, L. S., Eward, W. C., & Morrison, E. E. (2003). Immunohistochemistry of the canine vomeronasal organ. J Anat, 203(3), 329-338. https://doi.org/10.1046/j.1469-7580.2003.00190.x
Fang, J. (2010). Snake infrared detection unravelled. Nature. https://doi.org/10.1038/news.2010.122
Feil, C., Staib, F., Berger, M. R., Stein, T., Schmidtmann, I., Forster, A., & Schimanski, C. C. (2021). Sniffer dogs can identify lung cancer patients from breath and urine samples. BMC Cancer, 21(1), 917. https://doi.org/10.1186/s12885-021-08651-5
Foelix, R., Troyer, D., & Igelmund, P. (2002). Peripheral synapses and giant neurons in whip spiders. Microscopy Research and Technique, 58(4), 272-282. https://doi.org/https://doi.org/10.1002/jemt.10136
Goris, R. C. (2011). Infrared Organs of Snakes: An Integral Part of Vision. Journal of Herpetology, 45(1), 2-14, 13. https://doi.org/10.1670/10-238.1
Gracheva, E. O., Ingolia, N. T., Kelly, Y. M., Cordero-Morales, J. F., Hollopeter, G., Chesler, A. T., Sánchez, E. E., Perez, J. C., Weissman, J. S., & Julius, D. (2010). Molecular basis of infrared detection by snakes. Nature, 464(7291), 1006-1011. https://doi.org/10.1038/nature08943
Hillenius, W. J. (1992). The Evolution of Nasal Turbinates and Mammalian Endothermy. Paleobiology, 18(1), 17-29. http://www.jstor.org/stable/2400978
InterPro. (n.d.). G-protein alpha subunit, group I. ELIXIR. Retrieved November 5, 2021, from https://www.ebi.ac.uk/interpro/entry/InterPro/IPR001408/
Jendrny, P., Twele, F., Meller, S., Osterhaus, A. D. M. E., Schalke, E., & Volk, H. A. (2021). Canine olfactory detection and its relevance to medical detection. BMC Infectious Diseases, 21(1), 838. https://doi.org/10.1186/s12879-021-06523-8
Jenkins, E. K., DeChant, M. T., & Perry, E. B. (2018). When the Nose Doesn’t Know: Canine Olfactory Function Associated With Health, Management, and Potential Links to Microbiota [Review]. Frontiers in Veterinary Science, 5. https://doi.org/10.3389/fvets.2018.00056
Jia, H., Pustovyy, O. M., Waggoner, P., Beyers, R. J., Schumacher, J., Wildey, C., Barrett, J., Morrison, E., Salibi, N., Denney, T. S., Vodyanoy, V. J., & Deshpande, G. (2014). Functional MRI of the Olfactory System in Conscious Dogs. PLOS ONE, 9(1), e86362. https://doi.org/10.1371/journal.pone.0086362
Kelber, A. (2019). Infrared Imaging: A Motion Detection Circuit for Rattlesnake Thermal Vision. Current Biology, 29(11), R403-R405. https://doi.org/10.1016/j.cub.2019.04.043
Kokocińska-Kusiak, A., Woszczyło, M., Zybala, M., Maciocha, J., Barłowska, K., & Dzięcioł, M. (2021). Canine Olfaction: Physiology, Behavior, and Possibilities for Practical Applications. Animals (Basel), 11(8). https://doi.org/10.3390/ani11082463
Newman, E. A., & Hartline, P. H. (1982). The Infrared “Vision” of Snakes. Scientific American, 246(3), 116-127. http://www.jstor.org/stable/24966551
Rayor, L. S., & Taylor, L. A. (2006). Social Behavior in Amblypygids, and a Reassessment of Arachnid Social Patterns. The Journal of Arachnology, 34(2), 399-421, 323. https://doi.org/10.1636/S04-23.1
Rowland, H. M., Rockwell Parker, M., Jiang, P., Reed, D. R., & Beauchamp, G. K. (2015). Comparative Taste Biology with Special Focus on Birds and Reptiles. In R. L. Doty (Ed.), Handbook of Olfaction and Gustation (pp. 957-982). Wiley-Blackwell. https://doi.org/https://doi.org/10.1002/9781118971758.ch43
Sinakevitch, I., Long, S. M., & Gronenberg, W. (2021). The central nervous system of whip spiders (Amblypygi): Large mushroom bodies receive olfactory and visual input. Journal of Comparative Neurology, 529(7), 1642-1658. https://doi.org/https://doi.org/10.1002/cne.25045
Spielman, A. I. (1998). Chemosensory Function and Dysfunction. Critical Reviews in Oral Biology & Medicine, 9(3), 267-291. https://doi.org/10.1177/10454411980090030201
Stanford Health Care. (2017, September 11). Turbinate Reduction. Stanford Medicine. Retrieved November 5, 2021, from https://stanfordhealthcare.org/medical-treatments/n/nasal-surgery/types/turbinate-reduction.html
Treesukosol, Y., Smith, K. R., & Spector, A. C. (2011). The functional role of the T1R family of receptors in sweet taste and feeding. Physiology & Behavior, 105(1), 14-26. https://doi.org/https://doi.org/10.1016/j.physbeh.2011.02.030
Wiegmann, D. D., Hebets, E. A., Gronenberg, W., Graving, J. M., & Bingman, V. P. (2016). Amblypygids: Model Organisms for the Study of Arthropod Navigation Mechanisms in Complex Environments? [Perspective]. Frontiers in Behavioral Neuroscience, 10. https://doi.org/10.3389/fnbeh.2016.00047
Yan, K., Gao, L. N., Cui, Y. L., Zhang, Y., & Zhou, X. (2016). The cyclic AMP signaling pathway: Exploring targets for successful drug discovery (Review). Mol Med Rep, 13(5), 3715-3723. https://doi.org/10.3892/mmr.2016.5005
Yarmolinsky, D. A., Zuker, C. S., & Ryba, N. J. P. (2009). Common Sense about Taste: From Mammals to Insects. Cell, 139(2), 234-244. https://doi.org/10.1016/j.cell.2009.10.001