ABSTRACT
Gonium pectorale is a small 16-celled photosynthetic algae that provides a refreshing outlook on the evolution of green algae and the concept of modularity. Each individual cell operates with its own two flagella, which are propulsion appendages, and an eyespot, an optical light sensor. Without a central nervous system, signals captured by cells of Gonium pectorale are processed separately, and the variation of individual responses contributes to complex behavioral patterns, namely its methods of helical propulsion and phototaxis, or light-influenced movement. For such a small and seemingly simple organism, Gonium pectorale structures are surprisingly complex, and create movement using elaborate flagellar motion on behalf of individual cells. These systems have been adapted over millennia of evolution, which we can contextualize by inspecting Gonium pectorale’s evolutionary tree. These traits are indicative of Gonium’s position as a transitional organism in the volvocine lineage, between unicellular and larger multicellular volvocine organisms. In this paper, we will investigate the depth of Gonium pectorale’s physical structures and behavioral patterns and draw comparisons to mechanical and optical concepts to better understand how they apply to the anatomy and behavior of Gonium pectorale.
INTRODUCTION
Only in recent human history have we had the liberty of knowledge to understand how much we do not know. Consider that before humanity knew that bacteria, microorganisms, or even atoms existed, we had many basic understandings of how the world works that were incorrect. Today, being an engineer or a scientist means acknowledging that there is still an incredible amount of this world that we are yet to know or understand, and plenty that we do not understand correctly. Even organisms that we believe we understand turn out to be more complex than we could imagine, and such is the case with Gonium pectorale (Figure 1).
Gonium is a species of algae of the order Chlamydomonadales, also known as Volvocales. Itis a colonial organism, with colonies comprised either of 4, 8, 16, or 32 biflagellate cells of the same size. The most studied form of this organism is the 16-celled Gonium pectorale, whose cells form two concentric squares––an inner square with four component cells and an outer square with twelve, as shown in Figure 2 (De Maleprade et al., 2020). The cells form a flat plate with no anterior-posterior differentiation (Pennak, 1978).
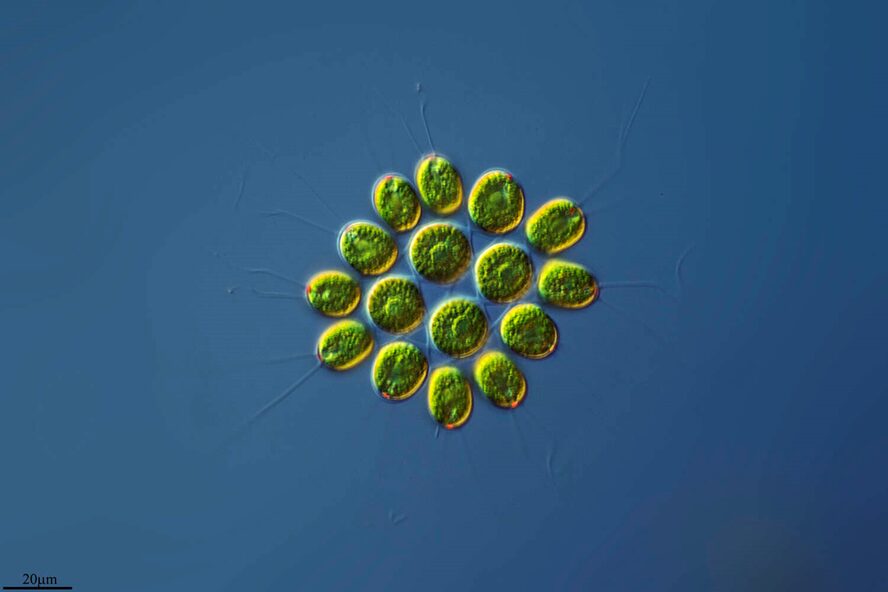
Figure 1: 16-cell colony of Gonium pectorale colony, pictured in lake water. Notice the faint flagella coming from each cell and the yellow-orange eyespots, currently visible on the 12 outer cells, but hidden on the four inner cells by the angle of the photo (Proyecto Agua, 2018).
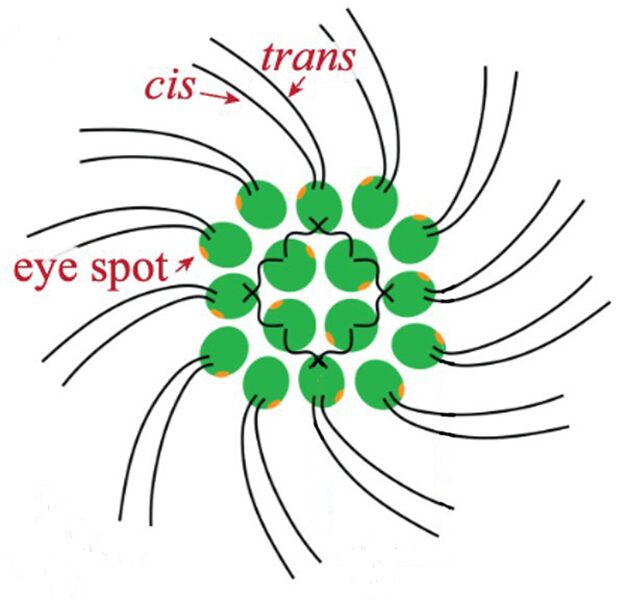
Figure 2: General structure of a Gonium pectorale colony.Note the 16 contributing cells in two concentric squares of 4 and 12 cells respectively, each cell containing cis and trans flagellum and an eyespot (De Maleprade et al., 2020).
As a species of algae, Gonium is a photoautotroph, a photosynthetic organism. It derives the energy it needs to develop, survive, and maintain itself from light provided by the sun. Colonies of Gonium have adapted and evolved to efficiently satiate this need for light through a strong light detection eyespot, and robust behavioral reorientation using flagellar movement in response to light detection. Gonium’s means of phototactic movement is itself adapted to the colony’s unique shape, presenting in a fascinating helical propulsion pattern.
The structure of Gonium is a marker of its remarkable position in the evolutionary lineage of the order of the Chlamydomonadales. It is closely related both to the unicellular Chlamydomonas and to the multicellular Volvox, which can contain up to 60,000 cells. For this reason, Gonium is often seen as a species of great interest when studying the evolutionary transition from unicellularity to multicellularity. Indeed, the cells which make up Gonium colonies are comparable to Chlamydomonas.Both cells possess two flagella, although their orientation differs between Gonium cells and Chlamydomonas. Both are of similar shape, Gonium cells are “ovoid to pyriform” according to Mueller, 1773, while Chlamydomonas cells are “cup-shaped” according to Guiry et al., 2007. Finally, both rely on an eyespot to sense light and sequentially direct orientation and motility.
The evolutionary proximity of these two species is especially accentuated by the fact that colonies of Gonium do not possess a central nervous system. In Gonium, there is no unified system collecting, processing, organizing, and reacting to information captured by the many eyespots of the colony. Rather, the movement of this species can be described as the result of the separate action of individual cells, each of which behaves comparably to an independent Chlamydomonas cell. Sixteen pilots issue their own commands to fly one plane in remarkable coordination.
Gonium’s motility highlights one of the species’ interesting features with potential comparative applications in robotics, computing, and other technological fields: modularity. As explained by Melo et al. (2016), “A system is modular if it can be divided into multiple sets of strongly interacting parts that are relatively autonomous with respect to each other.” This is certainly the case for Gonium, whose cells, while acting almost independently of each other, contribute to the overall movement of the colony to permit accurate phototaxis. This kind of robust and coordinated structure can be hard to mimic in human-made technology, where hierarchical system structures are much more common. Gonium’s modularity allows for precise control from specific cells and cuts out the leader, a possible point of failure present in hierarchical structures. Robotics can find advantages in the modularity of systems that could decrease failure rate, and achieving more modular computing could provide for more efficient systems in the future.
However, this modularity means Gonium must orchestrate coordinated action, which can be difficult. To understand how design solutions integrated into Gonium’s anatomy and behavior allow its modularity to be compatible with the coordinated nature of its motility and phototaxis, we will be examining the structural components of individual cells of Gonium pectorale, and then delving deeper into the behavioral systems that contribute to the success and survival of the colony by permitting accurate phototaxis.
ESSENTIAL PHYSICAL CHARACTERISTICS INVOLVED IN GONIUM’S MOTILITY
Gonium’s unique morphology is an essential contributor to the coordinated nature of its movement. From the structure of individual components within cells, to individual cellular properties and features, to the overall form of the colony, this organismis equipped with the biological tools necessary to detect and move towards sources of light. These tools will be explored in the following portions of this article.
Colony Structure
Each cell within a Gonium colony has two flagella that are 30-40 μm in length (De Maleprade et al., 2020). The flagella of the outer twelve cells point outwards, taking the shape of a pinwheel (Moore, 1916) as seen in Fig. 1. The flagella are slightly offset, tilted at an angle of roughly 30°; this angle allows the colony to generate thrust and rotation. The central flagella only generate thrust (De Maleprade et al., 2020).
Colonies are characterized by an extracellular matrix (ECM) consisting of three main zones. The innermost zone surrounding the membrane of each cell is a tripartite boundary comprised of a thick fibrillar inner layer, an electron-lucent layer, and a thinner fibrous outer layer. The outermost layer of Gonium’s ECM consists of a loose fibrillar material. Swilley (2018) highlights the importance of the ECM in maintaining intercellular adhesion within Gonium. Specifically, the tripartite layer surrounding cells and the presence of certain proteins in the ECM are identified as the components responsible for ensuring the integrity of the colony’s structure. Indeed, species of Gonium in which the tripartite wall is eliminated adopt a unicellular morphology.

While little research exists on the subject, in describing the connections and interactions between a colony’s cells, it is again necessary to emphasize that Gonium does not present a central nervous system (De Maleprade et al., 2020). As will be highlighted in the next section, properties allowing cellular coordination are built directly into elements of cellular anatomy.
Flagella
Flagella are found in a vast array of living organisms, both prokaryotic and eukaryotic. They can be found in the largest of animals and in some of the smallest unicellular organisms. Their presence in virtually all types of motile organisms suggests that flagella, as well as cilia, are ancient mechanisms for motility used by some of the earliest forms of life (Wan, 2018). This section will discuss the flagella of Gonium and its role in the motility of a Gonium colony. In order to discuss flagellar motion, the structure of the flagella must first be established.
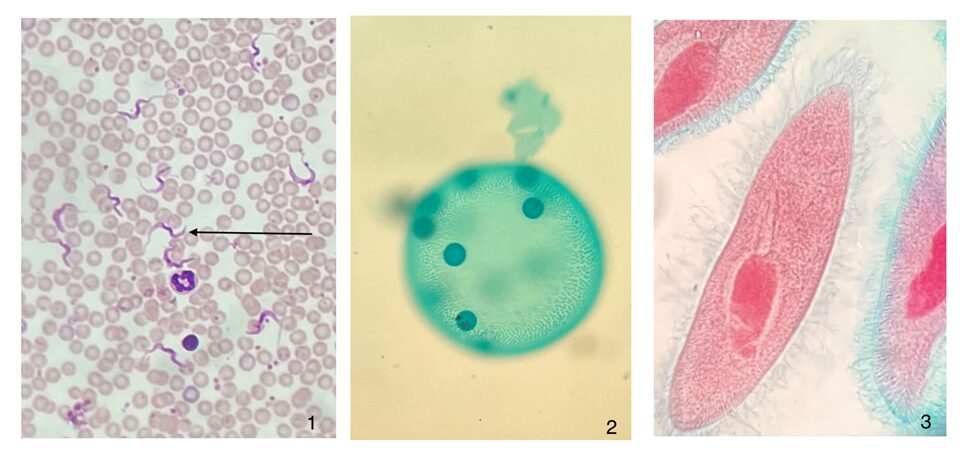
Figure 3: Photographs of microscope slides of flagellated and ciliated protists.1. Trypanosomes, a flagellated parasite, in human blood. 2. The somatic cells in a Volvox colony are flagellated, and permit locomotion of the colony. 3. Paramecium’s cell surface is covered with cilia, which is structurally equivalent to flagella (Courtesy of Andrew D’Argenio, 2022).
A flagellum is made up of a structure known as an axoneme (Gilpin et al., 2020). At the center of the axoneme is a pair of microtubules radially surrounded by nine other microtubule pairs, or doublets, which make up the “axonemal sheath” (Gilpin et al., 2020). These microtubules consist of “polymerized tubulin protein monomers” (Goldstein, 2015). The conformation of the axoneme is referred to as a “9+2 arrangement” (Gilpin et al., 2020). The nine microtubule doublets along the circumference of the axoneme are attached to each other via nexin links and are attached to the central pair via radial spokes, as can be seen in Figure 4, adapted from Gilpin et al. (2020).
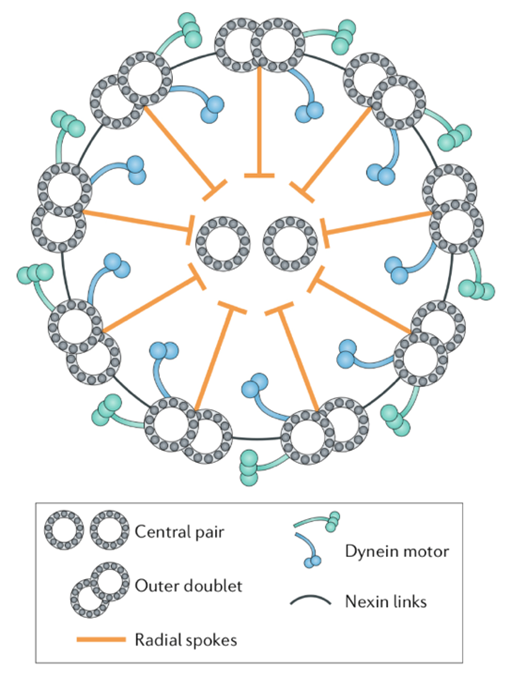
Figure 4: Structure of an axoneme found in flagella. While some variations can be found in the flagella of certain organisms, this figure represents the typical structure of an axoneme, with a central pair of microtubules surrounded by nine microtubule doublets, according to the 9+2 arrangement (Gilpin et al., 2020).
The dynein motor proteins found on each of the outer microtubule doublets are responsible for creating the flagellum’s wave-like motion. The dynein proteins hook onto their neighboring microtubules along the axonemal sheath and use the neighboring microtubules to pull their own microtubules in a direction parallel to their axis. This creates a sliding motion between the microtubules along the circumference of the axoneme, in such a way that resembles the actin-myosin interactions that occur in the contraction of muscles in humans (Brennen & Winet, 1977). While the exact mechanism by which the dynein and microtubule interactions translate to the overall beating of the flagellum is not known with certainty, experimental data suggests that the control of the flagellum’s cycle of oscillation is “distributed across a variety of asymmetric mechanical and chemical regulatory complexes” (Gilpin et al., 2020). One proposed mechanism for flagellar beating involves dynein molecules traveling in one direction along a microtubule, which “gradually increases elastic stress in the system; this stress eventually stalls the [dynein] motors and leads to reversal in their walking direction” (Gilpin et al., 2020). The dynein molecules are capable of walking along adjacent microtubules, until the tension between the two microtubules, presumably in the nexin links, reaches its limit and pulls the dynein molecules backward, making them walk in the opposite direction. An easier way to visualize this phenomenon would be to imagine a dog on a leash. As the dog walks away from its owner, the tension on the leash begins to increase, but the dog can still walk without resistance from the leash until the leash has reached its maximum length. At this point, tension in the leash is at its greatest, and the dog’s movement away from its owner is halted and the dog experiences a tug in the opposite direction of its motion. Essentially, the dynein motors repeatedly walk back and forth along the microtubule, reversing direction when they are pulled back by the elastic force between the microtubules.
Another important feature of the flagellar structure is their basal bodies. While research on the flagella and motility of Gonium itself may be limited, research on Chlamydomonas can provide insight on the motility of Gonium, seeing as Gonium is a colonial organism belonging to the Volvocaceae family, which are made up of “biflagellate Chlamydomonas-like cells” (Nakada & Nozaki, 2015). Therefore, the unicellular Chlamydomonas can be used to model the motility of the individual cells that make up the Gonium colony, with the colonies typically consisting of 16 cells. Chlamydomonas have two flagella, with the one closer to the cell’s eyespot being the cis flagellum, and the one further from the eyespot being the trans flagellum (Wan, 2018). The two flagella are interconnected through their basal bodies as can be seen in Figure 5, adapted from Wan (2018).
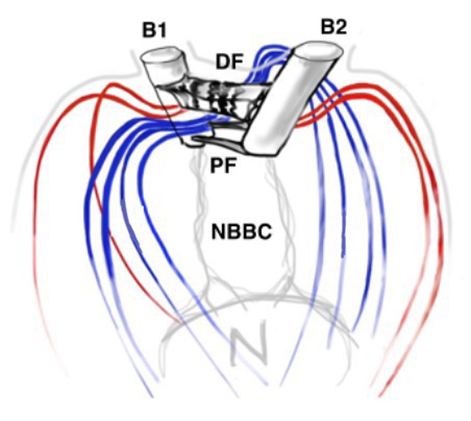
Figure 5: Basal Bodies of Chlamydomonas Flagella B1 and B2 represent the basal bodies of the Chlamydomonas’ flagella, interconnected via “fibrous/contractile connections” represented by DF, PF, and NBBC. N represents the nucleus (Wan, 2018).
The basal bodies are noteworthy because they assist the biflagellate cell in achieving a synchronized beating movement. This is referred to as “intracellular coupling” (Wan, 2018). When Chlamydomonas with only one flagellum were studied, “isolated cis flagella were found to beat with lower mean frequency as well as lower frequency noise” (Wan, 2018) than their trans counterparts. However, this difference in frequencies is not observed in wildtype Chlamydomonas, whose flagella beat in synchrony to produce a breaststroke-like movement (Figure 6). It is clear that the synchronization of a single Gonium cell’s flagella through their interconnected basal bodies is a necessary design feature of biflagellate cells that ensures effective flagellar motility and prevents the flagella from having different beating frequencies that would hinder motility in a particular direction, such as towards light, in the case of phototactic organisms like Gonium.
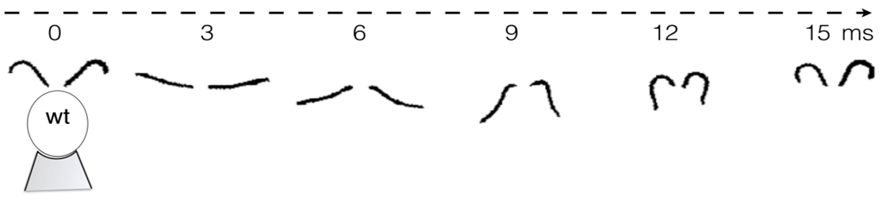
Figure 6: Movement of the Flagella of a Wildtype Chlamydomonas Wildtype C. reinhardtii was held in place with a micropipette (as seen at 0 ms) and the movement of its flagella was mapped over the course of 15ms, revealing its breaststroke beating pattern (Wan, 2018).
While Chlamydomonas are unicellular organisms whose flagellar interactions can be described by intracellular coupling, Gonium is a multicellular organism whose flagellar interactions are more complex. Chlamydomonas can be used to model the synchronization of each individual cell’s flagellar synchronization in a Gonium colony, but a different model needs to be used to understand how the flagella of the whole colony produce synchronized motion.
Experimental data suggests that spermatozoa swimming near each other have a tendency to synchronize their flagellar waves and end up beating in phase (Goldstein, 2015). Other experiments show that the phases of the flagella of somatic Volvox cells also couple, and that this coupling is dependent on how close the flagella are to each other (Gilpin et al., 2020). This is referred to as Huygens synchronization. The concept, named after Christiaan Huygens, involves the synchronization of two nearby objects. Huygens noticed this phenomenon in nearby clocks whose pendula were initially out of phase. After some time, the pendula of the clocks were in-phase and had become synchronized. This occurred because the clocks were hung on the same wall, with the vibrations of their shared structure being responsible for their synchronization. This same principle can be seen in the synchronization of Gonium’s flagella, that share a common fluid medium, through which the flagella are capable of coupling. This allows the multicellular organism to move in an effective and synchronized manner, without the interference of opposing flagellar beats.
Gonium, like all other microorganisms of that size, live in a low-Reynolds-number environment (Purcell, 1977). The Reynolds number is used to characterize fluid environments. It is a ratio that compares inertial forces to viscous forces in a particular fluid. The formula for calculating Reynolds number is written in Equation 1, in which Re, the Reynolds number, a unitless quantity (Gilpin et al., 2020), is equal to the velocity v multiplied by the “dimension a” (Purcell, 1977), which is more commonly denoted by length l, divided by the kinematic viscosity v of the fluid.
For microscopic organisms like Gonium living in an aqueous environment and having such a small size and velocity, the ratio of inertial forces to viscous forces is very small, to the point where “inertia is totally irrelevant” (Purcell, 1977). The effects of the viscous forces significantly outweigh inertial forces. According to Purcell, the Reynolds number for organisms as small as Gonium can be on the order of 10-4 or even 10-5. Therefore, it can be said that organisms like Gonium, i.e. “self-propelling bodies in the viscous (so-called low Reynolds number) regime experience no net forces or torques, so that motion is completely specified by their shape kinematics” (Wan, 2018). For Gonium to move, it must use its own motile mechanisms, the beating of its flagella, to push itself through the aqueous fluid it inhabits, since external forces would only make an organism of Gonium’s size continue to travel mere angstroms if discontinued (Purcell, 1977).
Since the Reynolds number is well below 1 for Gonium in water, Gonium’s locomotion would be considered laminar flow, which is characterized by the object moving through the fluid displacing said fluid in a manner that causes little mixing of the fluid layers displaced in addition to causing the fluid particles to move in regular trajectories (Lienhard & Lienhard, 2008). Moreover, the viscosity of the fluid is important to consider with this type of flow. Laminar flow can be described using Stokes’ Law (Wray, 1977). Stokes’ Law describes the movement of objects through viscous media, as well as the drag forces involved in slowing down the objects. Since Chlamydomonas are considered “pullers” (Goldstein, 2015), Gonium is likely considered a puller as well, that is its flagella are in front of the cell and lead the cell in the direction of motion. Figure 7, adapted from Goldstein (2015), represents the velocity lines of a Chlamydomonas cell traveling towards the left. The two green arrows represent the forward thrust produced by the breaststroke movement of the two flagella, while the purple arrow represents the drag force produced by interactions with the viscous fluid. Together, the 16 cells that make up gonium produce these same movements to collectively allow the colony to be motile. As it turns out, flagella are effective structures for moving in a high-Reynolds-number fluid environment because of their flexibility (Purcell, 1977). E. M. Purcell describes movement in such an environment for microscopic organisms as being analogous to a human in a “swimming pool that is full of molasses” in which it would be impossible to move and body part “faster than 1cm/min” (Purcell, 1977). In such a case, the only possible way to move is through the wiggling and deformation of one’s own body. However, if the deformation is a form of reciprocal motion, or a back-and-forth motion repeating the same deformation, the organism will not be able to travel anywhere, and will only travel in the same cyclical path, since inertial forces do not apply at such a low Reynolds number. Purcell explains The Scallop Theorem, which is that a scallop, having only one hinge, or “degree of freedom” (Purcell, 1977) to move, would be unable to travel in such viscous environments because of this reciprocal motion. Gonium overcomes this problem by having two flexible appendages, its flagella, which are designed to have a high degree of freedom in their movement, as can be seen in Figure 8. This allows Gonium to effectively move through the fluid environment it inhabits, which is crucial for phototaxis.
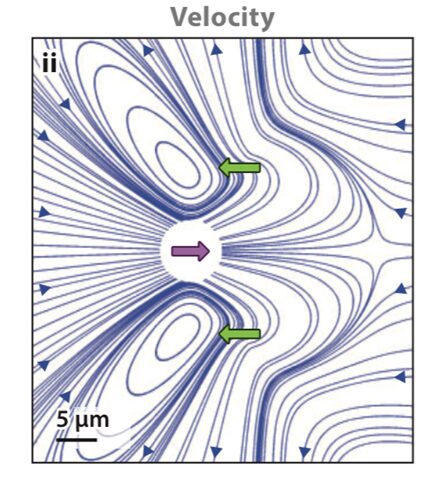
Figure 7: Flow Lines and Velocity of Chlamydomonas. “Color scheme indicates flow speed magnitudes” (Goldstein, 2015). The green arrows represent thrust produced by the flagella, while the purple arrow represents resistive drag force (Goldstein, 2015).
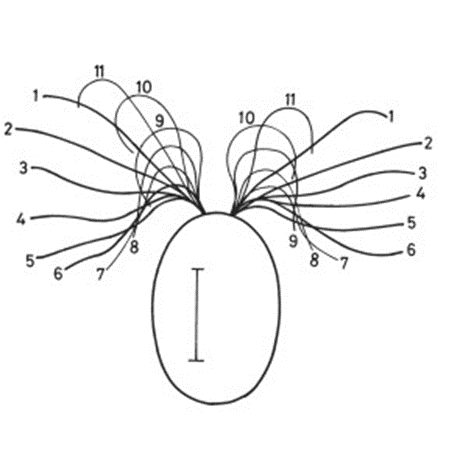
Figure 8: Stages of Flagellar Strokes. This diagram shows that the breaststroke-like swimming pattern Gonium and Chlamydomonas occurs over several steps through the bending of the flagella. Figure adapted from The Chlamydomonas Sourcebook (Second Edition) (2009).
Eyespot Optics and Properties
The eyespot, or stigma, of Gonium is an evolved structure focusing on providing a path to Gonium survival. For a photosynthetic organism, light is a lifeline, so Gonium must always stay with light. The orientation and movement it performs to stay in brilliant beams requires a reliable optical sensor, the Gonium eyespot. It consists of an exterior membrane, 3-8 rows of eyespot globules, and photoreceptors within the inner lining of the eyespot that make up the sensing ability of the stigma (Arakaki et al., 2013; Foster and Smyth, 1980; Lang, 1963). A single eyespot is about 70 micrometers in diameter and sensitive to light within the entire granule but most sensitive in the central section (Lang, 1963; Mast, 1916). This is where the bulk of the photoreceptors are located.
To focus light on this central area, we might hurriedly think that the exterior structure does all the focusing as a convex lens; however, no major focusing effect is seen in the outer membrane, although it does function as a small mirror, which we will touch on later (Böhm and Kreimer, 2021; Foster and Smyth, 1980). In contrast to the green-pigmented regions of the rest of the Gonium cells, the outer membrane of the eyespot is hyaline, taking on a yellow-orange appearance from the interior eyespot complex, as can be seen in Figure 9.
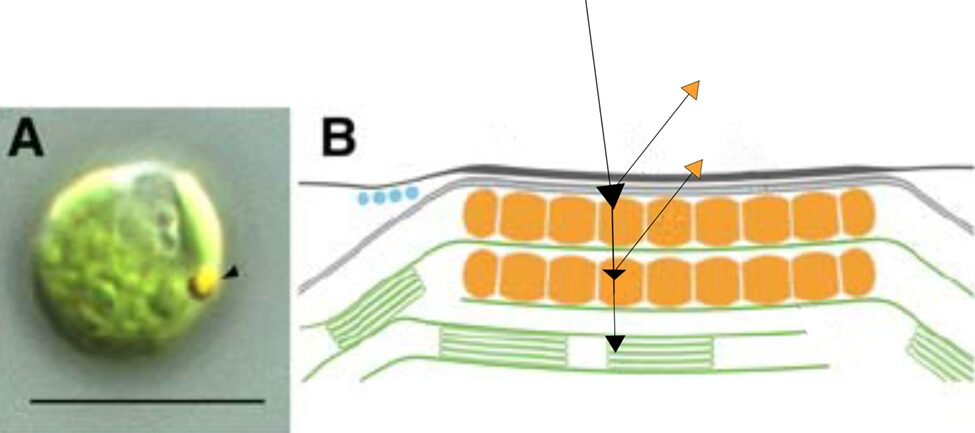
Figure 9: Light Micrograph and Reflectivity Diagram of Gonium Eyespot. Black arrows show a rudimentary path of incident light, some wavelengths of which are focused to photoreceptors, where other wavelengths are reflected, mostly in the yellow-orange visible spectrum (Schmidt et al., 2006).
This membrane does have a lower refractive index than the surrounding, green-pigmented areas, and takes in more light (Foster and Smyth, 1980). The magic happens once incident light shines through this membrane.
The rows of eyespot globules that can be seen in Figure 10 show where light is first received after entering the membrane. The layers of these eyespots are between 1 and 10 wavelengths (Foster and Smyth, 1980). These eyespot globules do not contain light processing capacity, instead, they act as a light directional field, using the reflection of light and interference to guide the incident light to the photoreceptors at the posterior of the organelle.
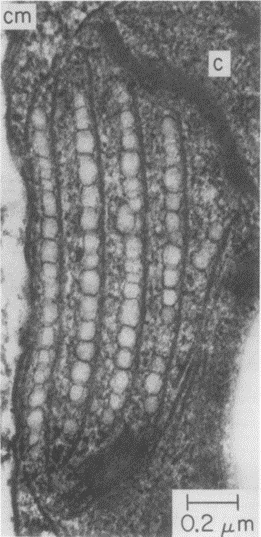
Figure 10: Rows of Eyespot Globules imaged using an electron micrograph. Four clear rows of eyespot globules can be seen in the figure, surrounded by membranes (Foster and Smyth, 1980).
They act as natural quarter wavelength reflectors—a structure with multiple alternating layers of reflective material that are approximately ¼ the thickness of the target wavelength (Foster and Smyth, 1980; Paschotta, 2023). They are a form of dielectric mirrors and have an important purpose: to amplify the light that is advantageous to Gonium and absorb light that is not. The reflector is made up of alternating layers with a contrast of reflective index between both. Light passing through these multiple layers exhibits Fresnel behavior and continues to be reflected within the eyespot, as can be seen in Figure 11 (Bragg Reflector, 2022). Gonium stacks contain 4 layers of eyespot globules, two pigmented and two unpigmented layers, which we can visualize as having one less stack than the quarter-wave reflector in Figure 11. If light enters from wide angles of incidence, it is unlikely to go through the layers of refraction and is instead absorbed or reflected (Paschotta, 2023; Foster and Smyth, 1980). However, light from close-to-ideal angles of incidence experiences significant constructive interference, increasing amplitude and coming out stronger. For Gonium, this structure is key to effectively activating photoreceptors in a broad range, but primarily in the central deposit of photoreceptors. Light that is transmitted through the quarter-wave stack of eyespot globules will be channeled towards the central deposit of proteins, ensuring a high level of effective activation, but even the light from non-ideal angles plays an important role as it is reflected across a broad range, leading to an activation of photoreceptors across the entire eyespot. This is a biological design solution for two problems—Gonium’s need for concentrated light in areas with concentrated photoreceptors and Gonium’s need for a wide range of photoreceptor activation, which is important for positioning itself to light sources.
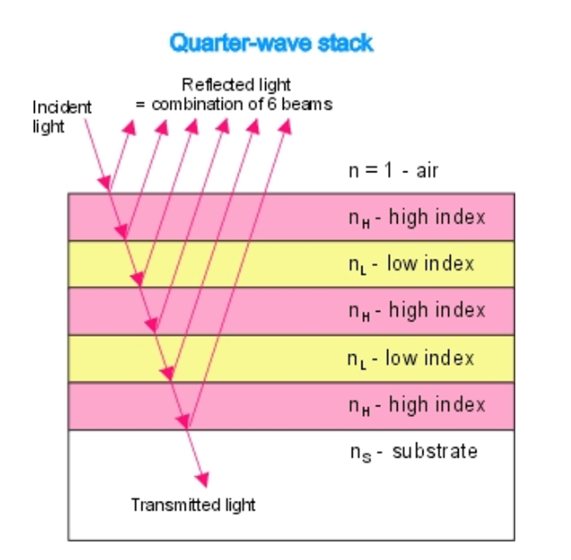
Figure 11: An example quarter-wave reflector. The quarter-wave stack model can be compared to the eyespot rows in Gonium pectorale to visualize the mimicry present (Bragg Reflector, 2022).
The layers of eyespot globules alternate between pigmented and unpigmented layers, with refractive indexes of 1.5 and 1.35, respectively. With three or more layers of eyespot globules, Foster and Smyth found that stacks were over 85% effective quarter wavelength reflectors. In addition to amplifying light, the interference caused by the stacks has a smaller destructive interference effect, which creates an interference curtain, spreading light across a larger area. This does not significantly diminish the more intense target light of the system, however.
If we zoom out a bit further, we can group the outer membrane and the layers of eyespot globules to see that Gonium’s eyespot forms another significant optics device—a Cassegrain reflector, otherwise known as a Cassegrain telescope. Gonium effectively mimics this structure, which can be seen in Figure 12. An original theory by Mast in the early 20th century posited that the outer hyaline membrane of green algal eyespots acted as a converging, convex lens, but Mast was partially disproven in the 1980s by Foster and Smyth, who posited the now-accepted theory that while the outer membrane might have some characteristics of a convex lens, what is more relevant is the characteristics it exhibits of a concave mirror within the reflectivity scheme of the eyespot.
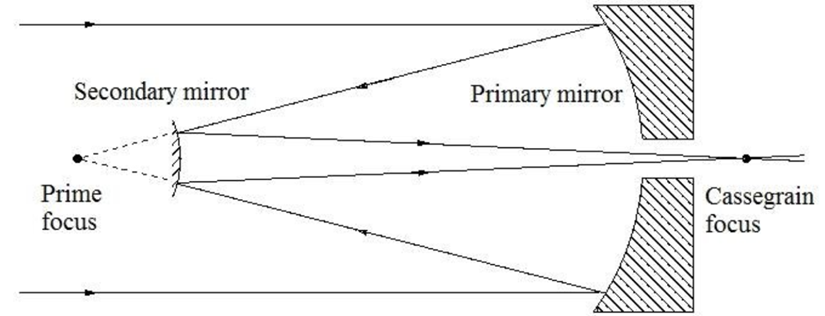
Figure 12: A Cassegrain reflector structure, which uses two mirrors to create a central focus for incident light. This structure is mimicked organically in Gonium (Fimin, 2020).
In short, once incident light passes through the outer membrane, it is partially reflected by the layers of eyespot globules with high reflectivity. Light will often be reflected towards the outer membrane, which from the interior, acts as a concave mirror. Reflected light will be focused on the beam path of the light in this case, towards the central cluster of photoreceptors. This mimics the structure of a Cassegrain telescope, allowing Gonium to make the most use of the light that it receives by ensuring incident light is received in the larger groups of eyespot globules (Britannica, 2016; Yoshimura and Kamiya, 2001). This is one part of Gonium’s physical design solution to the challenge it faces of gathering light for photosynthesis. It also contributes to the final optics structure we can see Gonium mimic––the directional antenna.
The combination of the natural quarter wavelength and Cassegrain reflector systems creates one more almost supernatural phenomenon, a directional antenna. In our lives, directional antennas are often seen as satellite dishes, which pick up light in a tight range of incidence angles but are supported by reflective systems like Cassegrain telescopes. The varied reflective index of the outer membrane allows for incident light primarily at angles closer to perpendicular to the surface of the eyespot (Böhm and Kreimer, 2021; Mast, 1932). The light that incidents at wide angles is often absorbed and standardized by the quarter wavelength reflectors, and therefore most of this light is not reflected (Foster and Smyth, 1980). Incident light from angles closer to perpendicular is much more likely to be reflected in higher proportions from the quarter wavelength reflector and then enter the Cassegrain system. This system converges incident light on a beam path behind the concave mirror, meaning that not only is light funneled to the larger group of pigment and photoreceptors, but the light is reflected in high intensity outside of the membrane as well, creating a true directional antenna. (Britannica, 2016; Foster and Smyth, 1980). All three of these natural optic systems optimize the light absorbed by Gonium, which is incredibly important in a photosynthetic system like Gonium which is heavily dependent on orientation to light for survival (De Maleprade et al., 2020).
Now, the result of this intense reflective system is exceptional light convergence to a wide range of photoreceptors. The reflected light and the incident light generated from the quarter wavelength reflector cause interference, which functionally guides additional light toward the various photoreceptors at the back of the cell. Photoreceptors receive light from the incident source and interference in the area. The interference patterns ensure that even though incident light is limited in angle, light reaches all different groups of photoreceptors at the posterior of the eyespot, which is important for accurate triggers of phototaxis. The interference curtain created by quarter wavelength receptors is essential in ensuring this and functions as a biological design solution to the conundrum of providing proper light for activation at a wide range of photoreceptors. (Arakaki et al., 2013; Böhm and Kreimer, 2021; Foster and Smyth, 1980). Once the light sources are properly focused on photoreceptor proteins, the cell can use light signals for various purposes.
The directional antenna system present in the eyespot is an incredibly valuable biological design solution, which can be seen easily when compared to a mutant type of Gonium that contains an eyespot with a noticeable absence of globule proteins (Böhm & Kreimer, 2021). This means light detection can take place independently of its incident angle. There is no directional antenna, and the result is the improper orientation of light and ineffective photoreceptor activation, summarized in a phenomenon called “negative phototaxis,” which will be further discussed in the phototaxis section of this article (Leptos et al., 2023).
Without getting too in-depth into chemical processes in the photoreceptors, it is important to understand the absorbance spectra and functions of the photoreceptor proteins. The proteins within the photoreceptors are primarily Channel-Rhodopsins, Phototropins, and Cryptochromes. Channel-Rhodopsins absorb light at a peak absorbance wavelength of 480 and wider red spectra. Cryptochromes provide a blue absorbance spectrum, as well as some red absorbance. Phototropins absorb light in blue spectra and primarily control the adjustment of the aperture of the eyespot (Böhm and Kreimer, 2021; Hubbard and Kropf, 1958). Because there are no spectra of absorbance in the yellow and lighter orange range, these wavelengths of light continue to reflect and create the soft, yellow-orange color that we see in the appearance of Gonium.
Once the correct wavelengths of light are absorbed by photoreceptors, they can be processed and provoke a chemical response that further leads to flagellar movement and, by extension, Gonium’s phototaxis. The eyespot is therefore an important structure that takes care of the light input required for the function of Gonium.
BEHAVIORAL SYSTEMS REQUIRED FOR MOTILITY OF GONIUM
While Gonium’s structure is particularly well-adapted to its need to perform phototaxis, the colony’s behavior is equally proficient in taking advantage of its structural properties for effective and accurate reorientation towards sources of light. It is observed within typical species of Gonium that colonies displace themselves to end up in a relatively consistent position, namely with their anterior face perpendicular to sources of intense light (De Maleprade et al., 2020). The following sections of this essay will address various aspects of Gonium’ behavior.
Helical Propulsion
To understand the movement of Gonium, it is necessary to adopt a particular interpretation of the Reynolds number. This interpretation, proposed by Langa and Powers (2009), suggests that at low Reynolds numbers, the Reynolds number can be viewed as a “non-dimensional coasting distance” when studying microorganisms like Gonium, for which this quantity is very small, as established previously. As the colony is considered to be in linear uniform motion while it is coasting, the external forces and torques to which it is subject compensate each other in accordance with Newton’s first law of motion. In this case, the directional forces being exerted on the colony are viscous drag and the forces exerted by the fluid in reaction to the forces exerted by Gonium’s flagella. The external torque forces exerted on the colony are represented by the vectorial sum of torque forces exerted by the fluid in response to flagellar activation, and torque forces due to drag.
As established earlier, given Gonium’s low Reynolds number, the fluid flow around the colony can be considered laminar and therefore it can be described using the Stokes equation. From this equation, an expression of the external viscous drag and torque forces as a function of the viscosity of water and the approximate radius of the colony were deduced by De Maleprade et al. (2020). These expressions were further used to deduce those of the forces and torques exerted by Gonium’s fluid environment in reaction to the action of the flagella.
Given in a frame fixed to the body of the colony, these expressions determine the direction of the colony’s movement while it coasts. The orientation of the directional force exerted by the fluid surrounding the colony indicates asymmetry in the forces exerted by the colony’s flagella. This force asymmetry in the body-fixed frame causes a remarkable helical movement, crucial to Gonium’s capacity to reorient and displace itself.
As seen in Figure 13, while the movement of the center of the colony is characterized by a helical trajectory, the flat surface of the disk formed by the colony remains perpendicular to the direction of movement (Mast, 1916; De Maleprade et al., 2020). Through this helical movement, Gonium can swim toward sources of light, but also describe wide arcs and turn and face sources of light with its anterior side. Gonium is therefore capable of both forward movement and rotation, which combine to allow omnidirectional motility.
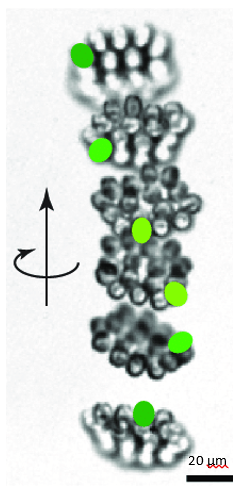
Figure 13: Helical upward swimming movement of a colony of Gonium. Successive images are separated by 0.4s. The green spots highlight successive positions of one specific cell (De Maleprade et al., 2020).
The distinctive behavior of Gonium’s flagella at the source of this force asymmetry is another notable design solution naturally incorporated into the organism. Indeed, through this asymmetry, lack of perfect flagellar coordination between cells becomes an asset for the colony as a whole.
Phototaxis
Gonium’s orientation mechanism is driven by phototaxis. The multicellular algae will move through the water in a direct path with its flat surface perpendicular to its trajectory. During its movement, it will rotate about itself in a helical motion as previously described (Mast, 1916). As this organism is photosynthetic, it requires the ability to orient itself to find light. This is where phototaxis becomes necessary for Gonium’s survival (De Maleprade et al., 2020). This biological process is an organism’s ability to steer itself toward light without the use of a system for optical image perception. In fact, all phototactic organisms share three traits: a helical swimming path of the cell(s) with axial rotation, photoreceptors that influence the beating of the flagella, and refractive or pigmented structures placed near the photoreceptors that cause its excitation to be dependent on the organism’s orientation with respect to the light (Böhm & Kreimer, 2021). This is how Gonium and other phototactic organisms have adapted to accommodate their photosynthetic needs. Phototrophs survive on photosynthesis and to meet this intrinsic need, the evolutionary solution was this method of direct orientation towards light.
In the case of Gonium, phototaxis depends on both the sensing of the light by the eyespot (the photosensor), and variations in flagella mobility which incite movement towards the light (Leptos et al., 2023). The eyespot’s ability to focus light depends heavily on the direction of the incident rays. Considering every one of the cells in the colony are in a singular plane, where the photosensors are each aligned branching out from the center of the plane in a similar direction, each of their focal points are also very similar. Unlike the focal points for lenses that are fixed, those for the Gonium cells vary with the angle of the incident light. Additionally, the amount of light absorbed by the eyespot is dependent on these fluctuating focal points indicating the photosensor possesses directional sensitivity (Leptos et al., 2023; Mast & Johnson, 1932). That is to say, it has the ability to detect the direction of light originating from the anterior side of the eyespot only. Furthermore, when light is detected, an adaptive response is triggered, causing a nonuniform axial force to be produced from the outer flagella of the cell, where the force is greatest for cells facing away from the light (Leptos et al., 2023). The force exerted by the flagella is dependent on the intensity of light captured by the photosensors. (De Maleprade et al., 2020). A result of the eyespot’s sensitivity, depending on its incident direction, is that the maximum photoresponse to the light occurs when the eyespot is perpendicular to the light, and light intensity is at its highest. A net torque about an axis within the same plane as the colony is generated as a result of this nonuniform force and light directionality, which when balanced by rotational drag, causes the cell to turn toward the light (Leptos et al., 2023). This reorientation is only possible due to a colony’s adaptive response to changes in light intensity. The adaptive phototactic response is actuated by the outer flagella within a period of time that is comparable to Gonium’s rotational period about itself. This allows the colony to be able to scan their environment with enough time to alter their trajectory towards the light if needed (De Maleprade et al., 2020). This design seems to explain how Gonium colonies are able to correctly orient themselves toward the light given they are in constant rotational motion. If the perception of light stimulation occurs at a rate significantly smaller or larger than the rotational period, no phototactic reorientation will take place. The asymmetrical flagellar forces (the flagella furthest from the light generating the greatest force) will not persist for a substantial amount of time, thus their change in orientation will likely not result in the colony being perpendicular to the light, preventing them from having maximal photosynthetic efficiency (De Maleprade et al., 2020).
Gonium’s direct orientation mechanism can be more easily understood by observing the change in the organism’s trajectory in response to the changing direction of light. If light is shined at a non-direct angle on the frontal face of a previously oriented Gonium, the organism will immediately turn to ensure that it is relatively perpendicular to the light (Mast, 1916). Moreover, whenever a change in the direction of light is noted, the Gonium cells will experience a noticeable slowdown where its swimming speed is halved for several seconds (De Maleprade et al., 2020). This can be seen in Figure 14, where there are noticeable dips in velocity when the light changes direction. Additionally, phototactic reorientation, visualized in Figure 15, contains a waviness character. This waviness is observed in all colonies. This allows a swifter change in direction compared to a smoother trajectory. That is to say, while this character is disadvantageous to swimming efficiency, it may favor more efficient phototaxis. The waviness of their path allows a better perception of their environment leading to a better detection of changes in light (De Maleprade et al., 2020). Thus, this slowdown and waviness character while working with the phototaxis response time provide the perfect solution to efficiently detecting the direction of light so that the colony can always orient itself directly.
Interestingly, if the intensity of the incident light decreases while maintaining its direction after the organism has already oriented itself, Gonium will increase its rate of movement for a short period of time. There is no response for the opposite scenario, where the intensity is increased (Mast, 1916). This may be explained by the relationship between flagella activity and light intensity: the flagella will cause a greater torque when exposed to a lesser intensity of light (Leptos et al., 2023). Thus, a decrease in intensity may correlate to what seems to be a change in the direction of light, causing increased activity to find the new direction of the light. However, this reaction is contingent on the rate at which the intensity of the light is changed. That is to say, if the intensity were to be gradually diminished over time, then there would not be any induced movement (Mast, 1916).
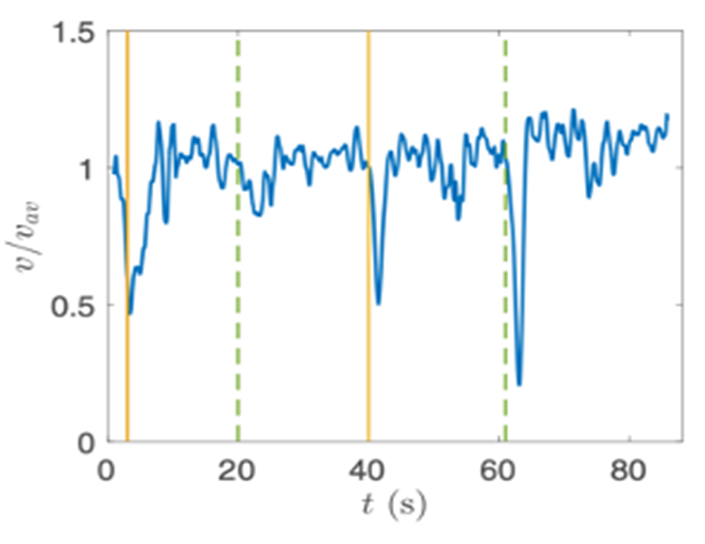
Figure 14: Velocity of a Gonium colony. Graph normalized as a function of time with alternating directions of light. The green line is from the left and the yellow from the right ( De Maleprade et al., 2020).
.
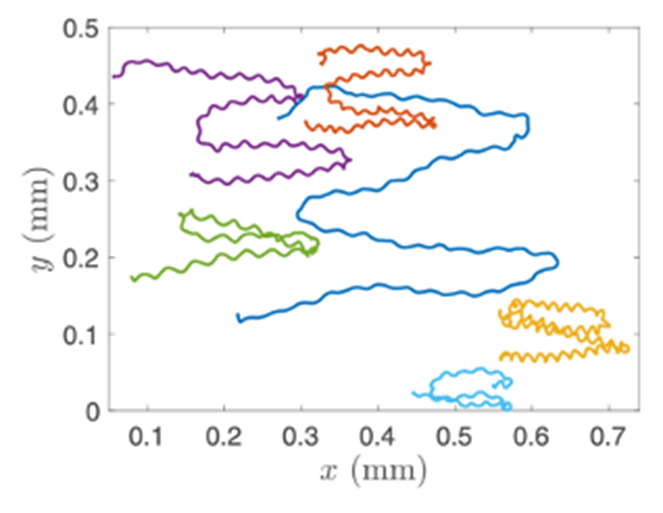
Figure 15: Trajectory of Gonium Colonies. The trajectories of multiple colonies as blue light is shone alternatively from the left and right sides ( De Maleprade et al., 2020).
The helical propulsion of a colony can be related to the cells’ phototactic tendencies. The light perceived by each eyespot depends on their respective cell’s angle relative to the incident light (De Maleprade et al., 2020). It is known that the ideal angle between the light and the eyespot is 90°, however, since the photoreceptors are placed outward, radially from the center of the colony, not all cells will be in their perfect positions at a given time (Leptos et al., 2023; Mast & Johnson, 1932). And so, when light hits the colony obliquely, the cells furthest from the light have eyespots that are shaded from the light while those closest are fully exposed (Mast, 1916). It is the flagella of these shaded cells that produce the most force to capture more energy from the light (Leptos et al., 2023). The colony will then turn so that the cells that were once in the light are now shaded, and those that were shaded are now in the light, as can be seen in Figure 16. This change in position is continuous since as the colony spins the surface of each zooid is exposed to light of a variable intensity (Mast, 1916). As each individual cell vies for their perfect position as they move towards the light, the wavy helical path is observed.
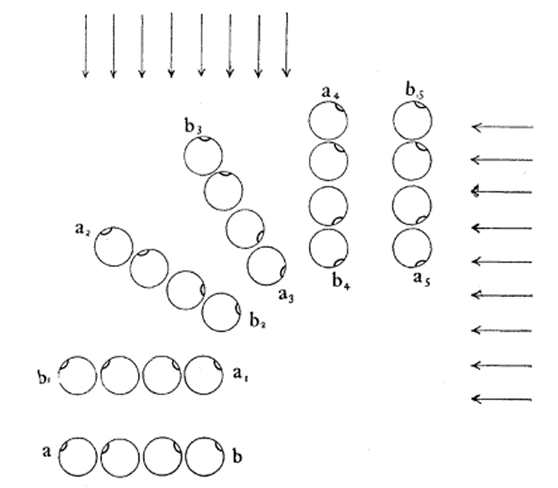
Figure 16: Light-prompted orientation of a section of a Gonium colony. Gonium cells reposition for ideal light absorption (Mast, 1916).
In the preceding parts of this section, positive phototaxis was described, where the anterior face of the cell moves toward the light. Organisms that produce this response to light are considered the wild type. However, there is a mutant type of Gonium that experiences negative phototaxis. Negative phototaxis is the result of the organism’s cell body functioning as a convex lens attributed with a refractive index greater than the surrounding water. With this “lens,” the intensity of light being absorbed on the posterior side of the photosensor is superior to that of the anterior face of the eyespot (Leptos et al., 2023). This phenomenon is prevented in wild types due to the globular layer of the carotenoid vesicles, allowing positive phototaxis (Böhm & Kreimer, 2021). As a phototactic organism will orient itself such that it will absorb the greatest intensity of light, mutant types will turn so that it is facing away from the light source (Bennett & Golestanian, 2015). Consequently, this error in orientation causes the organism to move away from the light, as the greatest detected intensity is meant to be in the direction of the light (Leptos et al., 2023).
CONCLUSION
Gonium pectorale exhibits a combination of seemingly simple but notably complex physical structures that allow it to have reliable motility through phototaxis and helical propulsion. When we observe the motility package of Gonium pectorale as a whole, we see a system of systems tried and tested from millennia of evolutionary pressures making it a truly effective organism. We can see how its systems experience divergent evolution as compared to other algae of the Chlamydomonadales Family. More differentiated colonies like Volvox have larger movement considerations, and less differentiated single-celled Chlamydomonas lack greater movement behaviors.
Gonium itself also exhibits a particular toolset of biological design solutions that differentiate it from other algae in its evolutionary lineage. Gonium is a photoautotroph, which brings a certain set of conundrums. Gonium must absorb light in the most effective way for photosynthesis and position or move itself in an efficient way. To move in an effective way, Gonium faces problems like having to function in low Reynolds number environments and prevent interference between flagella. Gonium solves this problem physically by the flexible shape of the two opposite flagella generating effective propulsion in low Reynolds number environments and with the basal body connection of the flagella inherently synchronizing cis and trans flagella within a cell. It also solves this problem behaviorally with metronome-like synchronization between the sixteen cells and its unique helical propulsion pattern that allows for omnidirectional simple movement. To collect light in an effective way, Gonium must achieve a wide range of activation while still guiding light to the center of the eyespot. Gonium manages this by its unique directional antenna’s focusing ability and interference effects. However, Gonium still faces the challenge of orienting to these light sources while under rotational motion. By using an adaptive response phototaxis for fine-tuned orientation to light and waviness character of trajectory for what is essentially light scanning, Gonium is able to orient incredibly efficiently and take full advantage of incident light sources. The biological design solutions Gonium has adapted make it a resilient organism that is well suited to its environment. These solutions and their respective conundrums can be better visualized in Figure 17.
On a visible scale, we can compare Gonium to jellyfish such as ctenophores, also known as comb jellies. Comb jellies exhibit similar propulsion abilities using cilia rather than flagella, and also do not have a central nervous system, although cell interaction is exhibited. The physical principles that evolved in Gonium that we have investigated do compare to the physical properties of organisms in much bigger and smaller magnitudes, so discovering the mechanisms of Gonium may give us some valuable insight and a point of comparison in the discussion of organisms like comb jellies (Smithsonian Ocean, 2023).
Beyond comparisons to other organisms, understanding the functions of photosynthetic microalgae and their “important role as primary producers and thus also for the global carbon cycle” gives us a reason to learn more about Gonium (Field et al. 1998, qtd. in Böhm & Kreimer, 2021). With carbon being an essential topic in global warming, Böhm & Kreimer suggest that a “better understanding of the processes [of] how the different inputs are integrated into an optimal orientation in the light is highly desirable.” Without photosynthetic algae, world ecosystems would not function, and understanding the connection shows us the value of learning more about these organisms. In a world where we can never know everything, it is a noble pursuit to broaden our understanding, even if it is one microorganism at a time.
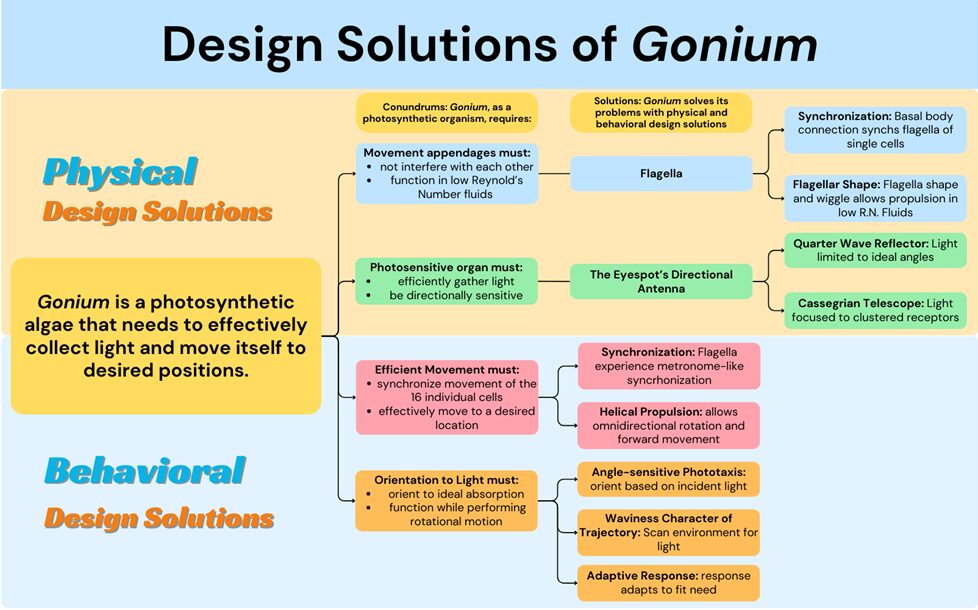
Figure 17: A flow chart visually organizing the conundrums and biological design solutions of the organism Gonium, created by our team.
REFERENCES
Arakaki, Y., Kawai-Toyooka, H., Hamamura, Y., Higashiyama, T., Noga, A., Hirono, M., Olson, B. J. S. C., & Nozaki, H. (2013). The Simplest Integrated Multicellular Organism Unveiled. PLoS ONE, 8(12), e81641. https://doi.org/10.1371/journal.pone.0081641
Bennett, R. R., & Golestanian, R. (2015). A steering mechanism for phototaxis in Chlamydomonas. Journal of The Royal Society Interface, 12(104), 20141164.
Böhm, M., & Kreimer, G. (2021). Orient in the World with a Single Eye: The Green Algal Eyespot and Phototaxis. In F. M. Cánovas, U. Lüttge, M.-C. Risueño, & H. Pretzsch (Eds.), Progress in Botany Vol. 82 (pp. 259-304). Springer International Publishing. https://doi.org/10.1007/124_2020_38
Bragg reflector. (2022, September 15). https://www.batop.de/information/r_Bragg.html
Brennen, C., & Winet, H. (1977). Fluid mechanics of propulsion by cilia and flagella. Annual Review of Fluid Mechanics, 9(1), 339-398.
Britannica, T. Editors of Encyclopaedia (2016, February 14). Cassegrain reflector. Encyclopedia Britannica. https://www.britannica.com/science/Cassegrain-reflector
The Chlamydomonas Sourcebook (Second Edition), Chapter 4 – Motility and Behaviour, edited by E. H. Harris, D. B. Stern, G. B. Witman. Academic Press 2009.
De Maleprade, H., Moisy, F., Ishikawa, T., & Goldstein, R. E. (2020). Motility and phototaxis of Gonium, the simplest differentiated colonial alga [Article]. Physical Review E, 101(2), Article 022416.https://doi.org/10.1103/PhysRevE.101.022416
Fimin, P. (2020). Methods for Resolving Optical Contradictions in TRIZ: An Overview.
Foster, K. W., & Smyth, R. D. (1980). Light Antennas in phototactic algae. Microbiological reviews, 44(4), 572–630. https://doi.org/10.1128/mr.44.4.572-630.1980
Gilpin, W., Bull, M. S., & Prakash, M. (2020). The multiscale physics of cilia and flagella. Nature Reviews Physics, 2(2), 74-88. https://doi.org/10.1038/s42254-019-0129-0
Goldstein, R. E. (2015). Green Algae as Model Organisms for Biological Fluid Dynamics. Annu Rev Fluid Mech, 47, 343-375. https://doi.org/10.1146/annurev-fluid-010313-141426
Greuel, Brian & Floyd, Gary. (1985). Development of the flagellar apparatus and flagellar orientation in the colonial green alga Gonium pectorale (Volvocales). Journal of Phycology. 21. 358 – 371. 10.1111/j.0022-3646.1985.00358.x.
Hubbard, R., & Kropf, A. (1958). THE ACTION OF LIGHT ON RHODOPSIN. Proceedings of the National Academy of Sciences, 44(2), 130-139. https://doi.org/10.1073/pnas.44.2.130
Lang, N. J. (1963). Electron Microscopy of the Volvocaceae and Astrephomenaceae. American Journal of Botany, 50(3), 280-300. https://doi.org/10.2307/2440022
Leptos, K. C., Chioccioli, M., Furlan, S., Pesci, A. I., & Goldstein, R. E. (2023). Phototaxis of Chlamydomonas arises from a tuned adaptive photoresponse shared with multicellular Volvocine green algae [Article]. Physical Review E, 107(1), Article 014404. https://doi.org/10.1103/PhysRevE.107.014404
Lienhard, J., & Lienhard, J. (2008). DOE fundamentals handbook: thermodynamics, heat transfer, and fluid flow. Washington, DC: US Department of Energy.
Mast, S. O. (1916). The process of orientation in the colonial organism, Gonium pectorale, and a study of the structure and function of the eye-spot. Journal of Experimental Zoology, 20(1), 1-17. https://doi.org/https://doi.org/10.1002/jez.1400200102
Mast, S. O., & Johnson, P. L. (1932). Orientation in light from two sources and its bearing on the function of the eyespot [Article]. Zeitschrift für Vergleichende Physiologie, 16(1), 252-274. https://doi.org/10.1007/BF00338127
Melo, D., Porto, A., Cheverud, J. M., & Marroig, G. (2016). Modularity: genes, development and evolution. Annual review of ecology, evolution, and systematics, 47, 463–486. https://doi.org/10.1146/annurev-ecolsys-121415-032409
Moore, A. R. (1916). The mechanism of orientation in Gonium. Journal of Experimental Zoology, 21(3), 431-432. https://doi.org/10.1002/jez.1400210306
Nakada, T., & Nozaki, H. (2015). Flagellate green algae. In Freshwater Algae of North America (pp. 265-313). Elsevier.
Paschotta, R. (2023, September 6). Bragg mirrors. 2023 RP Photonics AG. https://www.rp-photonics.com/bragg_mirrors.html
Pennak, R. W. (1978). Fresh-water invertebrates of the united states (2d ed.). Wiley.
Proyecto Agua. “Dieciséis Nudos Para Una Alfombra Mágica. Gonium Pectorale, Arroyo de Sogo.” Flickr, Yahoo!, 20 Oct. 2023, www.flickr.com/photos/microagua/27736037969/in/photostream/.
Purcell, E. M. (1977). Life at low Reynolds number. American journal of physics, 45(1), 3-11.
Schmidt, M., Geßner, G., Luff, M., Heiland, I., Wagner, V., Kaminski, M., Geimer, S., Eitzinger, N., Reissenweber, T., Voytsekh, O., Fiedler, M., Mittag, M., & Kreimer, G. (2006). Proteomic Analysis of the Eyespot of Chlamydomonas reinhardtii Provides Novel Insights into Its Components and Tactic Movements. The Plant cell, 18, 1908-1930. https://doi.org/10.1105/tpc.106.041749
Smithsonian Ocean. (2023). Jellyfish and comb jellies. Smithsonian Ocean. https://ocean.si.edu/ocean-life/invertebrates/jellyfish-and-comb-jellies
Swilley, K. L. (2018). Phenotypic analysis of two unicellular Gonium pectorale mutants defective in extracellular matrix assembly. [Thesis]. https://krex.k-state.edu/bitstream/handle/2097/38911/KaseySwilley2018.pdf?sequence=1&isAllowed=y
Yoshimura, K., & Kamiya, R. (2001). The Sensitivity of Chlamydomonas Photoreceptor is Optimized for the Frequency of Cell Body Rotation. Plant and Cell Physiology, 42(6), 665.
Wan, K. Y. (2018). Coordination of eukaryotic cilia and flagella. Essays Biochem, 62(6), 829-838. https://doi.org/10.1042/EBC20180029
Wray, E. (1977). Stokes’ law revisited. Physics Education, 12(5), 300.