Abstract
Dinoflagellates produce neurotoxins that target the voltage-gated channels of muscle and nerve cells. Consumption of these toxins through contaminated seafood is the cause of human illnesses such as paralytic shellfish poisoning (PSP). Some of these toxins are complex molecules and are some of the largest naturally produced carbon chains found in nature. Amongst the various energy sources seen in dinoflagellates, photosynthesis, osmotrophy and phagotrophy – which can be seen in mixotrophic dinoflagellates – stand out as a pivotal mechanism governing their survival and ecosystem dynamics. They emphasize the important role of peridinin in light harvesting and the unique regulatory mechanisms orchestrating metabolic rhythms in these organisms. Dinoflagellates are also remarkable due to their ability to produce bioluminescence on the ocean surface. This phenomenon is triggered by shear stress, initiating a cascade of cellular processes to generate the emission of light. Bioluminescence in dinoflagellates has been hypothesized to have evolved as a defense mechanism against predators. Dinoflagellates also show a tendency to move towards chemical stimuli and light stimuli to obtain nutrients and sunlight.
Introduction
Dinoflagellates are unicellular eukaryotic protists part of the phylum Dinoflagellata. First discovered and documented in 1753 by Henry Baker, dinoflagellates have since been studied by scientists and naturalists for centuries. They form a very diverse group of organisms: each dinoflagellate species has its own unique physiological and behavioral characteristics. Some dinoflagellates photosynthesise, others are heterotrophic and hunt for their food; several produce potent toxins, while the rest have not needed those; some are flamboyantly bioluminescent, others prefer to hide in the shadows. Surviving and passing the genetic information to the next generation is the driving force behind the observed diversity of all organisms, including dinoflagellates. A small behavioral adaptation or a particular physiological modification may improve drastically the success of the species. Therefore, these behaviors, morphologies, and adaptations emerge as solutions to environmental challenges.
To better understand how dinoflagellates operate and fully appreciate the solutions they came up with to improve their success in the face of environmental challenges, one must delve into the chemical processes that occur within their cell and their local environment. This requires us to view the dinoflagellates through a chemical perspective. We will describe the chemistry behind the toxins they produce, the way they acquire energy, their bioluminescence, and chemosensory mechanisms.
Dinotoxins
Some dinoflagellate species are known to produce potent toxins referred to as dinotoxins. Toxins are used by an organism to aid in predation, or to defend against predators. Normally, toxic dinoflagellate species are found in low numbers and produce small enough quantities of toxins that they have no environmental or human health impacts (Wang, 2008). However, when large quantities of dinotoxins are produced by blooming dinoflagellate populations, marine organisms, as well as some terrestrial organisms, are severely affected (Wang, 2008). This is of increasing concern considering that the occurrence and geographical distribution of harmful algal blooms has been increasing over the last few decades (Wang, 2008).
When present in large enough concentrations, dinotoxins accumulate in filter feeders such as shellfish, zooplankton, and herbivorous fish (Wang, 2008). These dangerous compounds are then transferred up the food chain; predators eat contaminated prey and accumulate the toxins in their bodies (Wang, 2008). Dinotoxins, as a result, cause the death of fish and shellfish and have led to the episodic death of marine mammals, birds, and animals depending on marine food webs (Wang, 2008).
Most dinotoxins are neurotoxins that affect the functioning of voltage-gated sodium, potassium, and calcium channels. A description of the toxin saxitoxin, as well its impact on animal health, will be presented in the following section.
Saxitoxins
Interestingly, saxitoxins are produced by two organisms in different kingdoms of life: prokaryotic cyanobacteria in freshwater systems and eukaryotic dinoflagellates in marine water systems (Cusick & Sayler, 2013).
Saxitoxin is produced by the dinoflagellates pyrodinium bahamense, several species of Alexandrium, and gymnodinium catenatum (Cusick & Sayler, 2013). Around 30 different saxitoxin analogues have been identified, some more toxic than others (Gad, 2014). Saxitoxin is an alkaloid: an organic molecule containing nitrogen (Cusick & Sayler, 2013). Its chemical formula is C10H17N7O4 and its structure is shown in Figure 1.
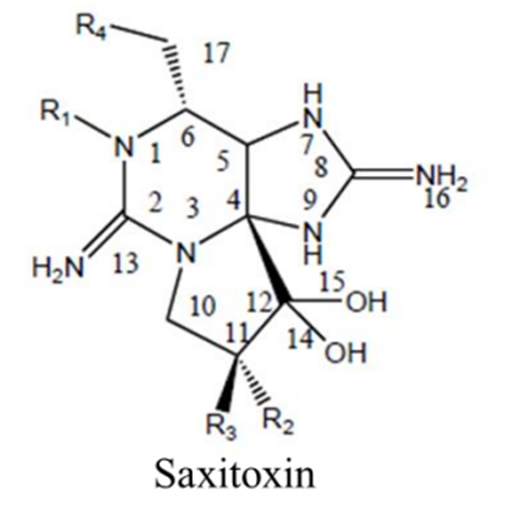
Figure 1: Chemical Structure of Saxitoxin. From (Cusick & Sayler, 2013).
Saxitoxin has the basic structure of a trialkyl tetrahydropurine (Cusick & Sayler, 2013). An amino functional group is present on position 2 and 8 of the purine ring (Cusick & Sayler, 2013). Each of these two amino groups are themselves a part of what is referred to as a guanidinium moiety (Cusick & Sayler, 2013). Here, a moiety simply means a significant part of the molecule. This guanidinium moiety is related to the guanidinium ion shown in Figure 2.

Figure 2: Chemical Structure of Guanidinium. From (PubChem Compound Summary for CID 32838, Guanidinium)
One guanidinium moiety occupies the position 1,2,3 of the molecule, with its characteristic amino group on position 2 (see Figure 1) (Cusick & Sayler, 2013). The other guanidinium moiety is located on position 7,8,9 of the molecule, with its characteristic amino group on position 8 (see Figure 1) (Cusick & Sayler, 2013). The 1,2,3 guanidinium group has a pKa of 11.28, whereas the 7,8,9 guanidinium group has a pKa of 8.22 (Cusick & Sayler, 2013). At physiological pH, both groups are protonated and carry a positive charge (Durán-Riveroll & Cembella, 2017). These guanidinium groups are permanent on the molecule; that is, they are present in all analogues of saxitoxin (Cusick & Sayler, 2013). There are four groups found on the outsides of the molecular structure that vary among the different saxitoxin analogues (Cusick & Sayler, 2013). These groups are labelled R1, R2, R3, and R4 in Figure 1 (Cusick & Sayler, 2013). The differing R groups are responsible for the relative toxicity of the molecule (Cusick & Sayler, 2013). Saxitoxin (STX) is the most toxic of the analogues. It is characterized by the presence of a carbamoyl group (OCONH2) at the R1 position and a hydrogen (H) at positions R2, R3, R4 (Cusick & Sayler, 2013). Saxitoxins are stable at high temperatures and low pH, making it a hard compound to excrete and metabolize for humans (Gad, 2014).
Marine neurotoxins, such as saxitoxins, interact with voltage-gated sodium, potassium, and calcium channels and modulate the flux of these ions into different cells (Cusick & Sayler, 2013). The primary target of saxitoxins is voltage-gated sodium channels of nerve and muscle cells. (Cusick & Sayler, 2013).
Voltage-gated channels (VGCs) are involved in the generation of electrical signals in cells (Terlau & Stühmer, 1998). VGCs are amphiphilic proteins embedded in the lipid bilayer of the cell membrane (Cusick & Sayler, 2013). They have a pore that allows passive diffusion of ions across the membrane when the protein is in a particular conformation (Cusick & Sayler, 2013). They exhibit specific ion selectivity (Terlau & Stühmer, 1998). They have voltage sensing domains that are very sensitive to changes in membrane potential (Blass, 2021).
The resting potential of the cell membrane is -70 mV (Blass, 2021). At this resting potential, the pore of the VGC is closed and no ions flow through (Blass, 2021). The stimulus for the activation of the VGC is depolarization of the membrane potential (Terlau & Stühmer, 1998). If the stimulus causes the membrane potential to rise above the threshold for activation, the channel will undergo conformational changes that allow ions to flow through the channel (Blass, 2021). In the case of sodium VGC, sodium ions rush into the cell, making the inside of the cell more positively charged. This is the depolarization phase (Figure 3) (Blass, 2021). Once a membrane potential of around 40 mV is reached, repolarization occurs (Figure 3). During repolarization, the sodium channels close, preventing the flow of sodium into the cell (Neuron action potentials: the creation of a brain signal). Meanwhile, potassium channels open, which allows positively charged potassium ions to leave the cell and brings the membrane potential back towards resting potential. Finally, there is hyperpolarization. Since potassium channels stay open a little longer before closing, the membrane potential becomes more negative than the resting potential (hyperpolarized); the sodium channel must reactivate temporarily to bring the membrane potential back to resting potential. The refractory period is when the VGC will not respond to another stimulus until the resting membrane potential is once again achieved (Figure 3) (Blass, 2021).
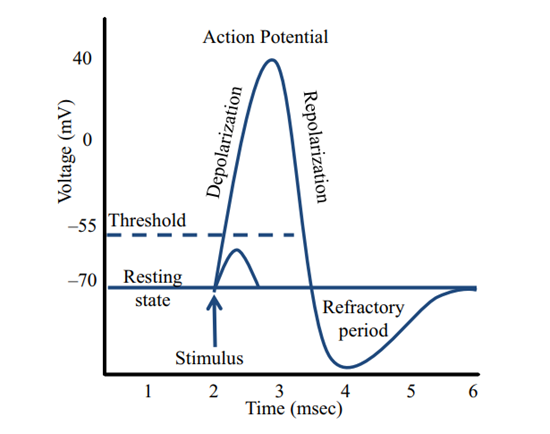
Figure 3: Electrical diagram of the voltage across the voltage-gated ion channel over time
VGCs generate electrical signals in nerve and muscle cells necessary for many cellular functions, such as contraction of muscles, secretion of hormones, sensing of the environment, processing of information in the brain, and output of electrical signals from the brain to peripheral tissues (Wang, 2008). Some dinoflagellates have evolved the ability to produce saxitoxins that block VGCs (Terlau & Stühmer, 1998). Only one saxitoxin molecule binds per VGC (Cusick & Sayler, 2013). Saxitoxins bind with high affinity to receptor site 1 of voltage-gated sodium channels in nerve and muscle cells (Cusick & Sayler, 2013). Receptor site 1 is made of two rings of amino acid residues. The 7,8,9 guanidinium moiety is the active group involved in the toxin binding (Cusick & Sayler, 2013). This positively charged guanidinium group is attracted to the negatively charged carboxyl group at the site (Gad, 2014). This binding blocks the sodium channel from the outside and prevents the inward flow of sodium into the cell (Cusick & Sayler, 2013). Consequently, the VGCs do not work properly.
Through the mechanism described, saxitoxin is known to be the causative agent of paralytic shellfish poisoning (PSP) in humans (Cusick & Sayler, 2013). PSP symptoms include paresthesia, numbness, muscular weakness, sensation of lightness and floating, ataxia, motor incoordination, drowsiness, incoherence and progressively decreasing ventilator efficiency (Cusick & Sayler, 2013). Death via intoxication of saxitoxin is usually a result of respiratory paralysis (Cusick & Sayler, 2013). Saxitoxin is one of the most potent natural toxins in nature, with a lethal dose in the low milligrams range for humans (Durán-Riveroll & Cembella, 2017). In areas of red tide (another expression for harmful algal blooms), people walking on the beach have reported difficulty breathing (Gad, 2014). This is thought to be due to inhalation of spray containing saxitoxin, further demonstrating the potency of this toxin (Gad, 2014).
Finding the genes associated with the biosynthesis of saxitoxins in dinoflagellates has been difficult for scientists (Cusick & Sayler, 2013). This is due to the size and complexity of the dinoflagellate genome (Cusick & Sayler, 2013). Their genome is highly redundant and contains a high gene copy number (Cusick & Sayler, 2013). However, modern genomic, proteomic, transcriptomic, and metabolomic methods are helping decipher how dinoflagellates biosynthesise saxitoxins (Akbar et al., 2020).
Saxitoxin is classified as a Schedule 1 Chemical Warfare Agents per the Chemical Weapons Convention of 1993 (Cusick & Sayler, 2013). Weapons using saxitoxins have been manufactured in the past but are now supposed to be decommissioned under the Chemical Weapons Convention of 1993 (Gad, 2014).
Finally, saxitoxin has been used as a chemical reagent for research purposes (Gad, 2014). It was used in experiments that helped explain the mechanisms involved in sodium channels used in cellular communication (Gad, 2014).
Other toxins
Interestingly, dinoflagellates also produce two toxins that are the two largest known continuous carbon atom chains found in a natural product (Cusick & Sayler, 2013). The first is a toxin called palytoxin (Figure 4). Palytoxin is produced by the dinoflagellate Ostreopsis siamensis (Cusick & Sayler, 2013). It is considered a polyketide. Polyketides form a group of compounds with diverse structures and biological activities (Cusick & Sayler, 2013). As a toxin, its only known target is the Na:K ATPase enzyme (Cusick & Sayler, 2013). It is the second largest known continuous carbon atom chain found in a natural product (Cusick & Sayler, 2013).

Figure 4: Chemical structure of palytoxin. From (Cusick & Sayler, 2013).
Finally, the dinoflagellate Gambierdiscus toxicus produces a polyketide toxin called maitotoxin (Figure 5) (Cusick & Sayler, 2013). It is considered a channel modifier; it allows non-selective ion passage through the channel (Cusick & Sayler, 2013). Maitotoxin is the longest know continuous carbon atom chain found a natural product (Cusick & Sayler, 2013).
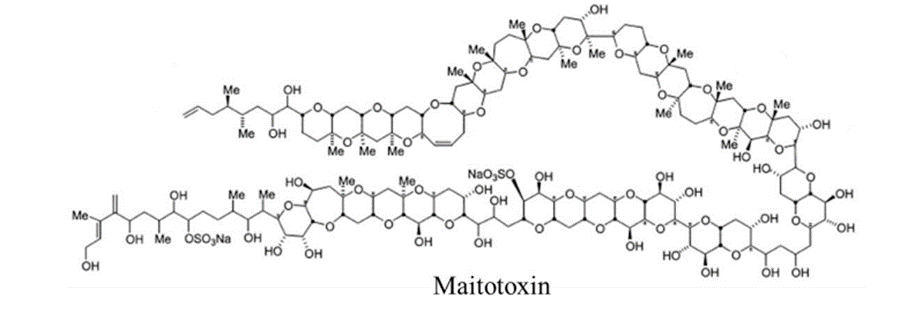
Figure 5: Chemical structure of maitotoxin. From (Cusick & Sayler, 2013).
Energy Sources and Nutritional Strategies
Dinoflagellates have diverse, and in many cases, flexible nutritional strategies that support autotrophic [1], heterotrophic [2], symbiotic [3], parasitic [4] and mixotrophic [5] lifestyles (Murray et al., 2016). Some of these strategies involve the active uptake of dissolved inorganic nutrients, osmotrophy (organic nutrition uptake), photosynthesis and phagotrophy (digestion of prey) (Murray et al., 2016).
[1] Autotrophs are organisms that produce new biomass from inorganic resources (carbon dioxide and mineral nutrients), using either light energy (photoautotrophs) or energy from reduced molecules in the environment (chemoautotrophs). (Sage, 2008)
[2] Heterotrophy is a mode in which in the absence of light, organic carbon dissolved in the culture media are used as substrates for energy production and growth in aerobic using respiration. (Hachicha et al., 2023)
[3] A mutualism among members of different species in which members of one species (the symbiont) live inside or on a body of the other (the host). (Leigh, 2017)
[4] A plant or an animal which lives upon or within another living organism at whose expense it obtains some advantage. (Acosta et al., 2020)
[5] Mixotrophy is the capacity to utilize more than one source of energy.
The Photosynthetic Apparatus
Photosynthesis is a complicated process that begins with photon absorption and ends with the export of stable carbon products (as illustrated in Figure 6). Two photosystems, I and II, catalyze the first step of this conversion. The two photosystems, coupled by a plastoquinone pool, the cytochrome b6f complex and the soluble electron carrier proteins plastocyanin (PC), operate the electron transportation in cascade. The electron transfer processes lead to differences in proton concentration across the thylakoid membrane, and the resulting electrochemical potential drives the synthesis of ATP by the ATP synthase. Finally, photosystem I reduces ferredoxin, providing the electrons for the reduction of NADP+ to NADPH by ferredoxin-NADP+-oxidoreductase (FNR) (Liu et al., 2020).
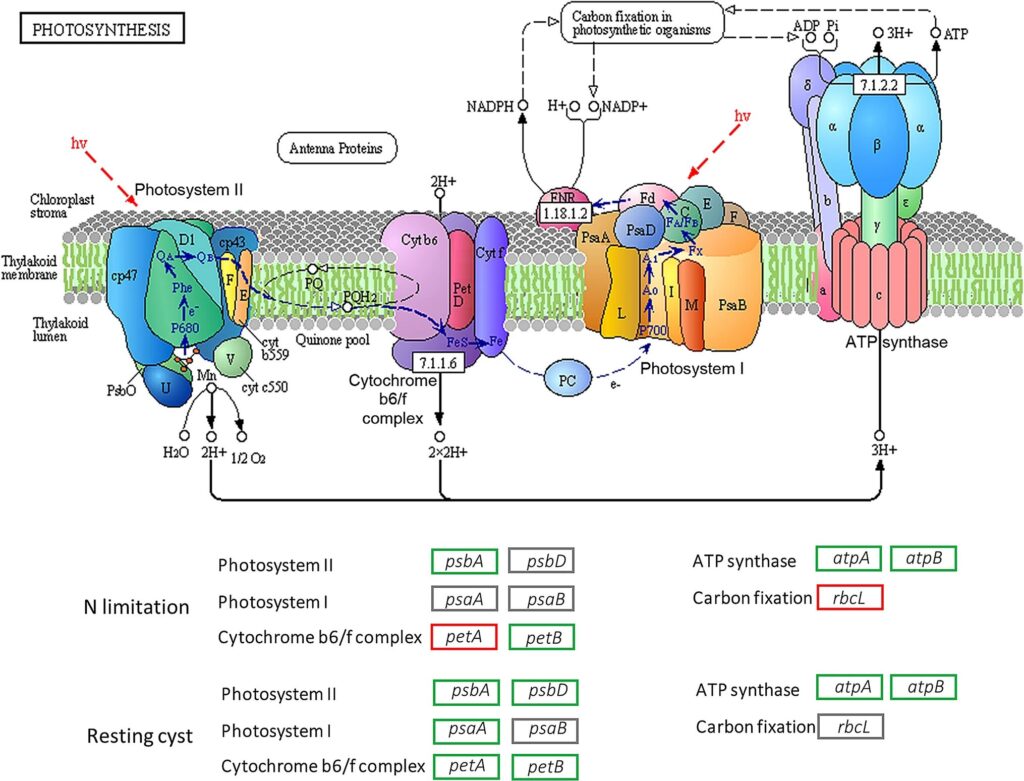
Figure 6: Illustration of the photosynthesis process (Liu et al., 2020).
A fundamental aspect of understanding photosynthesis in dinoflagellates lies in unraveling their unique pigment composition. The presence of peridinin (C39H50O7), which is an accessory carotenoid light harvesting pigment, distinguishes dinoflagellates from other photosynthetic organisms, imparting specific adaptive advantages in diverse marine environments. Most photosynthetic dinoflagellates possess plastids that contain peridinin. Peridinin-containing dinoflagellates have chloroplast genomes broken into single-gene minicircles that encode only 16 of the typically 130–200 plastid proteins (Koumandou et al., 2004). Gymnodinium cf. placidum containing peridinin plastids and the chemical structure of peridinin can be seen in Figure 7 and Figure 8.

Figure 7: Gymnodinium cf. placidum containing peridinin plastids. Plastids (p) are surrounded by three membranes, and nucleus-encoded proteins are trafficked to these plastids from the ER via vesicular transport through the Golgi (G) (Waller & Kořený, 2017).

Figure 8: 2D chemical structure of peridinin. (National Center for Biotechnology Information, 2023)
This plastid type has several definitive features, one of the most conspicuous being its pigment composition. The dominant secondary pigment is the carotenoid peridinin that is unique to dinoflagellates. This pigment is unusual for a carotenoid in containing three closed ring structures, and this is thought to contribute to its strong blue-light-absorbing properties (Rapoport et al., 1971). Furthermore, the peridinin plastid contains only a single chlorophyll c type (c2) in addition to chlorophyll a (Waller & Kořený, 2017). A second biochemically distinguishing feature of the peridinin plastid is its form II RuBisCO, atypical of eukaryotic types of this central photosynthetic enzyme (Morse et al., 1995). Form II RuBisCO assembles as homodimers, rather than the higher order heterodimers of the large and small subunits of form I RuBisCO found in other eukaryotic plastids and their cyanobacterial forebears (Waller & Kořený, 2017). The presence of this unusual RuBisCO suggests protein replacement via lateral gene transfer, most likely from proteobacteria where this RuBisCO form is also prevalent.
Kleptoplastidy (plastid retention from prey) is thought to represent an early stage of plastid acquisition by phagotrophs such as dinoflagellates, whereby a feeding cell temporarily sequesters the plastids of prey in order to benefit from the photosynthesis occurring in the stolen organelle (Stoecker, 2007). The process of phagocytosis allows dinoflagellates to recruit new, potentially adaptive genes through subsequent horizontal gene transfer. One of these genes in the toxin-producing Karenia brevis, is an electron transfer protein (plastocyanin) of green algal origin that likely allows this species to thrive under conditions of iron depletion (Nosenko et al., 2006). Genes encoding essential enzymes in the tricarboxylic acid cycle were found to have been laterally transferred from bacteria and a novel plastid-targeted fusion protein was found to have been transferred from a cyanobacterial species (Waller et al. 2006). The acquisition of these genes may have enabled the diversification of dinoflagellate nutritional strategies.
Regulatory Mechanisms, Environmental Responses and Circadian Rhythm
Mixotrophic dinoflagellates maintain a photosynthetic apparatus, mechanisms for phagotrophy and the uptake of complex metabolites such as vitamins or amino acids via osmotrophy, suggesting a broader array of functional requirements than their autotrophic or heterotrophic counterparts (Murray et al., 2016). These dinoflagellates specialize their metabolism to perform different tasks better at specific times of day. For example, in some dinoflagellates such as Lingulodinium polyedra, cells are specialized for photosynthesis during the day and bioluminescence and cell division at night. These rhythms are circadian as they are controlled by an endogenous circadian clock (Bowazolo & Morse, 2023). The metabolic rhythms follow coordinated changes in gene expression that occur at a translational level. These changes are revealed by ribosome profiling, a surrogate measure of protein synthesis rates. These dinoflagellates regulate the synthesis rate of over three thousand transcripts. Peak synthesis rates for the different transcripts are clustered around three different times over a light/dark cycle. (Bowazolo & Morse, 2023). The regulation of photosynthesis in dinoflagellates is a multifaceted process that is intricately linked to various internal and external cues. Circadian rhythms have been identified as key modulators of photosynthetic activity, orchestrating the temporal dynamics of light utilization and carbon assimilation.
Bioluminescence
Dinoflagellates’ bioluminescence has long been a source of wonder to civilizations. The first recorded incident of dinoflagellate bioluminescence dates all the way to 500 BCE (Valiadi & Iglesias-Rodriguez, 2013). These unicellular organisms are considered the main cause of bioluminescence observed in the ocean (Figure 9) (Hanley & Widder, 2017).

Figure 9: Dinoflagellate bioluminescence in Australia (Latz, 2019).
The Stimulus: What Triggers This Phenomenon?
Bioluminescence in dinoflagellate is said to be activated by shear stress (Maldonado & Latz, 2007). Simply put, shear stress refers to the force of movement of water on a creature. To trigger bioluminescence, shear flow has to be of 0.02 – 0.03 Nm – a threshold much higher than the normal fluid force of the ocean (Maldonado & Latz, 2007). This threshold is important as it allows bioluminescence to be a sustainable mechanism in these creatures. Indeed, bioluminescence consumes large amounts of energy and chemicals. Hence, this organism has had to evolve to react only to specific magnitudes of shear force rather than at any detection of force (Maldonado & Latz, 2007). The threshold of 0.02-0.03 Nm is significant as it corresponds to the force of shear force exerted on a dinoflagellate in the presence of a swimming predator (Maldonado & Latz, 2007). Above this threshold, the intensity of the bioluminescence produced increases with the shear stress (Maldonado & Latz, 2007). When this magnitude of shear stress is exerted on the cell, a mechanotransduction mechanism is triggered leading to the illumination of the species (Valiadi & Iglesias-Rodriguez, 2013).
The Process: What Happens inside the Cell?
As shear stress is applied on the cell membrane, a series of steps takes place to trigger bioluminescence. Firstly, in response to this mechanical stimulus, a G-protein coupled receptor found on the surface of the cell membrane is activated (Figure 10 (1)) (Valiadi & Iglesias-Rodriguez, 2013). The G-protein coupled receptor transfers this external mechanical signal to the inside of the cell for this signal to then be converted into an electrical signal (Valiadi & Iglesias-Rodriguez, 2013). This leads to the activation of transient receptor potential channels on the membrane, allowing for an influx of calcium ions inside of the cell (Figure 10 (2)) (Pozdnyakov, Matantseva, & Skarlato, 2021; Wang, 2022). This influx of positive charges inside of the cytoplasm causes the depolarization of the vacuole membrane due to the potential difference between the cytoplasm and the inside of the vacuole (Valiadi & Iglesias-Rodriguez, 2013). This change causes an action potential to flow through the vacuole membrane into the scintillon membrane attached to the vacuole [6] (Valiadi & Iglesias-Rodriguez, 2013). This electrical signal activates the opening of voltage gated proton channels found across the scintillon’s membrane (Figure 10 (3)). Once these channels are opened, the scintillon experiences an influx of protons (Valiadi & Iglesias-Rodriguez, 2013). This surplus of protons causes the acidification of the organelle, decreasing its pH from 8 to 6 (Figure 10 (4)) (Valiadi & Iglesias-Rodriguez, 2013). With this decrease of pH, the scintillon detaches from the vacuole and is now ready to hold the chemical reaction that emits the light (Wilson, 2013).
[6] Scintillons are unique organelles found in dinoflagellates. They contain the Luciferase enzyme, the Luciferin substrate and the Luciferin binding protein.

Figure 10: Process of bioluminescence in the cell. (1) Detection of shear stress by the g-protein receptor, (2) increase of Ca+ ions in the cytoplasm generating action potential, (3) activation of voltage gated ions on the membrane of the scintillon, (4) decrease of pH inside of the scintillon (Valiadi & Iglesias-Rodriguez, 2013).
The chemical reaction takes place inside of the scintillon organelle between the dinoflagellate luciferase (LCF) – the enzyme that catalyzes the reaction – and the luciferin (LH2) – the substrate of the reaction. The luciferin is bounded to a protein named the luciferin binding protein (LBP) (Hastings, Schultz, & Liu, 2009). When the pH level of a scintillon is 8, the LCF is inactive, and the luciferin is bounded to the LBP. This level of acidification inhibits a chemical reaction from taking place when not needed. Once the shear stress threshold is reached, and subsequently, the acidity of the organelle is decreased to 6, a change in conformation of the LCF takes place, leading to its activation and to the opening of binding sites for the luciferin substrate (Valiadi & Iglesias-Rodriguez, 2013). In parallel to this, the LBP also changes it conformation, freeing the luciferin substrate (Valiadi & Iglesias-Rodriguez, 2013). Hence, this acidification is key in generating the chemical reaction that triggers the emission of light (Figure 11).
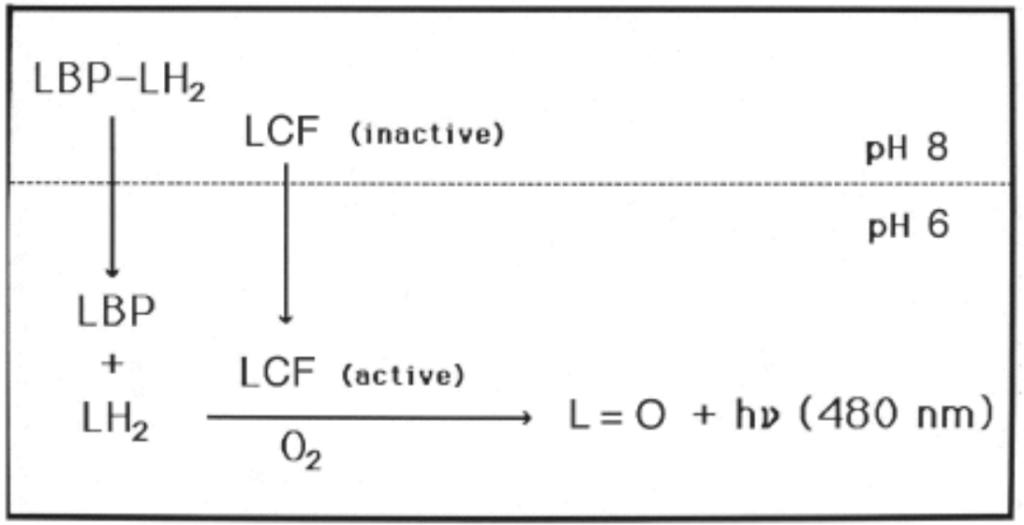
Figure 11: Activation of LCF and separation of LBP and LH2 due to a decrease in acidity of the scintillon organelle (Hastings, Schultz, & Liu, 2009).
The dinoflagellate luciferin is found to take the form of an open chain tetrapyrrole, hypothesized to have evolved from chlorophyll (Wilson, 2013). The dinoflagellate luciferin now reacts with O2 in the presence of luciferase resulting in the release of blue light (Figure 12) (Wilson, 2013). The time of this reaction, from the detection of the stimulus to the emission of light takes less than 20 ms (Valiadi & Iglesias-Rodriguez, 2013).

Figure 12: Chemical reaction between the dinoflagellate luciferin and O2 in the presence of luciferase. (Wilson, 2013).
The cause: why has nature evolved this way?
As aforementioned, dinoflagellates produce bioluminescence when experiencing an increase in shear rate, analogous to the one felt when a predator approaches. Hence, many have theorized that these creatures produce such a response as a defence mechanism. Three theories have been developed to explain how this unicellular organism uses bioluminescence as defence against predators.
To startle predators (Figure 13 (a))
This first theory hypothesizes that dinoflagellates have developed bioluminescence to startle their predators (Hanley & Widder, 2017). As the flash of light shocks the predator, it provides enough time for the dinoflagellate to escape. However, many have pointed out a flaw in this theory: the inevitable habituation of the predator to this technique. With time, predators will get accustomed to the flash of light and will no longer be startled. Hence, other theories have been developed (Hanley & Widder, 2017).
To call on secondary predators: a burglar alarm (Figure 13 (b))
The second and most supported hypothesis is the ‘burglar alarm’ theory. It is thought that the dinoflagellate, in the presence of its own predator, will emit light to attract the predator of its predator. In this sense, this technique would allow the dinoflagellate to escape as the bigger predator will take care of its aggressor (Hanley & Widder, 2017).
To warn predators of toxicity: an aposematic warning (Figure 13 (c))
This third theory presents bioluminescence as an aposematic warning to predators (Hanley & Widder, 2017). Certain dinoflagellates are known to be toxic, making them harmful to predators. Hence, dinoflagellates emit light to warn these predators of their toxicity. However, certain predators have been noted to being able to detect the difference between the two types. Some are even able to ingest the toxic dinoflagellates with minimal consequences (Abdulhussain et al., 2020). Hence, the second theory remains the most favoured amongst researchers.

Figure 13: Theorized uses of bioluminescence against predators. (a, top) To startle predators, (b, center) to call on a secondary predator, (c, bottom) to warn predators of toxicity (Wang, 2022).
Chemotaxis and Phototaxis
Because of the low Reynolds number environment of dinoflagellates, they swim in a helical pattern (Fenchel, 2001). Not only is this an effective way for dinoflagellates to swim, but it is also useful for the detection of changing concentration gradients (Fenchel, 2001). If a marine organism is swimming parallel to a potential nutrient source unidirectionally, it cannot detect the source of the nutrients easily. However, if it swims in a helical pattern, the nutrient source can be located by a rise and fall in the concentration of the chemical (Fenchel, 2001). Then, the dinoflagellate performs a specific combination of strokes at each concentration gradient to turn towards the nutrient. This method of swimming towards nutrients is incredibly effective, as Spero et al. reports that G. fungiforme dinoflagellates sense nutrients after 3 seconds, and start to swim towards it (Spero, 1985).
A specific form of chemotaxis that dinoflagellates use is known as helical klinotaxis. Helical klinotaxis is a phenomenon where organisms steer towards a source of a chemical gradient with the help of a helical movement pattern. Dinoflagellates must respond to both positive and negative changes in concentration gradient to move towards a source of nutrients. Blackburn et al. describe how helical swimming marine organisms path find using the changes in chemical concentration towards its nutrients. A simulation of a related protist, Strombidium shows how helical klinotaxis works for dinoflagellates (Blackburn & Fenchel, 1999). Suppose that a marine organism moves tangent to the source of nutrients. The dinoflagellate detects the lowering of concentration when moving past the target, and swims around towards the target by lowering its spin rate (Blackburn & Fenchel, 1999). Furthermore, dinoflagellates can maintain their current direction by increasing their spin rate (Blackburn & Fenchel, 1999). Blackburn et al. also noticed in the simulation that a response to both positive and negative stimuli are necessary for a marine protist to approach its target (Blackburn & Fenchel, 1999) as seen in Figure 14, where the organism can only effectively approach the source of chemicals if it responds to both positive and negative changes in chemical gradients.
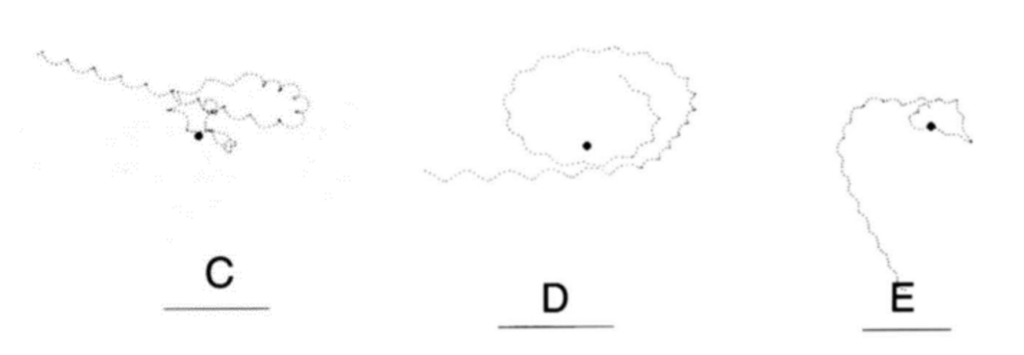
Figure 14: C: response to negative chemotaxis only D: response to positive chemotaxis only E: response to both positive and negative chemotaxis (Blackburn & Fenchel, 1999).
Dinoflagellates are incredibly sensitive to glycine (Hauser et al., 1974; Spero, 1985). Glycine is one of the simplest amino acids, with only one hydrogen as its side chain. It is quite prevalent in marine organisms. For example, the concentration of glycine in shrimp extract of Palaemonetes pugio is one order of magnitude higher compared to all other amino acids (Spero, 1985). Dying protists have a tendency to leak amino acids into the marine environment (Blackburn & Fenchel, 1999). Which means that it is plausible for dinoflagellates to chase after glycine for food. If they go towards glycine, it is likely that they will meet a dead organism with a whole range of nutrients. Therefore, glycine can be used as an indicator for nutrients.
Not only are dinoflagellates sensitive to changes in concentration gradients through chemotaxis, but they are also sensitive to concentrations of light. Some dinoflagellates perform photosynthesis to obtain nutrients, and therefore require light. However, gravitational forces pull dinoflagellates down, and therefore it is not worth it for the dinoflagellates to constantly swim near the surface of the ocean. Therefore, dinoflagellates perform what is known as phototaxis, where they would only swim closer to the surface of the water when there is sunlight (Kamykowski, Milligan, & Reed, 1998). Kamykowski et al. lit a chamber from 6 am until 10 pm, and it was observed that the dinoflagellates would slowly rise 80 centimeters from the bottom of the tank to the surface (Kamykowski, Milligan, & Reed, 1998). While light allows for dinoflagellates to perform photosynthesis, too much light can cause phototoxic effects. Therefore, dinoflagellates show negative phototaxis when the light is too strong.
Conclusion
Some dinoflagellates produce very potent toxins, such as saxitoxin. These toxins accumulate in filter feeders and transfer up the food chain. When humans consume seafood contaminated with the toxin, they risk developing illnesses such as PSP. The biologically active guanine group of the saxitoxin binds to the voltage-gated sodium channels of muscle and nerve cells, effectively blocking the flow of ions through the channel and preventing the generation of electrical signals. Toxins evolved as a solution to catch prey or to defend against predators more effectively by intoxicating them. The dinoflagellate Prorocentrum micans has different protein profiles when it grows autotrophically or mixotrophically, which suggests that the expression of genes may be switched on and off under different trophic modes, potentially reducing the overall cost to the organism (Shim et al., 2011). Two autotrophy-specific proteins showed homology to glyceraldehyde-3-phosphatase dehydrogenase (GAPDH) and a bacterial catalase, but three mixotrophy-specific proteins showed homology with hypothetical proteins that have completely unknown functions (Shim et al., 2011). Studies that identify genetic pathways involved in phagocytosis would allow for experiments on the ecological drivers of phagotrophy compared to autotrophy in mixotrophic organisms. Given the importance of these diverse nutritional strategies in the survival and proliferation of some dinoflagellate species, a deeper understanding of the genetics surrounding phagotrophy and kleptoplastidy (the mechanisms of retention of kleptoplastids in dinoflagellates) would allow for investigating their evolutionary ecology and determine the associations between genetic changes in specific lineages with particular functional traits (Murray et al., 2016).
Constraints faced by dinoflagellates in their environment have led to the evolution of specific design solutions. These creatures face predators, leading to the emergence of bioluminescence as a defence mechanism. Dinoflagellates have evolved to shine when they detect a certain magnitude of shear stress, analogous to that of a predator swimming. This has allowed dinoflagellates to only produce this high energy consuming reaction when necessary. The emitted light is used to either a) startle the predator, b) warn of potential toxicity and 3) call a secondary predator to take care of the aggressor. Dinoflagellates’ sensitivity to both positive and negative changes in chemical concentrations coincides with their helical swimming pattern, where the oscillations in chemical gradient can provide information on where a chemical is coming from. Dinoflagellates are also incredibly sensitive towards the amino acid glycine, as it is extremely prevalent in the ocean, and because they are generally excreted by dying organisms, providing dinoflagellates an efficient way to find feeding opportunities. Furthermore, their movement towards sunlight allows them to perform photosynthesis when there is enough light and sink back down otherwise to conserve energy.
References
Abdulhussain, A. H., Cook, K. B., Turner, A. D., Lewis, A. M., Elsafi, M. A., & Mayor, D. J. (2020). The Influence of the Toxin Producing Dinoflagellate, Alexandrium catenella (1119/27), on the Feeding and Survival of the Marine Copepod, Acartia tonsa. Harmful Algae, 98, 101890. https://doi.org/10.1016/j.hal.2020.101890
Akbar, M. A., Yusof, N. Y. M., Tahir, N. I., Ahmad, A., Usup, G., Sahrani, F. K., & Bunawan, H. (2020). Biosynthesis of saxitoxin in marine dinoflagellates: An omics perspective [Review]. Marine Drugs, 18(2), Article 103. https://doi.org/10.3390/md18020103
Blass, B. E. (2021). Chapter 3 – Classical targets in drug discovery. In B. E. Blass (Ed.), Basic Principles of Drug Discovery and Development (Second Edition) (pp. 111-183). Academic Press. https://doi.org/https://doi.org/10.1016/B978-0-12-817214-8.00003-8
Blackburn, N., & Fenchel, T. (1999). Modelling of microscale patch encounter by chemotactic protozoa. Protist, 150(3), 337-343.
Bowazolo, C., & Morse, D. (2023). Insights into daily metabolic changes of the dinoflagellate Lingulodinium from ribosome profiling. Cell Cycle, 22(11), 1343-1352. https://doi.org/10.1080/15384101.2023.2206771
Cusick, K. D., & Sayler, G. S. (2013). An overview on the marine neurotoxin, saxitoxin: Genetics, moleculartargets, methods of detection and ecological functions [Review]. Marine Drugs, 11(4), 991-1018. https://doi.org/10.3390/md11040991
Durán-Riveroll, L. M., & Cembella, A. D. (2017). Guanidinium Toxins and Their Interactions with Voltage-Gated Sodium Ion Channels. Mar Drugs, 15(10). https://doi.org/10.3390/md15100303
Gad, S. E. (2014). Saxitoxin. In P. Wexler (Ed.), Encyclopedia of Toxicology (Third Edition) (pp. 218-220). Academic Press. https://doi.org/https://doi.org/10.1016/B978-0-12-386454-3.00925-8
Hachicha, R., Elleuch, F., Ben Hlima, H., Dubessay, P., de Baynast, H., Delattre, C., Pierre, G., Hachicha, R., Abdelkafi, S., Fendri, I., & Michaud, P. (2023). Chapter 12 – Nonconventional treatments of agro-industrial wastes and wastewaters by heterotrophic/mixotrophic cultivations of microalgae and Cyanobacteria. In S. A. Bandh & F. A. Malla (Eds.), Valorization of Microalgal Biomass and Wastewater Treatment (pp. 239-260). Elsevier. https://doi.org/https://doi.org/10.1016/B978-0-323-91869-5.00002-8
Hanley, K. A., & Widder, E. A. (2017). Bioluminescence in Dinoflagellates: Evidence that the Adaptive Value of Bioluminescence in Dinoflagellates is Concentration Dependent. Photochem Photobiol, 93(2), 519-530. https://doi.org/10.1111/php.12713
Hastings, J. W., Schultz, W., & Liu, L. (2009). Dinoflagellate Bioluminescence and Its Circadian Regulation. Department of Molecular & Cellular Biology, Harvard University & Department of Structural Biology, Hauptman-Woodward Medical Research Institute, The State University of New York at Buffalo. Retrieved 2023-11-07 from https://www.csun.edu/~ll656883/pdfs/paper9.pdf
Koumandou, V. L., Nisbet, R. E., Barbrook, A. C., & Howe, C. J. (2004). Dinoflagellate chloroplasts–where have all the genes gone? Trends Genet, 20(5), 261-267. https://doi.org/10.1016/j.tig.2004.03.008
Latz, M. (2019). The Making of Infinity Cube , a Bioluminescence Art Exhibit. Limnology and Oceanography Bulletin, 28. https://doi.org/10.1002/lob.10345
Leigh, E. G. (2017). Cooperation, Evolution of☆. In Reference Module in Life Sciences. Elsevier. https://doi.org/https://doi.org/10.1016/B978-0-12-809633-8.02340-2
Liu, Y., Chen, T., Wang, X., Song, S., & Li, C. (2020). Variation in the photosynthetic activities of the dinoflagellate Akashiwo sanguinea during formation of resting cysts. Marine Biology, 167(11), 158. https://doi.org/10.1007/s00227-020-03774-y
Maldonado, E. M., & Latz, M. I. (2007). Shear-Stress Dependence of Dinoflagellate Bioluminescence. Biological Bulletin, 212(3), 242-249. https://doi.org/10.2307/25066606
Morse, D., Salois, P., Markovic, P., & Hastings, J. W. (1995). A Nuclear-Encoded Form II RuBisCO in Dinoflagellates. Science, 268(5217), 1622-1624. https://doi.org/10.1126/science.7777861
Murray, S., Suggett, D., Doblin, M., Kohli, G., Seymour, J., Fabris, M., & Ralph, P. (2016). Unravelling the functional genetics of dinoflagellates: a review of approaches and opportunities. Perspectives in Phycology, 3. https://doi.org/10.1127/pip/2016/0039
National Center for Biotechnology Information (2023). PubChem Compound Summary for CID 5289155, Peridinin. Retrieved November 5, 2023 from https://pubchem.ncbi.nlm.nih.gov/compound/Peridinin.
Neuron action potentials: The creation of a brain signal. Khan Academy. Retrieved November 05 from https://www.khanacademy.org/test-prep/mcat/organ-systems/neuron-membrane-potentials/a/neuron-action-potentials-the-creation-of-a-brain-signal
Nosenko, T., Lidie, K., Dolah, F., Lindquist, E., Cheng, J.-F., Bhattacharya, D., & Carver, R. (2006). Chimeric Plastid Proteome in the Florida ”Red Tide” Dinoflagellate. Mol Biol Evol, 23.
Pozdnyakov, I., Matantseva, O., & Skarlato, S. (2021). Consensus channelome of dinoflagellates revealed by transcriptomic analysis sheds light on their physiology. Algae, 36(4), 315-326. https://doi.org/10.4490/algae.2021.36.12.2
PubChem Compound Summary for CID 32838, Guanidinium. National Center for Biotechnology Information (2023). Retrieved November 04 from https://pubchem.ncbi.nlm.nih.gov/compound/Guanidinium
Rapoport, H., Strain, H. H., Svec, W. A., Aitzetmueller, K., Grandolfo, M. C., Katz, J. J., Kjoesen, H., Norgård, S., & Liaaen-Jensen, S. (1971). Structure of peridinin, the characteristics dinoflagellate carotenoid. Journal of the American Chemical Society, 93, 1823-1825.
Sage, R. F. (2008). Autotrophs. In S. E. Jørgensen & B. D. Fath (Eds.), Encyclopedia of Ecology (pp. 291-300). Academic Press. https://doi.org/https://doi.org/10.1016 /B978-008045405-4.00851-X
Shim, J.-B., Klochkova, T., Han, J. W., Kim, G. H., Yoo, Y.-D., & Jeong, J. h. (2011). Comparative proteomics of the mixotrophic dinoflagellate Prorocentrum micans growing in different trophic modes. Algae, 26, 87-96. https://doi.org/10.4490/algae.2011.26.1.087
Stoecker, D. (2007). Stoecker DK.. Mixotrophy among Dinoflagellates. J Eukaryot Microbiol 46: 397-401. Journal of Eukaryotic Microbiology, 46, 397-401. https://doi.org/10.1111/j.1550-7408.1999.tb04619.x
Terlau, H., & Stühmer, W. (1998). Structure and function of voltage-gated ion channels [Review]. Naturwissenschaften, 85(9), 437-444. https://doi.org/10.1007/s001140050527
Valiadi, M., & Iglesias-Rodriguez, D. (2013). Understanding Bioluminescence in Dinoflagellates-How Far Have We Come? Microorganisms, 1(1), 3-25. https://doi.org/10.3390/microorganisms1010003
Waller, R. F., & Kořený, L. (2017). Chapter Four – Plastid Complexity in Dinoflagellates: A Picture of Gains, Losses, Replacements and Revisions. In Y. Hirakawa (Ed.), Advances in Botanical Research (Vol. 84, pp. 105-143). Academic Press. https://doi.org/https://doi.org/10.1016/bs.abr.2017.06.004
Wang, A. (2022, August 8, 2022). Shining Blue Stars in the Ocean. Saltman Quarterly https://sqonline.ucsd.edu/2022/08/shining-blue-stars-in-the-ocean/
Wang, D. Z. (2008). Neurotoxins from marine dinoflagellates: A brief review [Review]. Marine Drugs, 6(2), 349-371. https://doi.org/10.3390/md6020349
Wilson, T. (2013). Harvard University Press. https://doi.org/doi:10.4159/harvard.9780674068025