Abstract
Many organisms possess an internal defense mechanism, intrinsic properties, and behaviors adapted for their survival. Spines and quills differ immensely across families, and this disparity is exacerbated further amongst taxa. Environmental pressures such as resource availability can result in various chemical and structural design mechanisms for spines and quills. However, despite distinct compositions, the spines of animal species have still developed similar purposes. Organizationally speaking, chemical properties translate to physical and structural properties. Even with different chemical compounds, the overall structure and strength of these spines are virtually invariable. There are numerous distinctions and parallels between spine and quill structures across organisms, such as protein and mineral contributions. More specifically, this essay delves into the composition and chemical properties of spines belonging to various organisms.
Introduction
Spines are used by a variety of organisms with a plethora of different compositions. Often, spines are derivations of hair, mainly composed of keratin, but with a different structure for spines. However, the proteins that make up spines across species are not well conserved, and different environmental pressures seem to have led to different proteins with the same purpose. Essential structural proteins like keratin and collagen combine to form different extracellular matrices, which are essential for forming strong spines. Porcupine fish spines contain collagen and hydroxyapatite, also found in the bones of other fish species. While individual species tend to have different proteins that form their spines, the overall chemical composition of these spines remains fairly similar. For example, the main component of many kinds of spines is calcium. The components that make up these spines are similar to those that make up bones and other hard-protecting layers like shells. This essay delves into the structural features of mammal spines like porcupines and hedgehogs, along with those of fish and creatures in aquatic environments. Lastly, the essay explores the formation of and the materials used in sea urchin spines. Even with the protein variation, the spines’ overall raw material content remains similar across species.
Mammal Spines
It is challenging to define a spine since its structure, function, and composition vary across animal orders. In mammals, such as hedgehogs, tenrecs, spiny rats, or porcupines, spines result from multiple evolutions of hair. Over time, animals have evolved structures such as spines to survive. Their function, however, is not limited to defense. Spines can be seen as hair that has been stiffened and strengthened. They are made of the same material as hair, keratin, and, more specifically, hard alpha-keratin. Hard keratin is higher in sulfur than soft keratin (McKittrick et al., 2012).
Keratin is a protein found in hair and spines but also in nails, hooves, and horns. Alpha-keratin is made up of two polypeptide chains curled in a helix shape. Cysteine is the amino acid present in higher quantities. Its hierarchical structure consists of intermediate filaments (IF) embedded in an amorphous keratin matrix. The intermediate filaments, as shown in Figure 1, are made up of protofilaments. Those protofilaments present non-helical N- and C-terminal domains that bond with other filaments and matrix (low-sulfur proteins compose the IFs while the matrix consists of high-sulfur and high-glycine–tyrosine proteins). The protofilaments are formed of two rows of tail-to-head coiled coils (dimer). Finally, two alpha-helix chains cross-linked with disulfide bonds between adjacent cysteine residues form a dimer (McKittrick et al., 2012).

Fig. 1 Structure of Alpha-Keratin. Intermediate filaments are the basic structural unit of keratin, which has a helix shape (McKittrick et al., 2012).
Mammal quills consist of two parts: the cortex, the outer layer of the spine, and the core, which is the foam-filled interior of the spine. The internal structure of spines differs from one mammal to another, which impacts the properties of the spines. For example, Old World porcupine quills present longitudinal stiffeners, while hedgehog quills contain longitudinal and transverse stiffeners. A greater alignment of the IFs will lead to greater tensile strength. The longitudinal sections of each quill present alpha keratin intermediate filaments oriented longitudinally (Yang & McKittrick, 2013). Figure 2 shows the three layers composing the core of a North American porcupine quill.
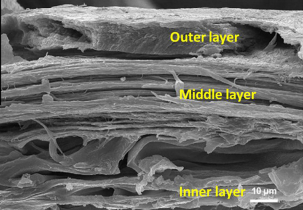
Fig. 2 The fracture surface of a porcupine quill shell with its three distinct layers (Chou et al., 2012).
The inner layer reinforces foam attachment. The outer layer contains hydrophobic lipids and, therefore, less water, which makes it less ductile than the other layers. This layer acts as a barrier, preventing water molecules from entering the spine since humidity affects the properties of the spine. The middle layer clearly shows the orientation of the fibers in the longitudinal direction of the quill. This alignment of the fibers gives the spine a higher tensile strength (Chou et al., 2012).
Mechanical properties of keratin depend on hydration, relative humidity, and temperature. Indeed, flexural strength and modulus keratin material decrease with humidity. For example, Young’s Modulus of a wet hedgehog quill is 2.3 GPa, while its value is higher (3.8 GPa) for a dry quill (McKittrick et a.l, 2012). The matrix-filament structure of the quill explains this phenomenon: intermediate filaments are insoluble in water, while the matrix absorbs water. This interaction of water molecules with the amorphous matrix breaks hydrogen bonds, which are responsible for the molecule’s stability. This increases the mobility of the fibers within the matrix, decreasing the stiffness of the spine (Lazarus, 2021). Moreover, the flexural strength of the spine is increased when it is conditioned at high temperatures. It is suggested that the elevation of temperature leads to forming more intermediate filaments, increasing material strength. Also, microfibrils may decrease water absorption since they are insoluble in water (Kennedy et al., 2017).
Keratin is a lightweight but also impact-resistant material. The stiff cortex and porous foam maximize its flexural strength/weight ratio. The hierarchical structure of keratin directly influences the toughness of keratin materials. Indeed, the levels of organization of a keratin-based material enable the spine to absorb more energy before failing (Lazarus, 2021).
Figure 3 below depicts the characteristic peaks of other keratin-based materials, like various amide groups and different content of amides. Interestingly these proteins also have sulfur-oxygen bonds, which tend to increase the overall strength of the respective compound (Torres et al., 2014).
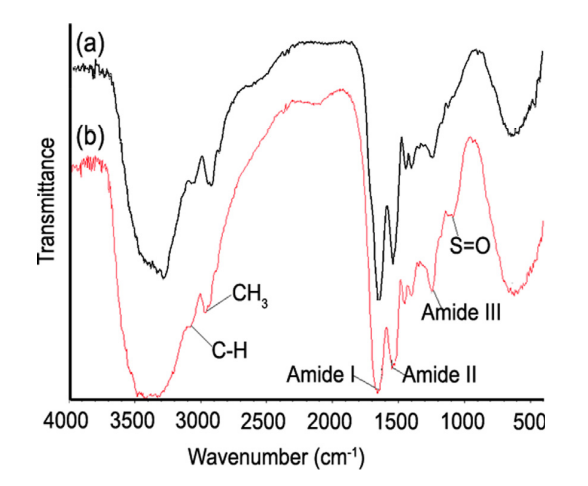
Fig. 3 Fourier transform infrared (FTIR) spectra of the (a) internal core and the (b) external shell of a representative porcupine sample (Torres et al., 2014).
Besides possessing interesting chemical compositions, spines can serve other chemical purposes. For example, porcupine spines are covered with free fatty acids that have been proven to provide antibiotic properties (Roze et al., 1990).
Interestingly, these free fatty acids seem only to prevent the growth of gram-positive bacterial strains seen in Figure 4. It is thought that they only affect these strains since the gram-positive strains lack an outer membrane but are instead made up of peptidoglycans.
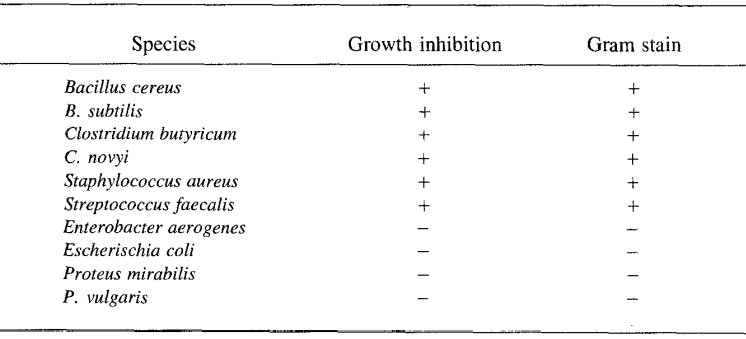
Fig. 4 Bacterial Growth Responses to Porcupine Quill Fatty Acids (Roze et al., 1990).
The antibacterial properties of these free fatty acids are thought to protect the animal from infection should its spines pierce themselves. This is often a common occurrence since porcupines tend to fall out of trees often, which can result in self-piercing (Roze et al., 1990). Free fatty acids have been extensively studied for various biomaterial applications thanks to their antibacterial properties, and they are already used in various commercial products (Casillas-Vargas et al., 2021).
The main strength of porcupine and hedgehog spines is derived from their keratin content and the formation of the distinct layers in this way. Keratin has been extensively studied for various hair-regenerating applications and other wound-healing capabilities. When we look at other species, we see that the same protein of importance is not always maintained; however, the composition of the spines tends to be quite similar.
Fish Spine Chemical Composition
Many fish contain spines embedded within their skin and scales. Porcupine and puffer fish possess spines and are in the Tetraodontiformes order and within the family of Diodontidae. They require spines to defend themselves from the jaws of predators. Diodon hystrix, a species of porcupine fish (Figure 5), was examined in a study to evaluate the chemical composition of its spines (Su et al., 2017). The spines of the porcupine fish are anatomically segmented, as shown in Figure 6. The spinous process of a porcupine fish spine is exposed, while lateral and axial processes are embedded within the fish’s skin.

Fig. 4 An image of Diodon hystrix; it is referred to as the ‘spot fin’ porcupine fish (Peppino, 2021).
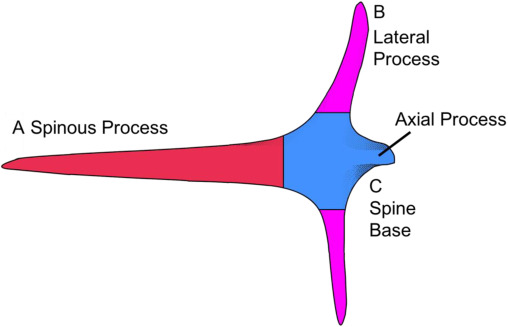
Fig. 5 The segments of a porcupine fish spine are labeled. The spine base at C is embedded within the fish, and the spinous process protrudes from the fish’s body (Su et al., 2017).
The spinous processes and the spine’s base elemental composition were determined (Su et al., 2017). There is a high carbon content (57.9%) and low calcium (0.4%) content in the base of the spine (Table 1). The significant presence of carbon indicates that the spine base is composed primarily of organic materials. The spinous process has the organic component of collagen. The spinous process is high in calcium and phosphorus due to the presence of hydroxyapatite in the spinous process of the porcupine fish. Hydroxyapatite (HAp) is significant in bone composition; this mineral surrounds bone fibrils (Currey, 2006).
Element | Spine Base(%) | Spinous Process(%) |
C | 57.9 | 16.3 |
O | 29.7 | 40.1 |
P | 12.1 | 25.4 |
Ca | 0.4 | 23.9 |
Collagen is the most abundant structural protein in the extracellular matrix. Fish have collagen in their connective tissues, such as skin and bone. Aquatic life with spines, such as porcupine fish, pufferfish, and zebrafish, all possess spines primarily reinforced with collagen. Interestingly collagen is starting to be used in various industrial applications, from scaffolds for tissue engineering to cellulose material for food packaging. Collagen can be purified and extracted from numerous sources, and it is a very valuable compound since it offers biocompatibility across species and is biodegradable. Collagen in fish vertebrates assists in storing, dissipating, and transmitting energy from exogenous forces. In fish, collagen composes 25-30% of proteins within their bodies. Three alpha-helical polypeptide chains form collagen; this protein conformation is exceptionally stable due to covalent linkage between proline and lysine. The molecule’s stability is required to maintain its structure at physiological temperatures. The covalent bonds responsible for the three helices within the molecule are between hydroxyl groups on proline and lysine residues. After these amino acids have undergone hydroxylation in the endoplasmic reticulum, they are secreted from the Golgi apparatus in their triple conformation into interstitial matrices. These alpha helices contribute to its functionality as a structural protein due to the inter-residue covalent linkages between the hydroxyproline and hydroxylysine residues within the helix (Calonje et al., 2018).
X-Ray diffraction (XRD) is utilized to confirm the presence of HAp in porcupinefish. This method confirms the crystallite structure of unknown compounds by measured diffraction due to the organization of atoms. A crystal structure is the regular repetition of atom patterns bounded by equally spaced planes. Crystals can act as a three-dimensional diffraction pattern for X-rays. The wavelength used by an X-ray corresponds to interatomic distances within the crystal (Garcia-Granda & Montejo-Bernando, 2005). Broad peaks indicate the sample is nanocrystalline (Figure 7), and crystalline sizes are calculated as 20.1nm, 20.4 nm, and 20.8nm from Equations 1 and 2. The XRD of porcupine fish spines matches that of HAp, indicating its chemical presence.

Fig. 7 The XRD rows descending from the top of D. hystrix are the spinous process, lateral process, and spines base. The bottom row is the XRD pattern for HAp powder (Elliot et al., 1973). The XRD of D. hystrix correlates to HAp, indicating its composition in the spines (Su et al., 2017).
Aside: If an incident wavelength λ, 0.15406 nm (Su et al., 2017) in XRD is applied at an incident angle θ ; this will be applied to planes within the crystal’s geometry, spaced ‘d’ apart (Fig.8). The reflected wave and the refracted wave within the crystal geometry will combine with positive interference if the wavelength difference is a multiple of the initial wavelength. Positive interference of wavelengths results from multiple wavelengths when their maxima and minima both align. This corresponds to the positive addition of wavelengths. The wavelength difference required for positive interference corresponds to nλ, the nth multiple of the initial wavelength (Garcia-Granda and Montejo-Bernando, 2005).
These sizes were calculated with the Scherrer equation, which manipulates the Bragg law to calculate crystal size.

D represents the average crystal sizes of HAp (20.1 nm, 20.4 nm, and 20.8 nm), λ is the X-ray wavelength of 0.15406 nm from the X-ray. 𝛃 is half of the width of XRD peaks corresponding to the three maximum peaks of the XRD data from D. hystrix. 𝛝 is the Bragg angle from the Bragg law:

Equations (1) and (2) are crucial for determining crystal size from XRD. The Sherrer equation and the Bragg law were used to calculate the sizes of the crystals, D, from the XRD of the porcupine fish spines. This mathematical analysis of HAp is possible due to the equally spaced planes in the crystal lattice (Figure 8), which provide a surface ideal for XRD.

Fig. 8 The Bragg reflection process utilizes parallel incident waves to calculate the path difference n𝝀. This difference is equal to 2dsin𝛝. The equidistant-spaced lines represent the planes within the crystal lattice (Garcia-Granda & Montejo-Bernando, 2005).
Fish Spine Structure
The spines are approximately 10 mm long and 1 mm in diameter. The water in the interstitial matrix of bone collagen fibrils can be replaced with minerals. Bridges connect longitudinally aligned mineralized collagen fibrils to form sheets, as shown by orange segments in Figure 9. These mineralized sheets are 2 μm thick. Unmineralized collagen fibrils, 0.5 μm in width, are radially aligned and wedged between the sheets. These segments are shown in green in Figure 9. The alternation of mineralized sheets and collagen fibrils assists in deflecting cracks and strengthening the spine during bending. While the increase in minerals typically corresponds to a stiffer and more brittle spine structure, the alternation of both forms of collagen maximizes strength from mineral additions and crack resistance from the more flexible unmineralized collagen (Jager & Fratzl, 2020). The collagen conformation in fish spines utilizes mineralized and unmineralized types to form a stress-resistant structure, as shown in Figure 9.
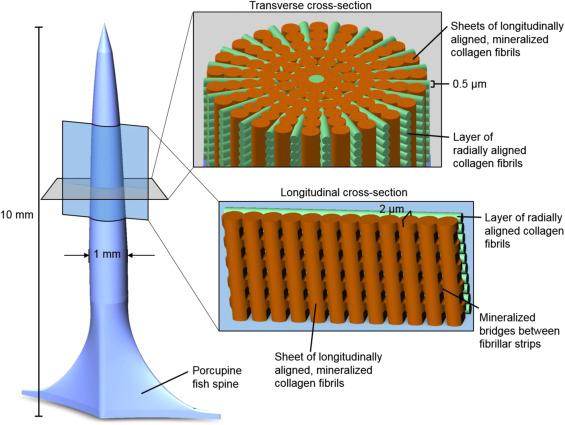
Fig. 9 A diagram of D. Hystrix spine. Mineralized collagen sheets are shown in orange, and unmineralized radial collagen fibrils are green (Su et al., 2017).
Also in the order of Tetraodontiforms is the Palembang puffer, Tetraodon steinachneri. Collagen is densely packed to form the outer, hydrophilic layer of T. steinachneri spines with a mineral core. The hollow bottom region, with its dermal cells connected via collagen, extends to the spine’s tip, forming a sharp point (Hertwig et al., 1992). The collagen fibrils extend outwards from the spine branch with nerve fibrils in the center (Figure 10). At rest, T. steinachneri spines are retracted; they extend while the fish is under threat (Figure 11).

Fig. 10 Nerve fibrils (nf) extend into the spine skeleton (sk) in between the collagen fibril bundles (cbf) from the skin or modified dermis region (md). Large vacuoles (vc) for storage are at the tip of the spine (Hertwig et al., 1992).

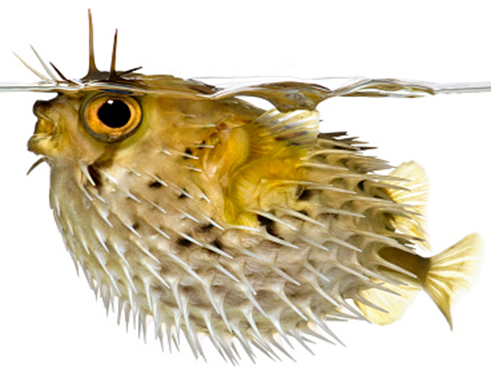
Fig. 11 On the left, a puffer fish at rest with its spines retracted. On the right, a puffer fish with its spines extended for protection (Bone, 2003).
Collagen is a critical component of proteins within fish. It composes 4.7% of the total protein content in the spiny lantern shark, 2.4% in the catfish, and 3.0% in the catfish (Sikorski et al., 1984). Collagen fibrils are within the skin of many fish species, like sea urchins and pufferfish. There are 29 forms of collagen, numbered in the order of their discovery. Type I collagen is organized in fibrils, forming extracellular matrices, tendons, bones, and the skin of fish. Furthermore, type I collagen is responsible for the mechanical protection of porcupine and puffer fish, as type I collagen forms the spines of these fish (Ikoma et al., 2003).
Chemical Composition of Sea Urchins
The composition of sea urchins’ spines differs significantly from porcupine or hedgehog spines. Its main component is a mineral rather than a protein. The formation of mineral skeletons in marine animals dates back to the Cambrian period (600 million years ago). Other marine organisms, such as corals, scallops, sea stars, and crabs, also use biomineralization to form skeletons by deposition of amorphous calcium carbonate (ACC). The ACC is then transformed into calcite. The spine also contains glycoprotein components that provide flexibility and enhance toughness since calcite is a brittle material (Drozdov, 2016). Some of those glycoproteins are the complex N-glycoproteins that are usually located near the growing amorphous section of the spine, while complex O-glycoproteins are at the base of the spine where skeletal growth is inhibited, thus indicating that the former contributes to spine growth while the latter is the cause of growth inhibition (Ameyea et al., 2001). There are also Spicule Matrix proteins, or SM proteins, scattered all around the spine skeleton (Ameyea et al., 2001). Needless to say, these highly complex proteins are the ones responsible for building up the spine from amorphous calcium carbonate and providing it with its porous interior, thus making it far more flexible at a low cost of toughness.
Every spine of sea urchins is composed of calcite single crystals, though its exact chemical formula and proportion of elements may vary between species, as shown below. The vast majority of the spine’s weight comes from calcium or magnesium carbonate. Additionally, a certain amount of sodium, chlorine, and sulfur can be found in the spines, depending on the sea urchin species. The following analysis will focus only on the Arbacia lixula, Paracentrotus lividus, and Sphaerechinus granularis.
As expected from the above, the weight percent of all ions in their spines combined is around 30% (Figure 12), with calcium being the most abundant at a weight percentage almost always above 25%. Trace amounts of magnesium, sulfur, sodium, and chlorine can also be found at a weight percent of around 1%. The remaining weight percent mostly comes from the countless carbonate ions that bond with those mentioned above (Varkoulis et al., 2020). In addition, it is clear from Figure 12 that some elements vary more than others. For example, calcium concentration is consistently higher within the Paracentrotus lividus, around 27% (Figure 12), while the magnesium concentration is always the highest within the Sphaerechinus granularis at above 10%. As for chlorine, sulfur, and sodium, those ions constantly fluctuate between all three species.

Fig. 12 Measure of weight percent or ratios of various ions in the spines of three species of sea urchins, the Arbacia lixula (Arb), Paracentrotus lividus (Par), and the Sphaerechinus granularis (Sph). The dark gray bars represent the spines’ percentages (Varkoulis et al., 2020).
Using the variation of values in Figure 12, one can tell which element varies the most depending on the season, though it is hard to tell if there is a cause or if it is changing due to pure coincidence. Some abiotic factors commonly mentioned were temperature, salinity, and pH levels (Varkoulis et al., 2020).
Indeed, researchers have investigated other abiotic factors; for example, they measured overall temperature and salinity throughout the year. There has been a spike in temperature around July, while there has been a spike in salinity around April (Figure 13). Nevertheless, the trend of ions in the spines did not show any significant change in reaction to those spikes. The sole exception for that is the amount of sulfur, with an average of around 0.4% weight total more in summer or autumn than in winter for all species (Varkoulis et al., 2020). Scientists believe that the change in ion proportion could be because of a change in diet. The Paracentrotus lividus and the Sphaerechinus granularis have been shown to change their diet in case their preferred meal becomes scarce. This selective feeding could cause the seasonal change of elements in their spines (Varkoulis et al., 2020). Moreover, this feeding behavior is not as typical in sea urchins belonging to the Arbacia Lixula, the species with the most stable element composition (Varkoulis et al., 2020), thus reinforcing this hypothesis even more. As such, one can reasonably claim that, for these three specific species, environmental variations do not directly affect the sea urchin’s spine composition. Consequently, this leaves us to believe that these environmental variations instead affect biotic or abiotic aspects of the sea urchin’s environment, which then indirectly affects the urchin’s spine composition. For example, one plausible hypothesis could be the more significant growth and abundance of algae in Greece during summer. Knowing that sulfur is one of the main components of algae cells, it is possible that the sea urchin consumed an excess of it. As a result, the spike in temperature measured in summer (Figure 13) could easily explain the higher sulfur concentration measured subsequently in early autumn (Figure 13) (Giordano et al., 2008).
Interestingly, when we generally think of how nutrients get repurposed in other animals, we usually see the ingested compound broken down into Acetyl-CoA, which can then be used to create various necessary compounds. Sea urchin spine composition is interesting because it appears that the composition of the spine can vary based on what nutrients are widely available for consumption at a given time. This suggests that for different nutrients they consume, they are more directly integrated into the spine without being first broken down into a universally common compound that can be repurposed for various uses. Nutrients in sea urchin spines are repurposed for use much faster than in systems where nutrients get broken down much more comprehensively.
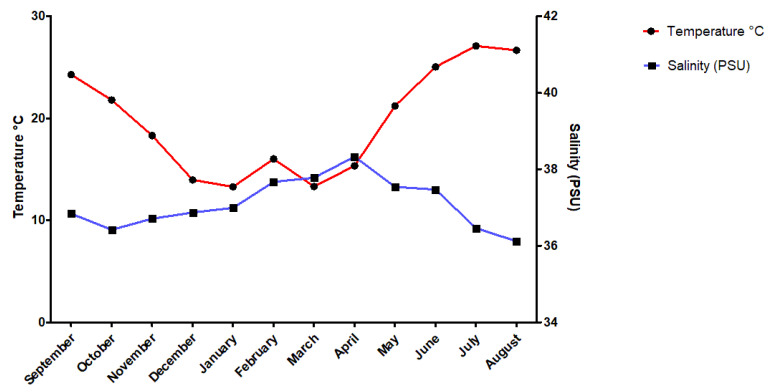
Fig. 13 Diagram displays the temperature and salinity trend from September 2017 to August 2018, at Agios Stefanos, Greece. Data was collected at a depth of 1 to 12 meters deep at several locations with hard substrates and rocks or soft substrates with sand to represent the target species’ natural habitat accurately. Note how the spike in temperature persists by early autumn (Varkoulis et al., 2020).
Biomineralization and Spine Regeneration
It is now clear that sea urchins form their numerous spines from calcite crystals. However, it is hard to understand where precisely those minerals come from and how they turn into a single crystal spine.
Sea urchins can consciously move underwater by moving their spines back and forth in a rotational motion, similar to how one would walk with dozens of stilts. Hence, they scour the sea floor looking for algae, kelp, plankton, and other organisms they can easily reach. Indeed, these sources of nutrients do contain carbon and minerals that eventually turn into the sea urchin’s spine. However, since the sea urchin heavily relies on its spines for locomotion and protection, it is only natural that they may break throughout the spiky creature’s lifetime. In these scenarios, the sea urchin has a relatively quick protocol that allows it to repair its broken limbs to its original size and quality.
When a spine is partially or entirely broken, the healing process happens at the furthest intact point of the spine, whether or not it is partially or fully broken. In order to reform a brand new spine, clumps of nanosized amorphous calcium carbonate are slowly transported to the broken area and solidified into the complex porous structure of the sea urchin’s spine. Although the process sounds simple, the whole repair requires the aid of several proteins that guide all the elements to the correct place and in the correct orientation.
Take the Strongylocentrotus purpuratus as an example; its healing begins seven days after the spine has been totally removed (Vinnikovaa et al., 2011). Spicules (Figure 14), small six branched-spikes, are first formed in a syncytium (Politi et al., 2008), an assembly of multiple specialized cells, and then transported inside a vesicle that travels from the base of the spine to the location of repair (Politi et al., 2008). That spicule is referred to as a larval spicule because it is still composed of amorphous calcium carbonate, and it will continue to change as it branches out (Figure 14). Finally, this initial stage is also characterized by the hydrated ACC, thus providing the material with a better capacity to be shaped (Politi et al., 2008).
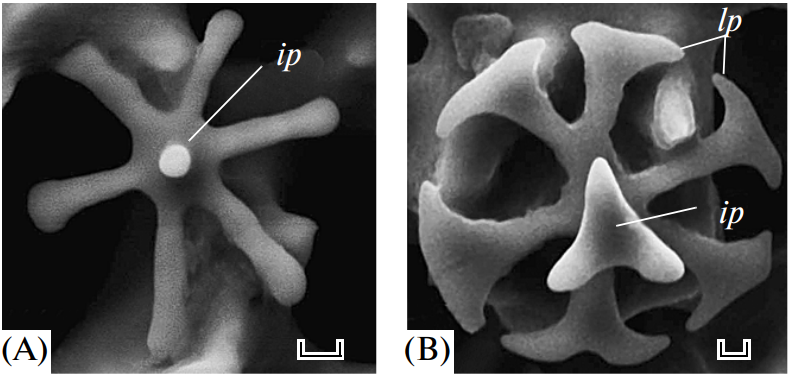
Fig. 14 The above images were taken with SEM. Primary spicules formed during the initial phase of spine regeneration. The scale bar at the bottom of Figure A is 20 µm while the one below Figure B is 10 µm (Vinnikovaa et al., 2011). The sea urchin belongs to the Scaphechinus mirabilis species.
In addition to the vesicles transporting all the elemental materials, around four dozen Strongylocentrotus purpuratus spicule matrix proteins, or SpSM proteins, participate in helping place and connecting the new calcium carbonates to the old spine (Gaurav et al., 2022). However, there are many hypotheses on what their precise roles could be. Genomics and proteomics have revealed that all SpSM proteins have a C-type lectin-like domain (Gaurav et al., 2022). All those proteins are responsible for binding materials together, most notably the new ACC to the old spine.
Shortly after the initial stage of spine repair, the larval spicule solidifies its base as it branches out hydrated ACC outwards, thanks to the vesicles continuously providing materials (Figure 16). However, the previously built sections solidify partially because the water has been extracted from the crystals. To do so, SpSM30 proteins crosslink to form specialized protein hydrogels that remove water molecules from the hydrated ACC as they dig their way into the structure. This results in the porous microstructure with bubble-like holes that one would see from up close since they essentially add pockets of air inside the growing spine (Figure 17).

Fig. 16 Cartoon representations of each type of SpSM protein at their most stable conformation. A circle means the protein folds on itself, while the curved lines are just disordered sections (Pendola et al., 2019).

Fig. 17 The above images were taken with a scanning electron microscope (SEM). Intermediate stage of the spine regeneration belonging to the Scaphechinus mirabilis. The tip of the repaired spine always contains more hydrated ACC than the old base that has already stabilized. After the larval spicule forms a circular base, it creates several branches in a circle that grow upwards, thus creating the cylinder that will eventually become the spine. The whole process, from spine fracture to total repair, takes about 40 to 45 days (Vinnikovaa et al., 2011). Scales: 20 µm (A, C, E, F, G, H); 10 µm (B); 100 µm (D, I).
Finally, researchers have found that SpSM50 is the main protein responsible for organizing and assembling calcium calcite, as a lack of it dramatically diminishes spine growth (Gaurav et al., 2018). Indeed, in an experiment of three growing spicule larvae, one was subjected to an extra dose of SpSM50, one to SpSM30, and the last one to nothing. In the end, the sample that received the dose of SpSM50 yielded the densest spine, with more than twice as much calcium carbonate as the other two (Figure 18). Based on this result, scientists determined that the main protein responsible for ACC transportation and assembly was SpSM50. It is also interesting to note that the sample that received the SpSM30 dose had the least mineral particles, even less than the control sample (Figure 18). This further shows that SpSM30 is responsible for clearing out the spine and causing the structure to increase in porosity. When tested in vitro in a hydrogel matrix, the porosity increased with the SpSM30 protein as more hydrogel proteins added air bubbles in the spine. In addition, this experiment also displays why a balance of both SpSM50 and SpSM30 is necessary. After all, an excess of either protein may render the spine too dense (SpSM50) or too hollow (SpSM30).
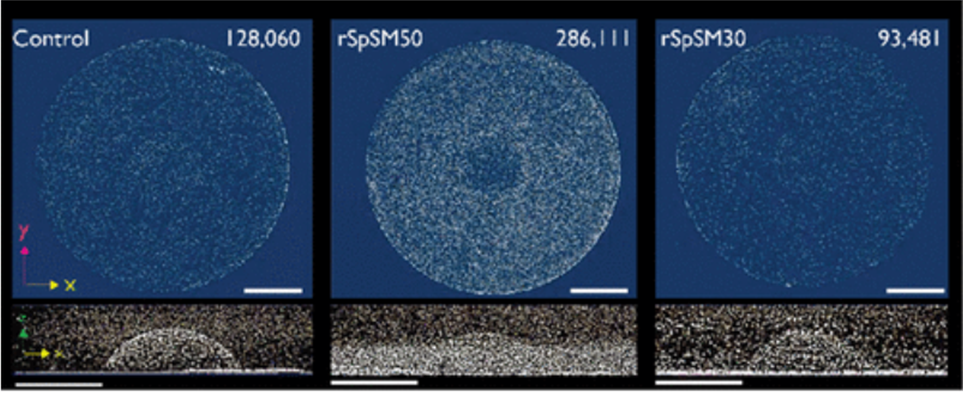
Fig. 18 µCT images of three growing spines with a top and side view. All spines grew the same amount of height. The scale bar for all images measures 1 mm. The number on the top right represents the number of mineral particles detected. Notice from the side-view images on the second row how the spines all have an extra dense base, as shown by the thick white line at the bottom of all three images. It is evident in the right-most picture on the second row (Gaurav J. et al., 2018).
Aside: Vaterite is a mineral, and is a polymorph of calcium carbonate. Calcite, aragonite, and amorphous calcium carbonate are also polymorphs of calcium carbonate (Calvert, 2003).
In essence, the main proteins that scientists could track and understand were the SpSM30 and SpSM50. Although their functions –clearing out the spine and assembling ACC – are straightforward, the impact that they have on the final structure is drastic. If one were to deposit vaterite crystals into a solution of a mix of those two proteins with water, it would be very easy to notice the differences compared to the same crystals submerged under plain water (Figure 19). As such, it is obvious why abiotically formed crystals can have a different shape, texture, or even strength compared to biotic minerals enhanced by highly complex proteins.
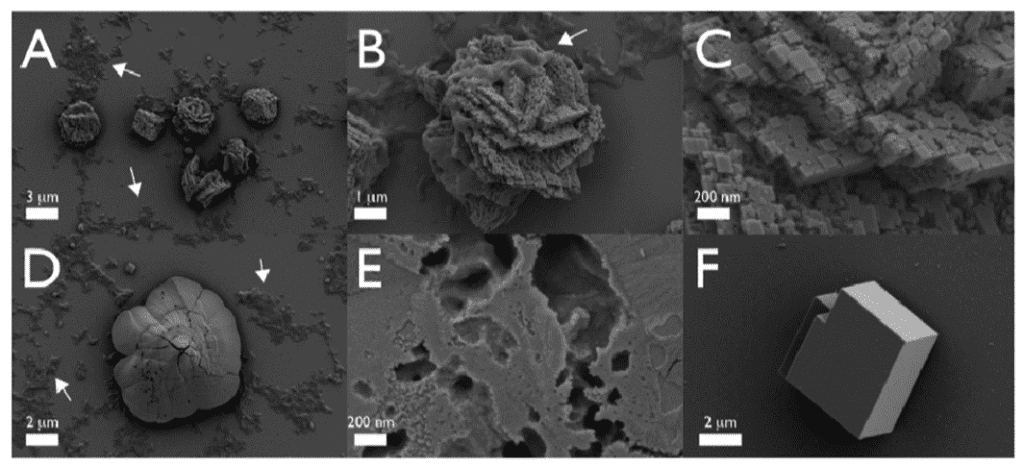
Fig. 19 The images above were taken with an SEM. All figures are vaterite crystals, though Figures A to E are ones submerged in an SpSM50 and SpSM30 solution, while Figure F is one in normal water. Note how in Figures A to E, one can find particular structures and rough textures, as well as porous voids on the surface of all minerals. On the other hand, Figure F displays a smooth, unchanged, vaterite crystal. Thus it retained its block-like structure (Gaurav et al., 2022).
Conclusion
Overall, spine types have different essential proteins. It is important to note that these all perform remarkably similar functions and illustrate that even with highly different environmental pressures, the basic ideas in design are illustrated through the similarities of these spines. Porcupine and hedgehog spines are all made of alpha keratin, a protein that exhibits a complex hierarchical structure. Keratin has a direct impact on the properties of the quills. It is a lightweight and resistant material which makes it interesting for engineers. In a manner similar to puffer fish spines, it provides excellent resistance while also having good flexibility. Porcupines and puffer fish contain collagen and hydroxyapatite. The alignment of mineralized collagen in the spine structure maximizes flexibility and strength. Hydroxyapatite has been recently utilized in biomedical applications such as bone regeneration. As for the sea urchin, it is clear it takes advantage of the mineral-saturated seawater to build its spine. After all, the spine’s components are all common elements found underwater, most notably calcium carbonate, a compound part of the ocean’s carbon cycle. However, what differentiates the crystal calcite spine of the sea urchin from a common rock made of the same materials is that biological proteins have enhanced it. Indeed, the sea urchin’s remarkable ability to quickly rebuild spines – far superior to abiotic counterparts – is entirely due to the product of their genome. Chemistry creates the basis of the strength of all spines. By harnessing and engineering better chemical properties, and using nature as inspiration, better biomaterials, load resistance structures, and countless other applications can be designed.
References
Aluigi, A., Zoccola, M., Vineis, C., Tonin, C., Ferrero, F., & Canetti, M. (2007). Study on the structure and properties of wool keratin regenerated from formic acid. International Journal of Biological Macromolecules, 41(3), 266-273. https://doi.org/https://doi.org/10.1016/j.ijbiomac.2007.03.002
Ameyea L.,Beckerb D. G., Killianc C., Wiltc F.,Kempsd R., Kuypersd S., Duboisa P. (2001). Proteins and Saccharides of the Sea Urchin Organic Matrix of Mineralization: Characterization and Localization in the Spine Skeleton. Science Direct. 134. 1. https://doi.org/10.1006/jsbi.2001.4361
Bone, Q. (2003). FISH | Introduction. Encyclopedia of Food Sciences and Nutrition (Second Edition), 2404-2408. https://doi.org/10.1016/B0-12-227055-X/00465-X
Chou, S. F., Overfelt, R. A., & Miller, M. E. (2012). Anisotropic mechanical behavior of keratin tissue from quill shells of North American porcupine (Erethizon dorsatum). Materials Science & Engineering. A, Structural Materials: Properties, Microstructure and Processing, 557, 36–44. https://doi.org/10.1016/j.msea.2012.05.111
Calonje, J. E., Brenn, T., Lazar, A. J., & Billings, S. D. (2018). McKee’s pathology of the skin, 2 volume set E-book (5th ed.). Elsevier. https://www.elsevier.com/books/mckees-pathology-of-the-skin/calonje/978-0-7020-6983-3
Calvert, P. D. (2003). Biomineralization and Biomimetic Materials. Encyclopedia of Physical Science and Technology (Third Edition), 193-205. https://doi.org/10.1016/B0-12-227410-5/00063-6
Casillas-Vargas, G., Ocasio-Malavé, C., Medina, S., Morales-Guzmán, C., Del Valle, R. G., Carballeira, N. M., & Sanabria-Ríos, D. J. (2021). Antibacterial fatty acids: An update of possible mechanisms of action and implications in the development of the next-generation of antibacterial agents. Progress in Lipid Research, 82, 101093. https://doi.org/https://doi.org/10.1016/j.plipres.2021.101093
Currey, J. D. (2006). Bones : structure and mechanics ([2nd ed.]). Princeton University Press.
Drozdov, A.L., Sharmankina, V.V., Zemnukhova, L.A. (2016). Chemical composition of spines and tests of sea urchins. Biol Bull Russ Acad Sci 43, 521–531. https://doi.org/10.1134/S1062359016060078.
Elliott J. C., Mackie, P. E., & Young, R. A. (1973). Monoclinic hydroxyapatite. Science (New York, N.Y.), 180(4090), 1055–1057. https://doi.org/10.1126/science.180.4090.1055
Garcia-Granda, S., & Montejo-Bernardo, J. (2005). X-RAY ABSORPTION AND DIFFRACTION | X-Ray Diffraction – Single Crystal. Encyclopedia of Analytical Science (Second Edition), 397-408. https://doi.org/10.1016/B0-12-369397-7/00672-5
Gaurav J., Martin P., Yu-Chieh H., Denis G., John S. E.. (2018). Secrets of the Sea Urchin Spicule Revealed: Protein Cooperativity Is Responsible for ACC Transformation, Intracrystalline Incorporation, and Guided Mineral Particle Assembly in Biocomposite Material Formation. ACS Publications. 3. 9. pp. 11823-11830. https://doi.org/10.1021/acsomega.8b01697
Gaurav J., Martin P., Yu-Chieh H., Denis G., John S. E.. (2022). A Model Sea Urchin Spicule Matrix Protein, rSpSM50, Is a Hydrogelator That Modifies and Organizes the Mineralization Process. ACS Publications. 10. https://dx.doi.org/10.1021/acs.biochem.7b00083
Giordano M., Norici A., Ratti S., Raven A. J.. (2008). Role of Sulfur for Algae: Acquisition, Metabolism, Ecology and Evolution. Research Gate.
http://dx.doi.org/10.1007/978-1-4020-6863-8_20
Hertwig, I., Eichelberg, H., & Hentschel, J. (1992). Light and electron microscopic studies of the skin of the Palembang puffer,Tetraodon steindachneri (Teleostei, Tetraodontidae). Zoomorphology, 111(4), 193–205. https://doi.org/10.1007/bf01633008
Ikoma, T., Kobayashi, H., Tanaka, J., Walsh, D., & Mann, S. (2003). Physical properties of type I collagen extracted from fish scales of Pagrus major and Oreochromis niloticas. International Journal of Biological Macromolecules, 32(3-5), 199-204. https://doi.org/10.1016/S0141-8130(03)00054-0
Jäger, I., & Fratzl, P. (2000). Mineralized collagen fibrils: A mechanical model with a staggered arrangement of mineral particles. Biophysical Journal, 79(4), 1737–1746. https://doi.org/10.1016/s0006-3495(00)76426-5
Lazarus, B. S., Chadha, C., Velasco-Hogan, A., Barbosa, J., Jasiuk, I., & Meyers, M. A. (2021). Engineering with keratin: A functional material and a source of bioinspiration. iScience, 24(8), 102798. https://doi.org/10.1016/j.isci.2021.102798
McKittrick, J., Chen, PY., Bodde, S.G.(2012). The Structure, Functions, and Mechanical Properties of Keratin. JOM 64, 449–468. https://doi.org/10.1007/s11837-012-0302-8
Nurul, I., Farida, W., & Purwaningsih, E. (2020). Microstructure of Quills in Sunda Porcupine Hystrix javanica (F. Cuvier, 1823) [Mikrostruktur Duri Landak Jawa Hystrix javanica. 16, 81-88. https://doi.org/10.47349/jbi/16012020/81
Pendola M., Gaurav J., Evans J. S. (2019). Skeletal development in the sea urchin relies upon protein families that contain intrinsic disorder, aggregation-prone, and conserved globular interactive domains. Plos One.
https://doi.org/10.1371/journal.pone.0222068
Peppino. (2021, June 11). Diodon hystrix. Monaco Nature Encyclopedia. https://www.monaconatureencyclopedia.com/diodon-hystrix/?lang=en
Politi, Y., Metzler, R. A., Abrecht, M., Gilbert, B., Wilt, F. H., Sagi, I., Addadi, L., Weiner, S., & Gilbert, P. U. (2008). Transformation mechanism of amorphous calcium carbonate into calcite in the sea urchin larval spicule. Proceedings of the National Academy of Sciences of the United States of America, 105(45), 17362–17366. https://doi.org/10.1073/pnas.0806604105
Roze, U., Locke, D. C., & Vatakis, N. (1990). Antibiotic properties of porcupine quills. Journal of Chemical Ecology, 16(3), 725-734. https://doi.org/10.1007/BF01016483
Sikorski, Z. E., Scott, D. N., & Buisson, D. H. (1984). The role of collagen in the quality and processing of fish. Critical Reviews in Food Science and Nutrition, 20(4), 301–343. https://doi.org/10.1080/10408398409527393
Su, F. Y., Bushong, E. A., Deerinck, T. J., Seo, K., Herrera, S., Graeve, O. A., Kisailus, D., Lubarda, V. A., & McKittrick, J. (2017). Spines of the porcupine fish: Structure, composition, and mechanical properties. Journal of the Mechanical Behavior of Biomedical Materials, 73, 38-49. https://doi.org/10.1016/j.jmbbm.2017.02.029
Torres, F. G., Troncoso, O. P., Diaz, J., & Arce, D. (2014). Failure analysis of porcupine quills under axial compression reveals their mechanical response during buckling. Journal of the Mechanical Behavior of Biomedical Materials, 39, 111–118. https://doi.org/10.1016/j.jmbbm.2014.07.017
Varkoulis, A., Voulgaris, K., Zaoutsos, S., Stratakis, A., & Vafidis, D. (2020). Chemical composition and microstructural morphology of spines and tests of three common sea urchins species of the sublittoral zone of the Mediterranean Sea. Animals: An Open Access Journal from MDPI, 10(8), 1351. https://doi.org/10.3390/ani10081351
Vinnikovaa V., Drozdov A. (2011). Spine Skeleton Morphogenesis During Regeneration in Clypeasteroid and Camarodont Sea Urchins. Russian Journal of Marine Biology. 37. 4. pp. 311-318. https://doi.org/10.1134/S1063074011040122
Politi, Y., Metzler, R. A., Abrecht, M., Gilbert, B., Wilt, F. H., Sagi, I., Addadi, L., Weiner, S., & Gilbert, P. U. P. A. (2008). Transformation mechanism of amorphous calcium carbonate into calcite in the sea urchin larval spicule. Proceedings of the National Academy of Sciences of the United States of America, 105(45), 17362–17366. https://doi.org/10.1073/pnas.0806604105
Yang, W., & McKittrick, J. (2013). Separating the influence of the cortex and foam on the mechanical properties of porcupine quills. Acta Biomaterialia, 9(11), 9065–9074. https://doi.org/10.1016/j.actbio.2013.07.004
Wang, B., Yang, W., McKittrick, J., Meyers, M-A. (2016). Keratin: Structure, mechanical properties, occurrence in biological organisms, and efforts at bioinspiration. Progress in Materials Science,Volume 76, pp. 229-318, ISSN 0079-6425, https://doi.org/10.1016/j.pmatsci.2015.06.001.