Abstract
Hooves are morphologically complex structures among ungulates that support body weight and provide traction to assist their adaptation to various external environments. This article aims to investigate the differences in hooves throughout the stages of life. The perspective narrows from the general topic of hooves down to the fascinating molecule, which makes up approximately 90 percent of the hoof—keratin. The structure of keratin and its unique chemical interactions are the reasons for its rigidity and ability to support the animal. For the fetal ungulate, the hooves have not comprehensively developed. The hoof’s structures are gradually formed through cell differentiation in the womb, and the rate of hoof length is highest right after birth. In mature ungulates, the thickness of the hoof wall is an essential indicator in determining whether the ungulate can survive in the wild. Keratinization is the process of keratin production, which thickens the hoof wall. Throughout the keratinization process, transcription errors may lead to the formation of tumorous cells, and hoof diseases, such as keratomas. Since the hooves are constantly abrased, hoof fractures may occur among ungulates. After ungulates die, analysis of the ungulate’s hoof can reveal information about their diet, lifestyle, and living conditions before death. In all, this article concentrates on the microscopic analysis of the hoof to explore the life of ungulates.
Introduction: The Microanatomy of the Hoof
Keratins are a group of filament-forming and water insoluble proteins produced in specific epithelial cells of vertebrates. They are a subset of the superfamily of intermediate filament proteins. Horny layers of epidermal appendages, including nails, hair, horns, feathers, and hooves are mostly composed of keratin. Keratinous materials have a high content of the amino acid cysteine and are characteristically tough, durable, and unreactive to the natural environment. If keratin is the chemical makeup for an anatomical feature, it likely functions as mechanical support or protects a structure from the external environment (Wang et al., 2016).
The hoof is composed of the hoof wall, the frog, the sole, and the digital cushion. These structures can be seen in Figure 1 below. The wall is visible when the animal stands, and the frog and the sole are visible when the foot is lifted off the ground. The digital cushion is a shock-absorbing tissue and is inside the hoof.
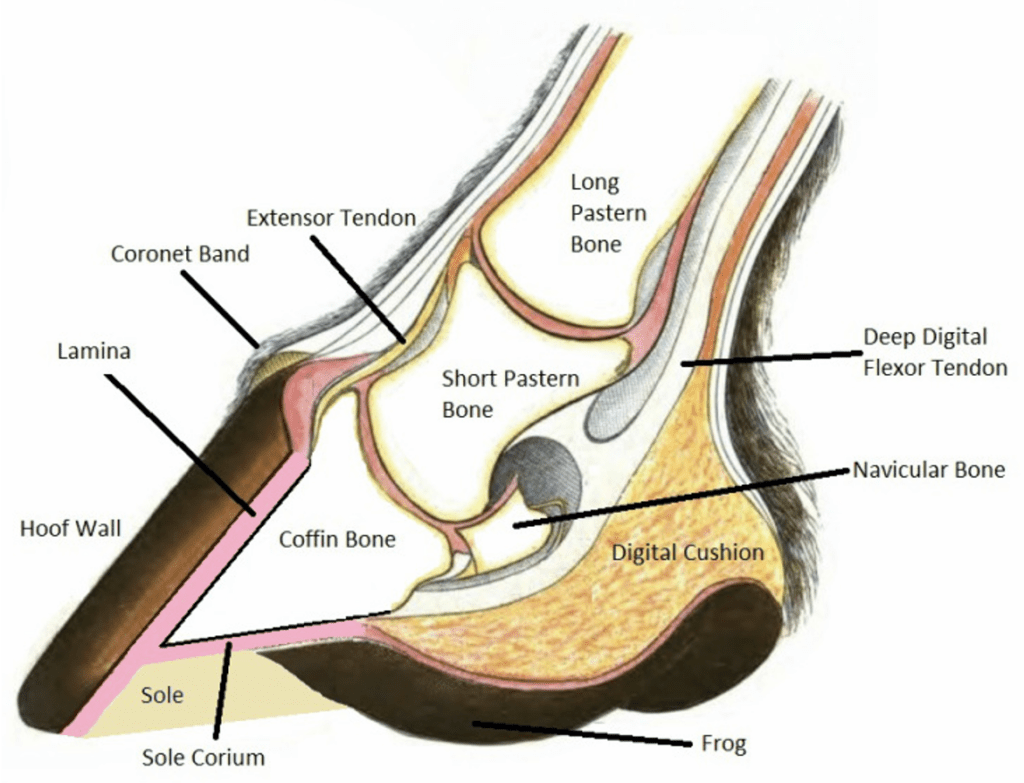
Fig. 1 Labeled, cross-sectional diagram of a horse hoof (Alderman, 2017).
In artiodactyl, the keratinous hoof wall is growing continually. A magnified illustration of the hoof wall can be seen in Figure 2. The hoof is worn down by natural use or is maintained by humans if the animal lives in captivity. Nerves and blood vessels are absent in the hoof wall (McLure, 1999).
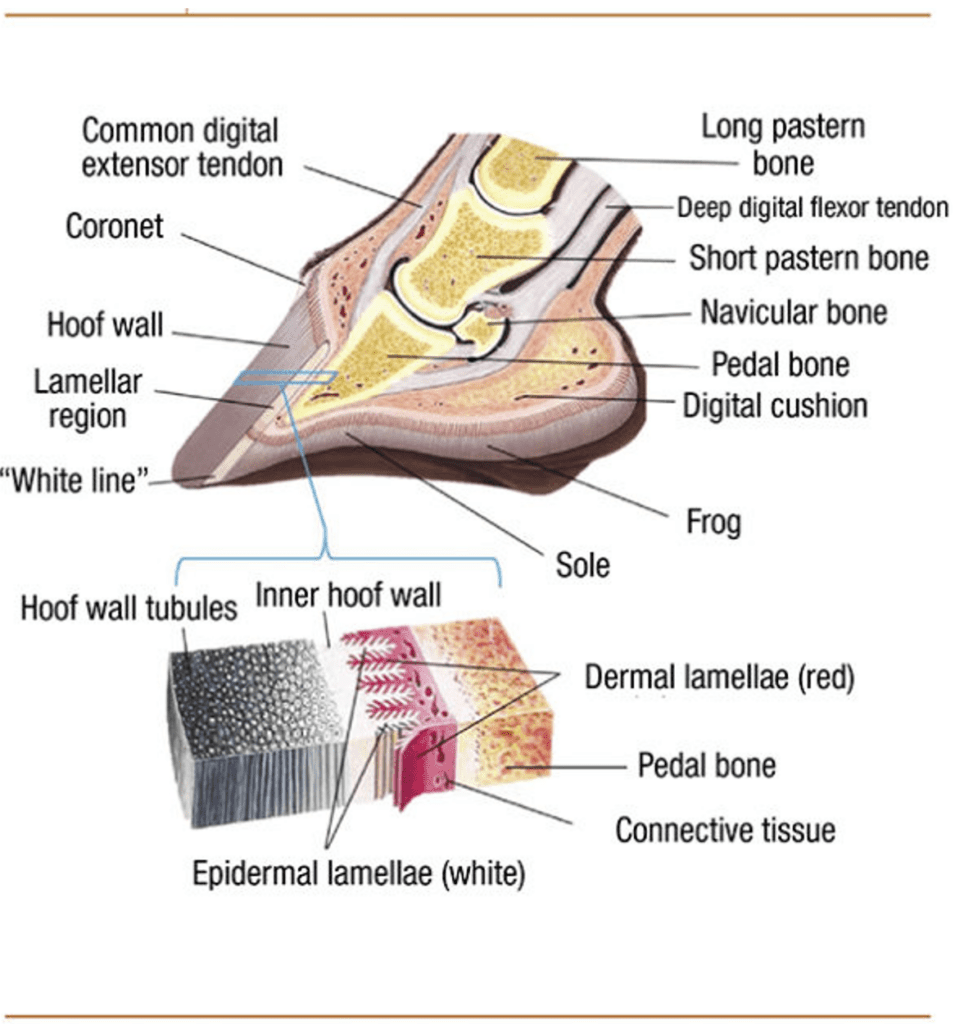
Fig. 2 A cross section of a cow hoof and the magnification of a segment near the hoof wall, where keratin is formed (Pecora, 2022).
In particular, the hoof wall is subjected to high magnitude ground-reaction forces in multiple directions and transfers these forces to the skeleton. Damage to the hoof wall remains until it is worn off, so the wall must be able to withstand high stresses frequently. In fact, Kasapi et al. (1997) found that the central epidermal layer (stratum medium) of the hoof wall is one of the most fracture-resistant biological materials known. To understand the chemical basis of this biomaterial, the molecular composition of the hoof wall will be analyzed.
The Hoof Wall and Keratin
Keratin proteins are classified based on their secondary structures: they can either be alpha, composed of alpha helices; or beta, composed of beta-pleated sheets. Alpha keratin is most commonly found in mammals, while beta keratin is typically present in hard reptilian and avian tissues. Furthermore, there are different classifications of keratins beyond their secondary structure used in literature, such as the amount of sulfur cross links, modes of biosynthesis and hard/soft keratins. The keratin comprising hooves, for example, is classified as hard as these keratin proteins have a higher amount of sulfur crosslinks—disulfide linkages between cysteine thiols—and a more consolidated structure than soft keratin (Fass & Thorpe, 2017). Some other examples of hard keratin are nails, hair, and claws and one example of soft keratin is the stratum corneum (outermost layer of skin) (Wang et al., 2016).
There are many similarities between alpha and beta keratinous materials at the nanoscale. For example, both have a fine filament-matrix structure composed of tightly bound polypeptide chains (Figure 3).
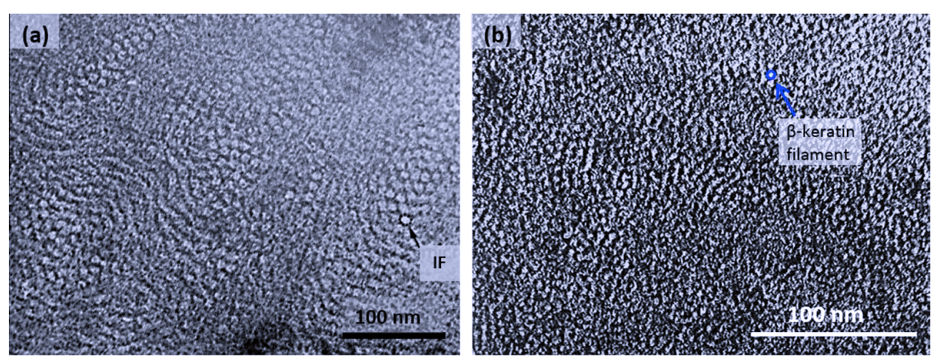
Fig. 3 Transmission electron micrograph of matrix-filament structure. Note that (a) depicts alpha keratin and (b) depicts beta keratin (Wang et al., 2016). IF stands for intermediate filament, an illustration can be seen in Figure 5.
Differences between alpha and beta keratins arise from the molecular structure and formation of the filaments. This paper will only examine alpha keratins, since hooves do not contain beta keratins. Hydrogen bonding stabilizes the coiled formation of an individual keratin molecule, as depicted in Figure 4 below.
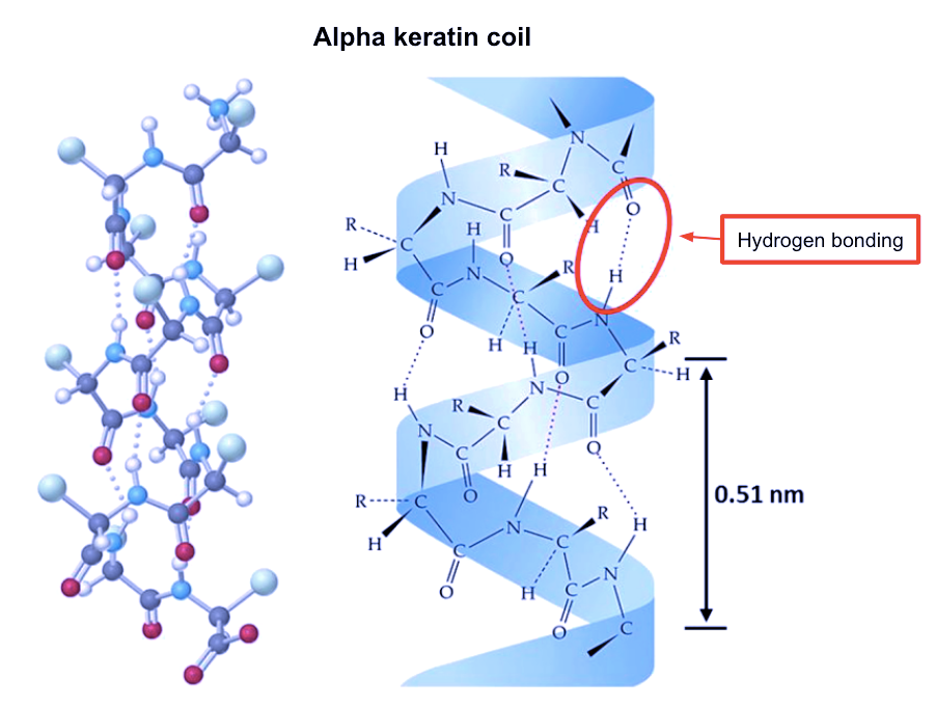
Fig. 4 An alpha keratin coil (Adapted from Wang et al., 2016).
Alpha keratins are then formed into coiled structures, containing two chains of polypeptides wound into helices. Disulfide cross-linking bonds two isolated alpha-helix chains and forms a dimer. The subsequent steps of alpha keratinous tissue formation are shown in Figure 5 below.
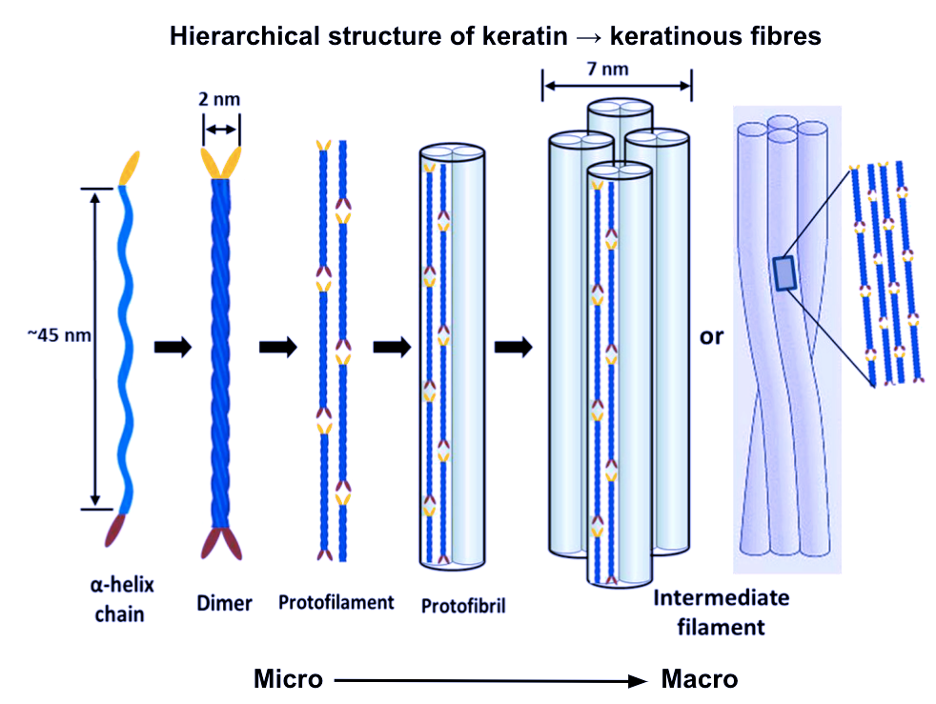
Fig. 5 The hierarchical structure of keratin to keratinous fibers (Adapted from Wang et al., 2016).
Hooves are considered multi-level hierarchical composites. They are composed of flattened keratinized cells organized into tubules with diameters between 200 and 300 µm that have hollow cavities. Relative to the long axes of the tubules, intratubular materials lie at large angles, creating a composite in the macroscale, fiber-reinforced at the nanoscale (Figure 6) (Wang et al., 2016).

Fig. 6 Illustration of the hoof wall, including magnification (Adapted from Wang et al., 2016).
The non-uniform structure of the hoof material impedes crack growth. Varying tubule and intertubular organizations, in addition to the formation of different volumes of the filament-matrix structure in different orientations, allows the substructures to absorb a high amount of energy as fractures grow (Figure 7). For a crack to propagate, the fracture must separate bonds at both the tubular and intertubular component levels, at cell boundaries in the hoof wall, and in different orientations of the filament-matrix (Wang et al., 2016).
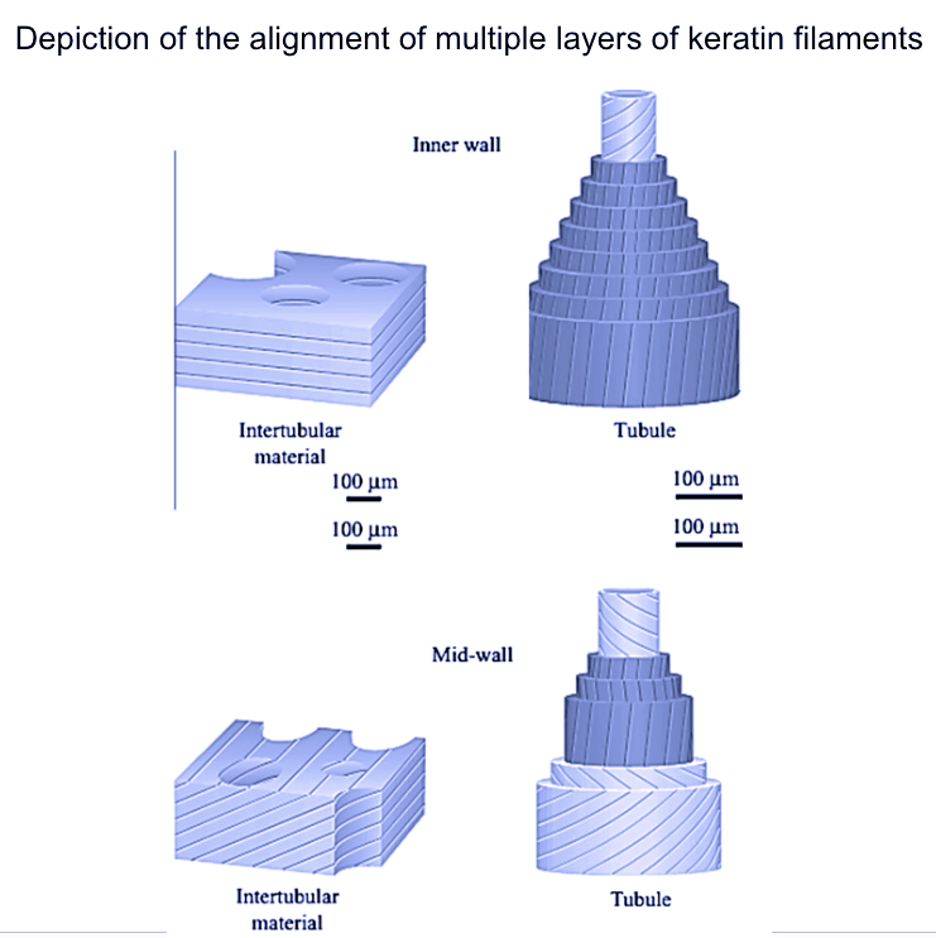
Fig. 7 Illustration of different volumes of the filament-matrix structure in different orientations (Adapted from Wang et al., 2016).
Scientists have created notches in hooves at different orientations and locations to elucidate crack diversion mechanisms. These mechanisms redirect cracks in the dead keratin so that they do not reach and damage live tissue. This crack redirection is related to the reason why they are prevented: the diversity of materials making up the keratinous tissue, including tubules and different layers of filaments. Furthermore, stress waves propagating through the hooves may damage living bone tissue when animals run. There are two proposals for the mechanism of damping. First, the scattering of waves by internal interfaces and tubules, and second, a decay of the wave caused by viscoelastic response of the keratin (Wang et al., 2016).
Solubility is important to consider for keratinous tissues, as hooves must remain strong in wet conditions. Keratins are insoluble because of interchain peptide linkages and intramolecular disulfide linkages (Fraser et al., 1972). However, hydration levels impact the mechanical properties of hooves, making them more resistant to fracturing.
Specifically, increasing hydration levels decrease the Young’s modulus of hooves and increases their strain. Bertram and Gosline (1987) predict that hydration changes more strongly affect the properties of the matrix phase than the microfibrillar phase because the matrix has few covalent crosslinks, so its stabilization may depend on secondary cross-linking mechanisms, which may be hydration dependent: hydrogen bonding, for example. The microfibrillar phase is unaffected by water because it is stabilized by disulfide crosslinks. Relative humidity—another term for hydration level—follows one gradient from top to bottom and one gradient from inside to out from high to low hydration level. So, referring to Figure 1, increasing distance from the pedal bone decreases moisture in the hoof keratin (Wang et al., 2016).
Fetus
Physiologically, the neonate mammal is different from the juvenile. During intrauterine maturation, the fetus’ systems, such as its respiratory, pulmonary, and cardiovascular systems, are well adapted to this hypoxic environment. Upon birth, an orchestrated and rapid adaptation to the extra-uterine conditions is executed to ensure neonatal survival (Morton, 2016). Similarly, the hooves of ungulates must be functional at birth; that is, infant hooves must be able to rapidly assume weight-bearing conditions to prevent lameness or infant mortality due to predators (Bidwell, 2006). This process involves biochemical changes and can be understood by examining the changes between the micro-architecture of the keratinized hoof of the fetus and of the newborn ungulate.
The development of the fetal hoof begins early in gestation. Mayor (2019) studied the fetal development of the red brocket deer (Mazama americana) and plotted the appearance of external morphological features with respect to the total dorsal length, TDL, in the graph shown in Figure 8. Hooves were found in embryos with TDL around 10 cm, corresponding to approximately 75 days of gestation and 36% of total gestation time (Mayor, 2019). In fact, in the red brocket deer, hooves begin to develop even before the condensation of the skin.

Fig. 8 Probability curves for external morphological features along the increase in total dorsal length (TDL) in 39 embryo/fetuses of red brocket deer (Mazama americana) (Mayor, 2019).
In the hoof, there are dozens of different types of keratin molecules, all with varying stiffnesses, molecular weights, and sulfur concentrations (Pollitt, 2004). Furthermore, hoof production is continuous: once initiated in utero, it does not stop until death. The hoof develops around the highly porous distal phalanx—also called the coffin bone or P3—which helps to maintain the mineral homeostasis of the hoof by reserving calcium, phosphorus and growth factors (Al-Agele, 2018). The first stage in fetal hoof development involves the layering of epidermal cells around the P3. Franciolli et al. (2011) discovered that hoof formation is typically initiated in equine embryos by day 27 of 340. By day 60, there are measurable variations in the thickness of the epidermis at the tips of limbs. Specifically, the hoof wall, with two to three layers of cuboidal cells, is thicker than the sole, with only one cuboidal layer (Rabelo, 2015) (Fig. 9). At this point, the parietal dermis and parts of the perioplic and coronary dermis are forming (Fig. 10).
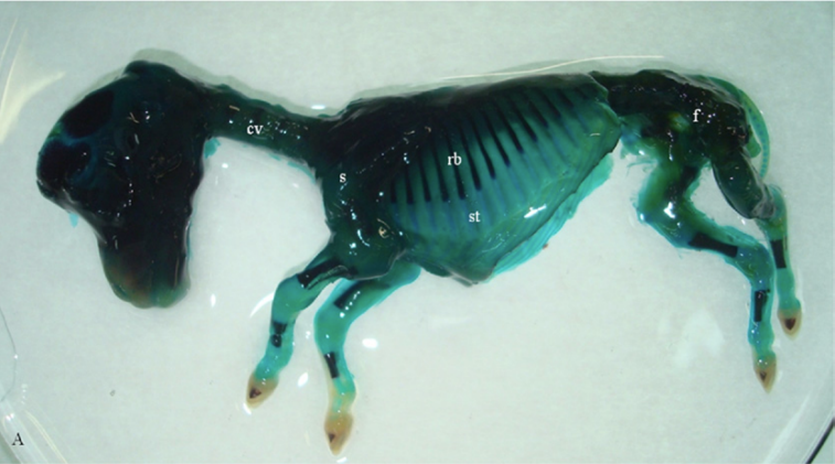
Fig. 9 A day 60 equine fetus subjected to the modified Alizarin technique. Cartilage is light blue, whereas areas of ossification are dark purple. cv, cervical vertebrae; f, femur; rb, ribs; st, sternum (Mayor, 2019).

Fig. 10 55-day-old fetus. Scanning electron micrograph of the hoof. Hoof wall [PaD = parietal dermis] and regions of perioplic [PD] and coronary dermis [CD] (Nunes Barreta et al., 2016).
Upon this newly formed dermis, increasing mitotic activity gives rise to the first finger-like laminar bodies of the hoof. Primary and secondary epidermal and dermal laminae project outwards from the epidermis and the dermis, respectively, to form an interconnected suspensory apparatus (Fig. 11). Laminae are made of collagen, a protein with great tensile strength. Eventually, the weight of the ungulate, which is passed down through the distal phalanx, will be passed into this matrix (Rabelo, 2015).
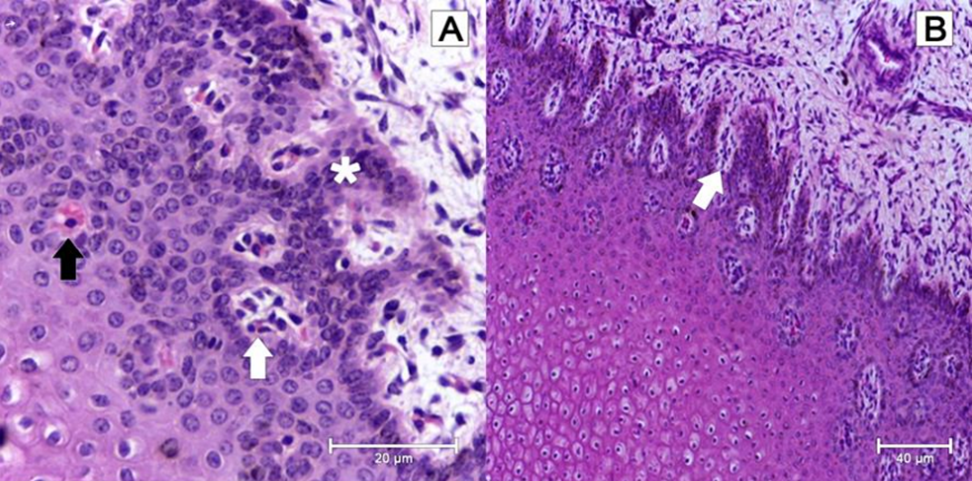
Fig. 11 In A, microscopic structure of 70-day bovine fetus’ epidermis. Notice the distribution with a tendency to agglomeration of keratinocytes (white arrow), the discreet formation of keratin (dark arrow) and the beginning of the formation of the epidermal papillae along the layers of spinous cells (asterisk). In B, the microscopic structure of a 70-day bovine fetus’s epidermis. Notice the formation and initial differentiation of the epidermal papillae (white arrow) (Rabelo, 2015).
Rigidity and strength are two important structural characteristics of the hoof. Rigidity describes the inability of the hoof to be easily bent out of shape, and strength describes the ability of the hoof to withstand large forces from many directions. These properties are afforded to the hoof through the cementation of keratin fibers. This is accomplished by the secretion of the membrane coating material (MCM), a bio-active extracellular matrix. Given that MCM is formed from the cell membranes of adjacent cells, which contain proteins and glycoproteins, such molecules are also present in MCM. A few major glycoproteins in extracellular matrices are fibronectin, tenascin, and laminin. Laminin is important for MCM found in hooves because this glycoprotein is released by epithelial cells into the basal lamina, a sheet of MCM separating epithelial cells from connective tissue (Halper & Kjaer, 2014). Mutations in genes expressing the proteins that form MCM may jeopardize the pathological integrity of the neonate hoof. For example, Yang et al. (2019) stated that alterations of MCM in the hoof are known to be the root cause of the “pathogenesis of infectious diseases of the hoof.” As such, a reliable in vitro reproduction of MCM can offer a biotechnological method to study chronic inflammation and disease.
Upon birth of the ungulate, various microscopic and chemical changes occur inside the hoof. First, juvenile ungulates have a thicker epidermal layer of the hoof wall than fetal ungulates. This thickening is partially due to changes in the long, thin cylindrical structures found in horn of hooves (horn tubules). Horn tubules only appear in the final stages of fetal life, when they are discreet, randomly distributed, and oriented perpendicular to the epidermal papillae (Rabelo, 2015). At birth, approximately 1300 of these tubules are present (Harrison et al., 2007). As the juvenile ages, the diameter of the horn tubules, the gap between the horn tubules and the number of horn tubules increases (Conrad, 2017). Combined, these three changes thicken the epidermal layer of the hoof. Second, there are changes to the stratum internum as the infant ungulate ages. In fetuses, the stratum internum of the inner hoof wall is equally thick around the toe and the sides of the hoof. Upon birth, the primary epidermal laminae begin to branch, increasing the density and the overall length of the stratum internum (Figure 12), and subsequently increasing the weight-bearing capabilities of the hoof.
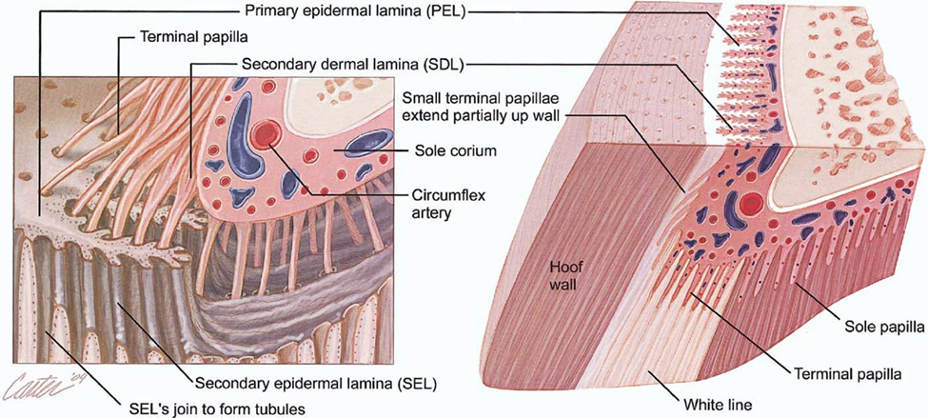
Fig. 12 Anatomy of the equine hoof (Gerard, 2021).
Hoof growth is controlled by the rate of production of keratinocytes in the coronary epidermis (Gerard, 2021). Furthermore, the rate of growth of the infant hoof is different from the rate of juvenile hoof growth, which is different from mature hoof growth. Upon birth, a ridged line separating the prenatal and postnatal growth of the hoof – the foal hoof crease – appears near the hairline and grows towards the tip of the hoof (Flueck & Smith-Flueck, 2005). The time for this line to grow out takes 145 ± 15 days (Curtis et al.). On the other hand, the hoof renewal rate for weanlings is 283 ± 29 days (Curtis et al.), and for mature horses, it is 337 days (Josseck, 1991). In other terms, the hoof grows 0.43 mm per day in foals, 0.28 mm per day in weanlings, and 0.20 mm per day in mature horses. This increased hoof growth speed in foals can be attributed to the increased production of keratinocytes in the epidermis. This feature of foal hoof growth allows the overall size of the hoof to increase rapidly, consequently, the distal phalanx to expand (Conrad, 2017). Therefore, the age of the newborn ungulate can be reliably predicted from the length of new-hoof growth (Grovenburg et al., 2014).
The hoof is both a semi-permanent structure that has been cemented by MCM and a constantly renewing apparatus that can quickly react to new environmental conditions. Understanding these mechanisms and reactions lends valuable insight into the possibilities of biotechnology and the development of methods to study inflammation and pathological diseases.
Healthy Individual
As the ungulate ages, the length of hooves and thickness of the hoof wall are continuously increasing to adapt to external environmental changes (Faramarzi et al., 2009). Horses are considered mature when their bones are fully developed after the age of four (Hanousek et al., 2020). As well, the thickness of the hoof wall continuously increases since it provides significant support for the ungulate’s body weight achieved by keratinization. The hoof wall of a healthy adult equine grows at a rate of approximately 0.6-1.0 centimeter per month (Curtis, 2017). The rigidity of hooves is due to the sulfated protein structure, keratin, which provides the structural basis for the unique properties and protection against a wide range of environmental factors (Hendry et al., 2001). Additionally, the hardness of the hoof wall serves as a vital indicator of hoof health.
The hoof wall is composed of three distinct layers of stratum corneum (Douglas & Thomason, 2000). The stratum externum and stratum medium, are the two outer layers which are generated by and grow downwards from the epidermis of the coronary band (Figure 13).

Fig. 13 The cross-section of a neonatal horse’s hoof wall. Stratum internum, stratum medium, and stratum externum are illustrated from left to right (Jennings, 2017).
The stratum externum is the outermost and thinnest layer of the hoof wall. The stratum medium is the middle and thickest layer. Based on the current analysis, it is evident that the hardness of the medium stratum layer directly correlates to the hoof’s health (Bragulla et al., 2010; Luccia et al., 2010). The innermost layer of the hoof wall is the stratum internum, produced by the epithelium of the laminar epidermis and fused to the stratum medium, forming a relatively contiguous hoof wall as shown in Figure 14 (Jennings, 2017).
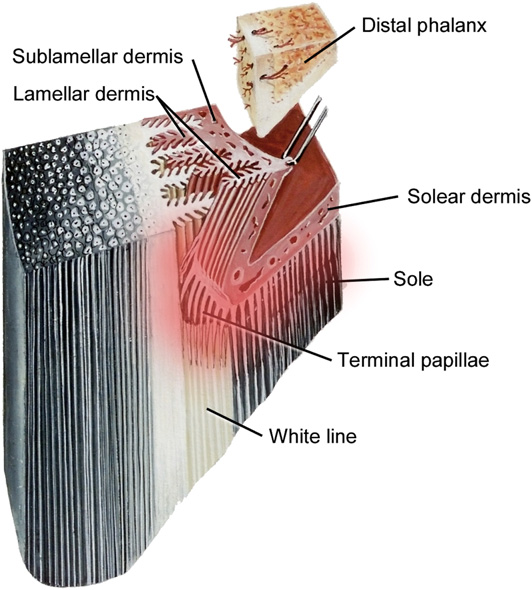
Fig. 14 The anatomy of the hoof wall which shows the exact location of dermal lamellae and epidermal lamellae (Pollitt, 2017).
Cytokeratin (CK) is a protein found on living epithelial cells which are in the red zone in Figure 14. Cytokeratin is also a marker used to detect epithelial tumor cells during keratinization (Wattle, 2000). CK serves to fuse the stratum internum and the stratum medium by generating a cytoskeleton through polymerization of intermediate filaments from cytokeratin (Wattle, 2000). The separation of stratum internum and stratum medium may cause fungal infection on the hoof wall that may lead to the serious white line disease and incur other health issues among equines (O’Grady et al., 2006). CK is distributed in the basal cells of the matrix of the stratum medium of the hoof wall and in the basal and suprabasal cells of the stratum internum (Wattle, 1998; Wattle, 2000). CK 4, 5/6, 10, 14 are four different indicators that can be found in distinct layers of hooves (Hendry et al., 2001). CK 5/6 and CK 14 were shown to be present in the basal epidermis of healthy tissue, whereas CK 10 was found in supra-basal layers in healthy tissue by immunohistochemistry (Hendry et al., 2001). The distribution of cytokeratin also corresponds to the diseases affecting the hooves, such as solar ulceration. For instance, at the site of solar ulcers, CK 5/6 and CK 14 were each found in both the basal and supra-basal epidermis (Jadotte & Schwartz, 2012). The healthy distribution of cytokeratin can help to detect the growth of ulceration since ulceration stimulates the repair of epidermal protein synthesis and keratinocyte proliferation.
The functional integrity of the hoof essentially depends on the keratinization of the hoof epidermal cells. Keratinization of the hoof epidermis is controlled and modulated by a variety of bioactive molecules and hormones (Hsu et al., 1991). This process is dependent on an appropriate supply of nutrients, including vitamins, minerals, and trace elements from the dermis layer. During the keratinization process, minerals such as calcium, phosphorus, sodium, zinc, and potassium play a prominent role in the formation of a healthy hoof wall (Fraser & MacRae. 1980).
Zinc (Zn) has the most ubiquitous distribution among cells; in addition to Zn being the most abundant intracellular trace element, it plays a prominent role in the basic functions of protein formation (Tomlinson et al., 2004). While Zn is a component involved in over 200 enzyme systems, it also has a role in 3 key functions in the keratinization process — catalytic, structural, and regulatory (Cousins, 1996). Catalytic roles are observed in enzymes such as RNA nucleotide transferases, RNA polymerase, alkaline phosphatase, alcohol dehydrogenase, and carbonic anhydrases (Cousins, 1996; Fox & Tylutki, 1998). Zn exists as an activator in these catalytic enzymes; thus, Zn is an essential component in the differentiation of keratinocytes. Zn also plays a key role in the formation of structural proteins during the keratinization process. Zn participates in the formation of zinc-finger protein, a common DNA binding domain found in many transcription factors (Ede et al., 2017). Zinc-finger proteins are involved in protein-to-protein interactions, most of which affect cellular differentiation and proliferation (Tomlinson et al., 2004; Cousins, 1996). Zn-finger proteins consist of an amino acid sequence: -C-X2-C-Xn-C-X2-C-, where C represents cysteine (Cys) and X represents other amino acids. The pentapeptide sequence Cys-Gln-Pro-(Ser, Thr)-Cys is identified in the α-helix chain of hard keratins; as discussed previously, the hard α-helix keratin is the main material of the hoof (Fraser & MacRae, 1980). Therefore, insufficient Zn may decrease the formation of Zn-finger proteins and consequently, the formation of keratin filaments needed in the development of keratinocytes would decrease as well. Also, Zn plays a key regulatory role during the formation of regulatory enzymes, such as calmodulin, protein kinase C, and inositol phosphate (Sherry et al., 2005). Calmodulin is responsible for binding calcium ions (Ca2+) and carrying them into the cytosol through ion channels (Walsh, 1983). Calmodulin is important in the final step of the developing keratinocyte since calcium activates epidermal transglutaminase, an enzyme that catalyzes the formation of protein-to-protein crosslink (Eckert et al., 2005; Sárdy et al., 2002). Besides zinc, other regulatory enzymes and hormones are also essential for keratinization. Protein kinase C is responsible for the phosphorylation of proteins, thus adding available energy to the differentiation process (Lim et al., 2015). Thyroid hormones regulate the action of calmodulin and protein kinase C. Inositol phosphate increases Ca2+ by mobilizing this ion from intracellular stores, primarily from the endoplasmic reticulum. Therefore, keratinization – a major process to generate the rigid hoof wall requires many elements. However, zinc cannot be naturally generated by ungulates; thus, a diet containing appropriate amounts of zinc is essential to maintain the health of ungulate hooves.
The shape and internal content of hooves can reflect the age and habitat of ungulates. Ungulates in moist habitats typically have longer hooves, while ungulates in stiff and dry habitats typically have shorter hooves (Florence & McDonnell, 2006). Also, the age of the animal is reflected in its hooves. Water content and magnesium concentration increase with age and potassium concentration is lower in the hooves of pregnant animals. As mentioned previously, the thickness of the hoof wall increases with age. Thus, the hoof wall of older ungulates contains more calcium, copper, zinc and phosphorus and lower concentrations of water, sodium, potassium, and iron than the hoof of younger ungulates.
Diseases of the Hoof
The hoof, like any other part of the animal’s body, may suffer from different diseases throughout its lifetime. Understanding the mechanism of such diseases and their treatments can provide insight into the role and limitations of the hoof.
Keratoma is a benign tumor of the epidermal cells that produce keratin, found on the inner layer of the hoof wall (Deidre et al., 2010). The tumor is a hyperplastic mass that grows slowly, eventually occupying significant space in the affected area (Miller et al., 2015). Keratomas are made of poor-quality horn that decays easily, allowing bacteria and fungi to enter the hoof and produce a necrotic core lacking an extracellular matrix (Miller et al., 2015). Such a necrotic core does not allow for the proper formation of the hoof, instead forming a hyperplastic mass, as shown in Figure 15. No link between the animal’s age, breed, or sex, has been found for the disease of keratoma. On the contrary, keratoma cases are observed at any age, breed, and sex, no matter the climate or geographical region of the ungulate (Christman, 2008).
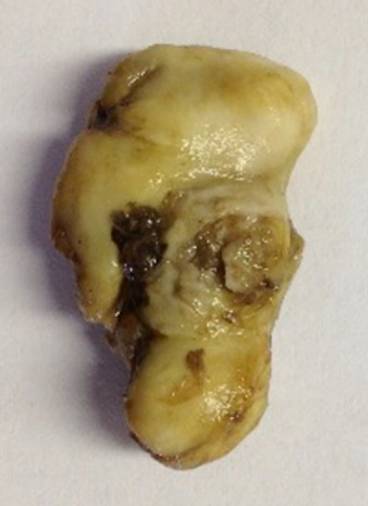
Fig. 15 Keratoma from a horse’s hoof (Miller et al., 2015).
Because of the restricted space inside the hoof, the keratoma grows downwards, generating an intense pain for the horse (Deidre et al., 2010). Keratomas also open the door for infections that can spread quickly in nearby tissues (Miller et al., 2015). However, the most significant complication a keratoma creates is that it can permanently deflect the hoof wall, changing the architecture of the hoof (Christman, 2008). Such a change can have a huge effect on the function of the hoof, the force it can provide, and the distribution of weight; keratomas can cause discomfort even after they are treated. Figure 16 shows a keratoma on the hoof and the infection in nearby tissue.

Fig. 16 Keratoma and infected tissue of a horse (Miller et al., 2015).
The precise cause for the formation of keratomas is unknown (Deidre et al., 2010). However, recent studies and observations suggest that previous trauma or chronic irritation on the hoof may play a significant role in keratoma formation, such as by causing permanent damage to the hoof wall epidermal cells (Miller et al., 2015). Such damage could prevent the cells from differentiating and inhibit the formation of the hoof. Subsequently, the cell’s feedback system detects incomplete hoof growth, causing the tumor to grow uncontrollably (Miller et al., 2015).
Since a single keratoma can negatively impact the animal’s life, it is important to diagnose the disease as early as possible. The primary evaluation is to notice any changes in the animal’s behavior, such as discomfort when walking. Examination of the sole of the animal’s hoof may also show a white tumor or infectious tissue (Deidre et al., 2010). Then, magnetic resonance imaging (MRI), radiographic imaging, or a venogram may be used to detect the presence and place of a keratoma (Osborne et al., 2021). Figure 17 shows a keratoma seen by radiography, and Figure 18 shows a different keratoma using a venogram.
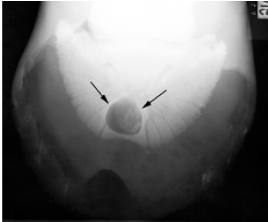
Fig. 17 Radiography of a keratoma (Deidre et al., 2010).
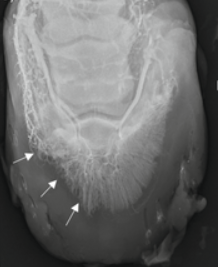
Fig. 18 Venogram Showing a keratoma (Deidre et al., 2010).
The treatment of a keratoma requires surgical removal, including hoof wall resection, meaning that a part of the hoof wall must be broken to access and remove the keratoma (Christman, 2008). Figure 19 shows three keratomas removed from a nineteen-year-old horse.
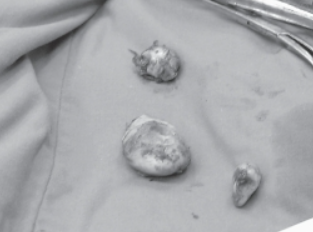
Fig. 19 Keratomas (5 cm x 4 cm, 4 cm x 3 cm, 4 cm x 2 cm) (Christman, 2008).
As seen before, hoof wall fracture is required to access the tumor. However, once the tumor is removed, veterinarians do not replace the hoof (Figure 20) (Christman, 2008).

Fig. 20 Hoof after a resection (Christman, 2008).
While hoof fracture may be the result of surgery, most of the time, a fracture or crack on the hoof occurs because of extensive forces applied on the hoof, caused by various circumstances, such as uneven terrain (Bertram & Gosline, 1986). As previously established, hoof keratin is one of the toughest biomaterials because of the crosslinking established between the fibers and the extracellular matrix, so a fracture caused by natural causes is rarely seen (Bertram & Gosline, 1986). A group of scientists performed a compact tension fracture test on horses’ hooves to show the strength of such material (Bertram & Gosline, 1986). This study proved that hooves could resist more than 400 MPa of pressure before breaking (Bertram & Gosline, 1986). They also found that the fracture behavior of the hoof depends on the intertubular orientation. This means that the fracture mostly occurs on the plane of intertubular orientation, as shown in Figure 21. Such behavior prevents the fracture from expanding inwards, towards the cells that produce keratin (Bertram & Gosline, 1986).

Fig. 21 Intertubular orientation (Bertram & Gosline, 1986).
The ungulate repairs hoof fracture, since the hoof wall cells receive feedback that there is an injury and produce more keratin to repair the hoof (Christman, 2008). However, depending on the size of the fracture, the intervention of a veterinarian may be required. In Figure 22, a fracture created by a chainsaw can be seen.

Fig. 22 Hoof fracture created by a chainsaw (Chaffin et al., 1989).
In such cases, the doctors cleaned the wound to avoid infections and applied a bandage to keep the hoof clean. No other treatment was needed since the structure of the hoof was not compromised (Chaffin et al., 1989). When the structure of the hoof is compromised, the hoof must be stabilized, and different artifacts are used, depending on the size and form of the fracture, to allow the hoof to correctly grow. Figure 23 shows an extreme example of how the hoof must be shaped for a proper recovery (Chaffin et al., 1989). Since healing from a fracture takes a long time, the horses must have patience and slowly and carefully return to normal activities (Deidre et al., 2010). In Figure 23, the horse was able to fill the holes in the hoof in approximately nine months (Deidre et al., 2010).
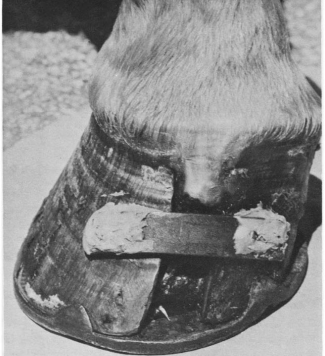
Fig. 23 Medical intervention of a hoof fracture (Chaffin et al., 1989).
Hooves after Death
Specialized conditions, such as a cold and dry climate, might allow for the fossilization of hooves for several thousand years (Zazzo et al, 2007). However, protein-based appendages are not usually well preserved in fossilization under typical conditions. Given that 90% of the hoof in dry weight is keratin (Zazzo et al., 2007), the only components of hooves which are normally capable of surviving fossilization are pigments and calcium phosphate (Saitta et al., 2017). Meanwhile, fungi and bacteria work together to decompose keratinous structures to take advantage of this protein- and nitrogen-rich biomaterial. It was found that this decomposition requires a coordinated effort of a minimum of three different keratinolytic enzymes: an endo-acting, an exo-acting and an oligopeptide-acting keratinase (Figure 24) (Lange, 2016). Knowledge about these enzymes can be applied to biotechnology methods. For instance, an industrial method to decompose hooves and other keratinous structures such as hair, teeth and claws using these three enzymes could transform these keratinous by-products into animal feed.
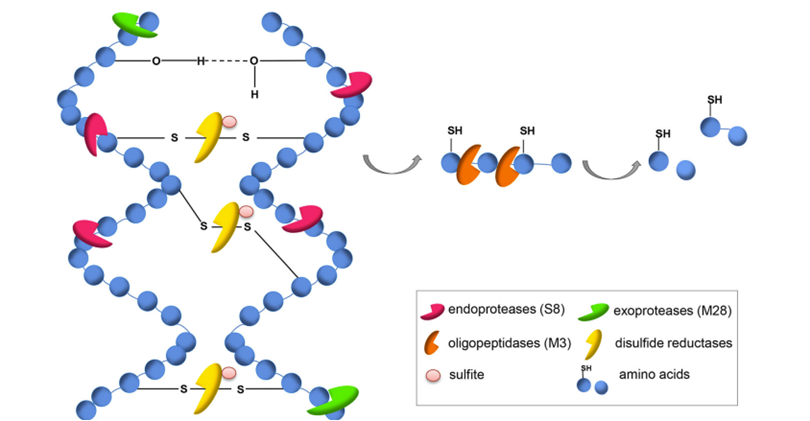
Fig. 24 The proteinaceous structure of keratin can be decomposed by a synergistic effect of three proteases: extracellular endoproteases (S8), exoproteases (M28), oligopeptidases/metalloproteases (M3), and sulfite/disulfide reductases (Lange, 2016).
A post-mortem examination of hooves can reveal information about the final months of the ungulate’s life, particularly about the dietary history of the mammal. For instance, Harrison et al. (2007) showed that a change in the concentration of the stable carbon isotopes, δ13C, in the bovine diet, is reflected in the hoof wall. Bovines were switched from a C3 diet (~ 28% δ13C) to an experimental C4 diet (~ 14.7% δ13C). Following this change, a steep increase in the δ13C value occurred (Fig. 25). Similarly, Walter (2013) discovered that the amount of δ15N in free-ranging elk (Cervus elaphus) hoof tissue was directly related to their diet. Notably, δ15N was greater in the hoof of elk who grazed on forested land and grassland than those who grazed in the Wichita Mountains Wildlife Refuge. Again, this difference correlates to the difference of δ15N in the vegetation of these two landscapes.
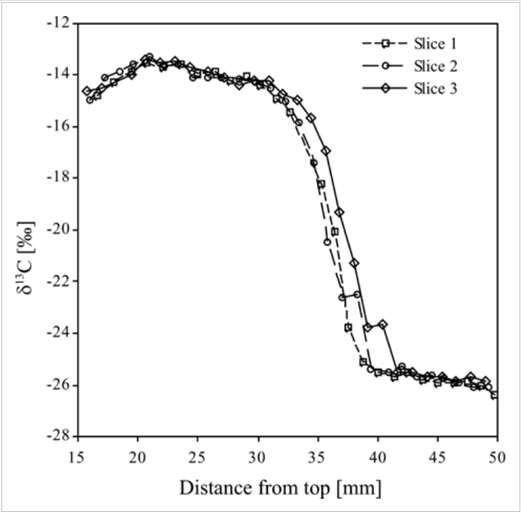
Fig. 25 Hoof δ13C obtained by high-resolution, superficial sampling on three slices of bovine hoof taken from animal 10 that received the experimental diet for 168 days (Harrison et al., 2007).
The change in the presence of δ13C and of δ15N following a diet change reveals two critical pieces of information that permit the analysis of the ungulate hoof. Firstly, the isotopic composition hoof is directly linked to the animal’s diet, and secondly, the hoof grows from the inside out such that the deeper layers of the hoof wall are younger than the outer layers (Harrison et al., 2007). These conclusions can be used when examining the presence of other isotopes, vitamins, and trace minerals in the hoof.
Conclusion
In conclusion, keratin plays an integral role in the formation of the hoof wall. The cross-linking property of keratin and tubular organization of the hoof wall allows hooves to offer mechanical support and protective functions, allowing the animal foot to resist more than 400 MPa of pressure. It is estimated that the hoof formation initiates in equine embryos around day 27 of 340 during gestation. Around day 60, differences in the thickness of the hoof wall can be observed (Franciolli et al., 2011). Once the animal is born, the chemistry of the hoof and the rate at which keratin is being produced changes as the animal grows until adulthood. In a healthy adult, once all bones are completely developed (Hanousek et al., 2020), the hoof wall grows at a rate of 0.6-1.0 cm per month (Curtis, 2017). The keratinization process depends on minerals such as calcium, phosphorus, sodium, and zinc (Fraser & MacRae. 1980). One common disease related to the incorrect process of keratinization is keratoma. In this case, the poor-quality hoof wall decays and becomes a necrotic core, where keratin does not solidify but instead creates a hyperplastic mass inside the hoof wall (Miller et al., 2015). If there is a crack in the hoof wall, the specialized formation and crosslinking of keratin does not allow the crack to propagate inside the hoof (Bertram & Gosline, 1986). Hoof cells receive feedback from the crack and start to produce more keratin to repair the damage (Christman, 2008).
Because of the structural composition and strength that keratin provides to the hoof wall, scientists have been studying if hoof keratin can be used for bioengineering applications. The cellular binding motifs of keratin mimic the cellular attachment of the extracellular matrix (Kakkar et al., 2014). For this reason, hoof keratin can be used in tissue engineering constructs to give more stability without compromising or contaminating the tissues (Kakkar et al., 2014). Bovine hooves are a solid waste from slaughterhouses that could be highly used for bioengineering applications since they have been demonstrated to be biocompatible with humans (Kakkar et al., 2014). The process to extract keratin from the hoof involves pulverizing the hoof, de-fattening the sample, cleaning the sample using different detergents for at least fourteen hours, centrifuging the sample, washing with water and drying it (Kakkar et al., 2014). Figure 26 shows pure keratin from a bovine hoof. This extracted keratin was successfully used for tissue engineering applications.
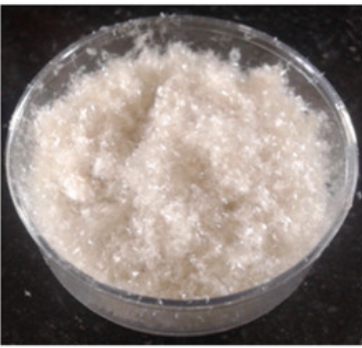
Fig. 26 Pure extracted keratin (Kakkar et al., 2014).
References
Alderman, L. (2017). Everything you need to know about laminitis [Review of Everything you need to know about laminitis]. Irongate Equine Clinic. Retrieved from https://www.irongateequine.com/education/laminitis
Bertram, J. E., & Gosline, J. M. (1986). Fracture Toughness Design in Horse Hoof Keratin. Journal of Experimental Biology, 125(1), 29–47. https://doi.org/10.1242/jeb.125.1.29
Bidwell, L. A., Bowker, R. M. (2006). Evaluation of changes in architecture of the stratum internum of the hoof wall from fetal, newborn, and yearling horses. American Journal of Veterinary Research 67(12). https://doi.org/10.2460/ajvr.67.12.1947
Bragulla, H., & Homberger, D. (2007). The role of the specific, profilaggrin-containing keratohyalin granules in the developing epidermis of the fetal horse hoof. Pferdeheilkunde, 23 (1), 5-20. https://doi.org/ 10.21836/PEM20070101
Bragulla. H. (2003). Fetal development of the segment-specific papillary body in the equine hoof. Journal of Morphology 258(2) p. 207-224. https://doi-org.proxy3.library.mcgill.ca/10.1002/jmor.10142
Bragulla, H. H., Foster, A. L., Stokes, A. M., Lopez, M. J., & Homberger, D. G. (2010). Distribution Patterns of Soft and Hard Keratin Proteins in Finger Nails, Cat Claws, and Horse Hooves. The FASEB Journal, 24(S1). https://doi.org/10.1096/fasebj.24.1_supplement.822.3
Chaffin, M. K., Carter, G. K., & Sustaire, D. (1989). Management of a Keratoma in a Horse: A Case Report. Journal of Equine Veterinary Science, 9(6), 323–326. https://doi.org/10.1016/s0737-0806(89)80066-8
Christman, C. (2008). Multiple Keratomas in an Equine Foot. The Canadian Veterinary Journal, 49, 904–906. https://doi.org/19043490
Conrad, S. E. (2017). Hoof Development: From Fetus to Maturity. Accessed on 10.29.2022 from https://thehorse.com/110031/hoof-development-from-fetus-to-maturity/
Cousins, R. J. (1996). Zinc. Pages 293–306 in Present Knowledge in Nutrition. 7th ed. E. E. Ziegler and L. J. Filer, Jr., ed. ILSI Press, Washington, DC.
Curtis, S. J. (2017). The effect of loading upon hoof wall growth and hoof shape in the Thoroughbred foal. Accessed on 10.28.2022 from https://core.ac.uk/download/pdf/132197425.pdf.
Deidre, M. (2010). Keratoma in Horses. VCA Animal Hospitals. Retrieved October 30, 2022, from https://vcacanada.com/know-your-pet/keratoma-in-horses#:~:text=What%20is%20a%20Keratoma%3F,laminae%2C%20causing%20pain%20and%20lameness.
Eckert, R. L., Sturniolo, M. T., Broome, A.-M., Ruse, M., & Rorke, E. A. (2005). Transglutaminase Function in Epidermis. Journal of Investigative Dermatology, 124(3), 481–492. https://doi.org/10.1111/j.0022-202x.2005.23627.x
Ede, D. R., Farhang, N., Stover, J. D., & Bowles, R. D. (2017). 4.32 Gene Editing Tools. Comprehensive Biomaterials II, 589–599. https://doi.org/10.1016/b978-0-12-803581-8.09290-0
Fass, D., & Thorpe, C. (2017). Chemistry and Enzymology of Disulfide Cross-Linking in Proteins. Chemical Reviews, 118(3), 1169–1198. https://doi.org/10.1021/acs.chemrev.7b00123
Faramarzi, B., Thomason, J. J., & Sears, W. C. (2009). Changes in growth of the hoof wall and hoof morphology in response to regular periods of trotting exercise in Standardbreds. American Journal of Veterinary Research, 70(11), 1354–1364. https://doi.org/10.2460/ajvr.70.11.1354
Florence, L. & McDonnell, S. M. (2006). Hoof growth and wear of semi-feral ponies during an annual summer ‘self-trimming’ period. Equine Veterinary Journal, 38(7), p. 642-645. https://10.2746/042516406X158350
Fox, D. G., & Tylutki, T. P. (1998). Accounting for the Effects of Environment on the Nutrient Requirements of Dairy Cattle. Journal of Dairy Science, 81(11), 3085–3095. https://doi.org/10.3168/jds.s0022-0302(98)75873-4
Fraser, B., & MacRae, T. P. (1980). Molecular structure and mechanical properties of keratins. The Mechanical Properties of Biological Materials p. 211-246. Cambridge University Press, Cambridge, UK.
Fraser, B., & MacRae, T. P., & George Ernest Rogers. (1972). Keratins: Their Composition, Structure, and Biosynthesis. Charles C. Thomas Publisher.
George, A. N. (2017). The Post-Natal Development of the Horn Tubules and Fibres (Intertubular Horn) in the Horns of Sheep. British Veterinary Journal 112(1) 30-32. https://doi.org/10.1016/S0007-1935(17)46855-2
Gerard, M. P. (2021). Anatomy and Physiology of the Equine Foot. Veterinary Clinics: Equine Practice, 37(3). https://doi.org/10.1016/j.cveq.2021.07.002
Grovenburg, T. W. et al. (2014). Re-Evaluating Neonatal-Age Models for Ungulates: Does Model Choice Affect Survival Estimates? PLoS ONE 9(9): e108797. doi:10.1371/journal.pone.0108797
Halper J, Kjaer M. 2014. Basic components of connective tissues and extracellular matrix: elastin, fibrillin, fibulins, fibrinogen, fibronectin, laminin, tenascins and thrombospondins. In: Halper J. (eds) Progress in heritable soft connective tissue diseases. Advances in experimental medicine and biology, vol 802. Springer, Dordrecht
Harrison, S. M. et al. (2007). Three-dimenstional growth of bovine hoof as recorded by carbon stable isotope ratios. Wiley InterScience. https://10.1002/rcm.3309
Hendry, K. A. K., MacCallum, A. J., Knight, C. H., & Wilde, C. J. (2001). Synthesis and distribution of cytokeratins in healthy and ulcerated bovine claw epidermis. Journal of Dairy Research, 68(4), 525–537. https://doi.org/10.1017/s0022029901005052
Hanousek, K., Salavati, M., & Dunkel, B. (2020). The Impact of Horse Age, Sex, and Number of Riders on Horse Performance in British Eventing Horse Trials. Journal of Equine Veterinary Science, 94, 103250. https://doi.org/10.1016/j.jevs.2020.103250
Hsu, D. J., Daniel, J. C., & Gerson, S. J. (1991). Effect of zinc deficiency on keratins in buccal epithelium of rats. Archives of Oral Biology, 36(10), 759–763. https://doi.org/10.1016/0003-9969(91)90042-s
Jadotte, Y. T., & Schwartz, R. A. (2012). Solar cheilosis: An ominous precursor. Journal of the American Academy of Dermatology, 66(2), 173–184. https://doi.org/10.1016/j.jaad.2011.09.040
Jennings, R. (2017). Specialized Anatomic Sites. Pressbooks.pub; The Ohio State University. Retrieved on October 29 from https://ohiostate.pressbooks.pub/vethisto/chapter/7-specialized-anatomic-sites/
Josseck, H. (1991). “Hufhornveranderungen bei Lipizzanerpferden und ein behandlungsversuch mit biotin.” Inaugural-Dissertation. Zurich: University of Zurich.
Kasapi, M. A., & Gosline, J. M. (1997). Design complexity and fracture control in the equine hoof wall. Journal of Experimental Biology, 200(11), 1639–1659. https://doi.org/10.1242/jeb.200.11.1639
Kakkar, P., Madhan, B. & Shanmugam, G. (2014). Extraction and characterization of keratin from bovine hoof: A potential material for biomedical applications. SpringerPlus 3(596). https://doi.org/10.1186/2193-1801-3-596
Lange, L., Huang, Y. & Busk, P.K. (2016). Microbial decomposition of keratin in nature—a new hypothesis of industrial relevance. Appl Microbiol Biotechnol 100, 2083–2096. https://doi-org.proxy3.library.mcgill.ca/10.1007/s00253-015-7262-1
Leccia, E., Gourrier, A., Doucet, J., & Briki, F. (2010). Hard alpha-keratin degradation inside a tissue under high flux X-ray synchrotron micro-beam: A multi-scale time-resolved study. Journal of Structural Biology, 170(1), 69–75. https://doi.org/10.1016/j.jsb.2009.11.006
Lim, P. S., Sutton, C. R., & Rao, S. (2015). Protein kinase C in the immune system: from signalling to chromatin regulation. Immunology, 146(4), 508–522. https://doi.org/10.1111/imm.12510
Logesh Kumar, S., Anandhavelu, S., Sivaraman, J., & Swathy, M. (2017). Modified extraction and characterization of keratin from Indian goat hoof: A biocompatible biomaterial for tissue regenerative applications. Integrated Ferroelectrics 184(1), p.41-49, https://doi.org/10.1080/10584587.2017.1368642
Mayor, P. et al. (2019). Embryonic and fetal development of the red brocket deer (Mazama americana). Theriogenology 134 53-64. https://doi.org/10.1016/j.theriogenology.2019.05.015
McLure, R. (1999). Functional anatomy of the horse foot [Review of Functional anatomy of the horse foot]. University of Missouri; MU Extension, University of Missouri. https://extension.missouri.edu/publications/g2740
Miller, S. M., & Katzwinkel, R. H. (2015). Solar Keratoma: An Atypical Case. Journal of the South African Veterinary Association, 86(1), 1257–1258. https://doi.org/10.4102/jsava.v86i1.1257
Molecare. (2016). Laminitis – Molecare Veterinary Services. Molecare Veterinary Services. Retrieved on October 29 from https://www.molecarevetservices.com/laminitis/
Morton, S. U., & Brodsky, D. (2016). Fetal Physiology and the Transition to Extrauterine Life. Clinics in perinatology, 43(3), 395–407. https://doi.org/10.1016/j.clp.2016.04.001
Nunes Barreto, R. d. S., et al. (2016). Organogenesis of the Musculoskeletal System in Horse Embryos and Early Fetuses. American Association for Anatomy: The Anatomical Record 299(6). https://doi.org/10.1002/ar.23339
Osborne, C., Elce, Y. A., Meehan, L., Davern, A. J., & Lescun, T. B. (2021). Neoplasia within the Equine Foot: A Retrospective Case Series of Four Horses. Equine Veterinary Education, 34(10). https://doi.org/10.1111/eve.13523
Ovnicek, G. (2003). Natural balance trimming and shoeing: appearance of a selfmaintained foot, In: Lameness in the Horse, Eds: M. Ross and S. Dyson, W.B. Saunders, St. Louis, pp 271-273.
Pecora, K. (2022). Hoof structural integrity, formation and the importance of hard alpha-keratin [Review of Hoof structural integrity, formation and the importance of hard alpha-keratin]. AG Proud; Progressive Publishsing. https://www.agproud.com/articles/54351-hoof-structural-integrity-formation-and-the-importance-of-hard-alpha-keratin
Pollitt, C. C. (2010). The anatomy and physiology of the hoof wall. Equine Veterinary Education, 10(S4), 3–10. https://doi.org/10.1111/j.2042-3292.1998.tb01775.x
Pollitt, C. (2017). The anatomy and physiology of the suspensory apparatus of the distal phalanx. Undefined; https://www.semanticscholar.org/paper/The-anatomy-and-physiology-of-the-suspensory-of-the-Pollitt/84d4ef014d83020858a91bcc8c402eb77cad882d
Rabelo, R. E., et al. (2015). Histomorphological evaluation of the digital coronary region at different fetal development stages of Holstein cattle. Arq. Bras. Med. Vet. Zootec 67(1) 1-6. http://dx.doi.org/10.1590/1678-7529
Saitta, E. T., et al. (2017) Low fossilization potential of keratin protein reveal by experimental taphonomy. Wiley Online Library 60(4). https://doi.org/10.1111/pala.12299
Sárdy, M., Kárpáti, S., Merkl, B., Paulsson, M., & Smyth, N. (2002). Epidermal Transglutaminase (TGase 3) Is the Autoantigen of Dermatitis Herpetiformis. Journal of Experimental Medicine, 195(6), 747–757. https://doi.org/10.1084/jem.20011299
Sherry M., L., Charlotte E., H., & Duane E., U. (2005). Nutrition and Nutritional Diseases. The Laboratory Primate, 181–208. https://doi.org/10.1016/b978-012080261-6/50013-1
Tomlinson, D. J., Mulling, C. H., Fakler, T. M. (2004). Invited Review: Formation of Keratins in the Bovine Claw: Roles of Hormones, Minerals, and Vitamins in Functional Claw Integrity. Journal of Dairy Science 87 797-809. Accessed on 10.29.2022 from https://www.journalofdairyscience.org/article/S0022-0302(04)73223-3/pdf
Walsh, M. P. (1983). Review Article Calmodulin and its roles in skeletal muscle function. Canadian Anaesthetists’ Society Journal, 30(4), 390–398. https://doi.org/10.1007/bf03007862
Wang, B., Yang, W., McKittrick, J., & Meyers, M. A. (2016). Keratin: Structure, mechanical properties, occurrence in biological organisms, and efforts at bioinspiration. Progress in Materials Science, 76, 229-318. https://doi.org/10.1016/j.pmatsci.2015.06.001
Wattle, O. (2000). Cytokeratins of the Stratum Medium and Stratum Internum of the Equine Hoof Wall in Acute Laminitis. Acta Veterinaria Scandinavica, 41(4), 363–379. https://doi.org/10.1186/bf03549627
Walter, D. (2014). Use of stable isotopes to identify dietary differences across subpopulations and sex for a free-ranging generalist herbivore, Isotopes in Environmental and Health Studies, 50:3, 399-413, DOI: 10.1080/10256016.2014.875545
Werner, T. F., & Jo, A. M. S.-F. (2005). Hoof growth in neonatal patagonian huemul (hippocamelus bisulcus): a tentative tool for aging. Mastozoología Neotropical, 12(2), 245–248. Retrieved October 30, 2022, from http://ref.scielo.org/4c52jk.
Yang, Q., Pinto, V., Duan, W., Paxton, E. E., Dessauer, J. H., Ryan, W., & Lopez, M. J. (2019). In vitro Characteristics of Heterogeneous Equine Hoof Progenitor Cell Isolates. Frontiers in bioengineering and biotechnology, 7, 155. https://doi.org/10.3389/fbioe.2019.00155
Zazzo, A. et al. (2007). Experimental determination of dietary carbon turnover in bovine hair and hoof. Canadian Journal of Zoology. https://doi-org.proxy3.library.mcgill.ca/10.1139/Z07-110