Abstract
People often argue about which field of science is best to describe life. Is it physics, chemistry, or could it be math? For Daphnia, chemistry surely plays an important role in its activity and in explaining its interactions with both other animals and their environment. Over the centuries, Daphnia have mastered many challenges in biochemistry, organic and inorganic chemistries, and thermodynamics. People could say they are the original “Plastics”. From their morphology to their gene expression, they can change many parts of anatomy and biology. Indeed, in a previous paper, it was shown that Daphnia exhibit inducible morphological changes that thwart predators. This paper will explain that not only do predators influence water fleas, but different contaminants as well as temperature can influence them. In a world more and more at risk because of pollution, these zooplanktons are both an indicator of how bad the situation is, but also a possible remediator to this scourge.
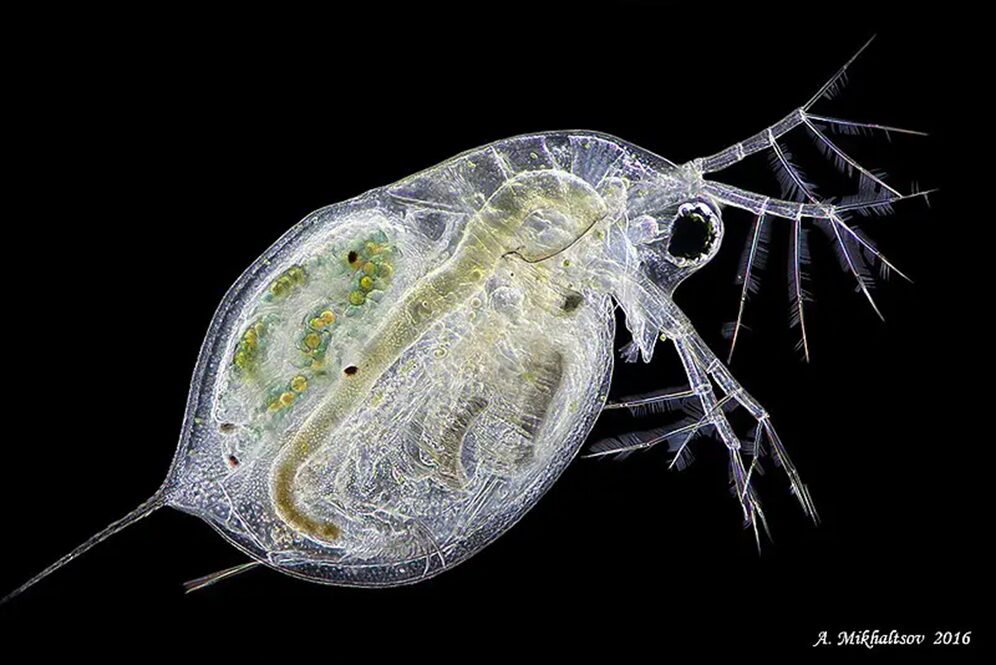
Figure 1: Dark field microscopy of a Daphnia (Mikhaltsov, 2016).
Introduction
Daphnia, commonly referred to as water fleas, are diminutive freshwater crustaceans that fulfill an essential ecological role as efficient filter feeders and a vital nutritional resource for larger aquatic inhabitants, particularly fish. These remarkable organisms possess a highly sensitive chemoreceptor system that enables them to discern kairomones and chemical signals emitted by other organisms, which can trigger specific behavioural and physiological responses. When Daphnia detects these cues, they employ various defense mechanisms, including developing more prominent spines, helmets, and even adjustments in their reproductive strategies to bolster their survival prospects. Chemical interactions via kairomones underpin a spectrum of ecological relationships, influencing predator-prey dynamics and reproductive behaviours. Extensive research has delved into the effects of pollutants on Daphnia, with Daphnia magna being a longstanding bioindicator used to gauge the impacts of substances like metals, pharmaceuticals, and pesticides on aquatic ecosystems. In response to environmental stressors, Daphnia exhibits a repertoire of reactions, from modifications in behaviour and reproduction to alterations in their resilience. These responses provide critical insights into the condition of their environment and the magnitude of the encountered stressors. Notably, Daphnia serves a dual role as a sentinel species, serving as a biological early warning system, detecting harmful substances in aquatic environments. Furthermore, these resilient organisms can be harnessed as bioremediators, proficiently removing specific chemicals from wastewater. Observing the adaptation of Daphnia‘s genome across generations offers valuable insights into their evolving response to environmental chemicals. These multifaceted attributes of Daphnia render them invaluable in comprehending and managing the complex interplay between organisms and their chemical surroundings.
The Relationship Between Kairomones and Daphnia
Overview
Daphnia have a special ecological relationship with kairomones which are chemical substances emitted by other organisms that may elicit a particular behavioural or physiological response within these crustaceans. Upon detection of such substances through their sensitive chemoreceptor system, Daphnia may develop protective features, such as larger spines or helmets, and even adjust their reproductive strategy to enhance survival rates. Within aquatic ecosystems, chemical signals are fundamental in orchestrating a variety of organismal interactions that include both predatory dynamics and reproductive behaviors. Moreover, the evolution of prey sensitivity to kairomones is advantageous for prey survival and has been favoured by natural selection. Despite the chemical and temporal complexity of these signals, their detection and quantification are essential for understanding the ecology of predator-prey interactions, which hinges on identifying specific chemoreceptors and their ligands (Weiss, 2019).
Detection of Kairomones
Diving into the specifics of detecting kairomones, chemoreceptors are what allows the marvel to happen. They include olfactory receptors (ORs) and gustatory receptors (GRs are specialized proteins that bind to chemical cues and are located on chemosensory organs (Weiss et al., 2015). For Daphnia, their first antennae serve as a primary site for chemoreception, and are simple, hair-like structures that are constantly moving and come into direct contact with the surrounding water (Weiss et al., 2015). These contain sensory cells that detect chemical cues dissolved in the water.
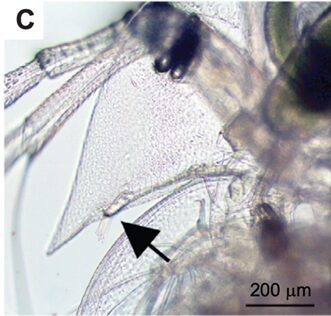
Figure 2: First antennae of female D. longcicephela shown by black arrow (Weiss, 2019).
Around 1,000 ORs are identified within vertebrates which are part of the G-protein coupled receptors (GPCR) family and exclusive to vertebrates. In contrast, insects possess their own unique groups of ORs and GRs that function differently and are not homologous to those in vertebrates (Weiss, 2019). More specifically, ORs are primarily responsible for detecting dissolved substances similar to odors through receptors, such as antennae, and GRs focus on the detection of soluble chemicals, similar to how taste works in terrestrial animals (Weiss, 2019). With only 58 chemoreceptors identified, Daphnia‘s chemoreceptors are less understood compared to those in insects and mammals. No insect or like genes are found in Daphnia, suggesting a more recent evolutionary expansion of these genes with the rise of hexapods (Weiss, 2019). The complete understanding of environmental chemical cue processing in Daphnia requires further research.
In particular, the Chaoborus is well-studied kairomone present in many chemical interactions with Daphnia. They are released by the fourth instar phantom midge larvae during the digestion and egestion process after preying on Daphnia and are composed of fatty acids attached to an L-glutamine residue (Weiss, 2019). This compound prompts other Daphnia to develop defensive neckteeth which is highly indicative of a notable predation risk. The kairomone’s molecules, lipidated L-glutamine conjugates, are water-soluble and effective as communication chemicals (Weiss, 2019). This continuous production renders the kairomone a non-dietary, activity-dependent signal that benefits only the prey as a reliable cue for predatory presence and danger (Weiss, 2019).
Neuronal Process
Now, taking a look at what happens after the kairomones are detected. In the complex neural networks of both vertebrates and invertebrates, olfactory receptor neurons (ORNs) with chemoreceptors initiate odor detection, transmitting signals to glomeruli where synaptic processing occurs in structures integral to learning and innate behaviors (Weiss, 2019). This system utilizes projection neurons (PNs) and local neurons (LNs) that may exhibit excitatory or inhibitory effects. While the nervous system of Daphnia is less complicated, these organisms are able to differentiate various signals, indicating a sophisticated level of neural integration (Weiss, 2019). Daphnia’s brain shares structural similarities with other arthropods, featuring three distinct regions interconnected with sensory components, such as antennae which are crucial for predator cue detection (Weiss, 2019). In addition, Daphnia exhibit cellular elements outside the nervous system, such as large polyploid cells near defensive structures, which are believed to contribute to the development of defensive traits through signaling molecules (Weiss, 2019).
Predator-Induced Morphological Changes
There are several examples of morphological changes that occur amongst Daphnia upon processing of kairomones. First, the helmet development in Daphnia cucullata is a fascinating example of phenotypic plasticity, where the presence of predator cues can induce the formation of a protective “helmet” structure in the water fleas (Weiss et al., 2015). This adaptation is known to be an evolutionary response that aims to reduce vulnerability to predation, particularly from filtering feeders that might ingest smaller or more regular-shaped prey. Specifically, the helmet increases the body size appearance of D. cucullata, making them less likely to be consumed or at least more difficult to handle, thereby improving their survival chances in environments where predators are present (Weiss et al., 2015). Another example is in response to the predatory larvae of Chaoborus, Daphnia pulex exhibit the remarkable ability to develop protrusions known as neckteeth. These structures are induced by predator-released chemical cues and are specifically formed along the dorsal head and neck region of the water flea (Weiss et al., 2015). The neckteeth functions as an anti-predatory adaptation, deterring predation by making D. pulex less palatable or more difficult to capture and ingest for the Chaoborus larvae (Weiss et al., 2015). Moreover, Daphnia longicephala exhibits a specific morphological change in the presence of heteropteran backswimmers that are known to be agile and visual predators. To counter this, D. longicephala develops an elongated crest that extends from its head, altering its body shape and motion in water, which not only makes itself less recognizable as prey but also may interfere with the hydrodynamic cues that predators use to detect their movements (Weiss et al., 2015). The crest development is a key survival tactic for D. longicephala, providing a clear example of morphological change driven by the need to evade predators. Further, the introduction of fish predators can lead to a significant transformation in Daphnia lumholtzi, where they develop pronounced head and tail spines. These spines act as a physical defense mechanism, increasing the size and altering the shape of D. lumholtzi to reduce the risk of predation (Weiss et al., 2015). The spines can make them more challenging to swallow and may injure or discourage predators (Weiss et al., 2015). Finally, upon predation by tadpole shrimp, Triops sp., Daphnia atkinsoni has evolved the remarkable “crown of thorns” structure. This crown consists of pointed projections that surround the body, providing a mechanical defense against the grasping and ingestion techniques of their shrimp predators (Weiss et al., 2015). The crown not only makes D. atkinsoni harder to capture and consume but also increases the energy expenditure required by the predator to handle them (Weiss et al., 2015). This morphological adaptation signifies a direct response to the specific hunting behavior of tadpole shrimp, illustrating a targeted evolutionary approach to survival in hostile environmental conditions (Weiss et al., 2015). In particular, certain cuticle proteins and chitin-modifying enzymes cause carapace fortification in kairomone-exposed Daphnia (Otte et al., 2014). Further details on the chemicals and enzymes involved require additional long-term research. As can be seen below, several of these examples are displayed in Figure 3.
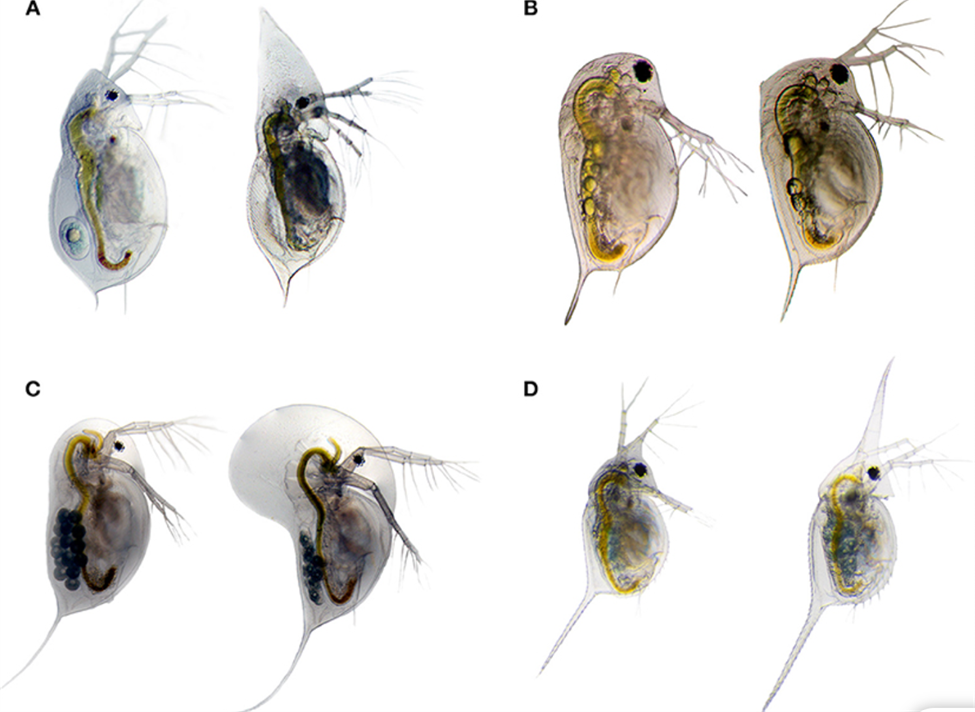
Figure 3: Inducible morphological defenses that include helmet expression in D. cucullata (A); crest expression in D. longicephala (C); head- and tail-spine formation D. lumholtzi (D), and neckteeth expression in D. pulex (B). Undefended morphotypes are displayed on the left side, and the defended morphotype on the right side (Weiss, 2019).
Changes in Life-History Traits
As an adaptive response to increased predatory danger, the presence of kairomones from predators instigates significant alterations in the life history traits of Daphnia. For example, when exposed to kairomones, Daphnia often exhibits a reduction in size at maturity, making it rather difficult for larger visual predators, such as fish, to detect and prey on them. — especially during the onset of unfavorable conditions, allowing them to produce hardy resting eggs that can withstand harsh conditions and hatch when the environment becomes more conducive. This strategy maximizes the longevity of their lineage in the face of threats from predators. Further, Daphnia may also adjust the timing of their reproduction cycle, opting for earlier maturation and increased rates of egg production to quickly generate new generations prior to the impact of predation on their survival. These phenotypic changes in Daphnia illustrate the dynamic and responsive nature of their life history strategies in the presence of predator cues. As displayed below, one life-history adaptation with regards to the size of the offspring is observed in Figure 4. Moreover, such size and shape alterations, as well as reproductive changes, are controlled by many genetic and hormonal factors, specifically those involve in the juvenile hormone or ecdysteroid pathways (Miyakawa et al., 2018).
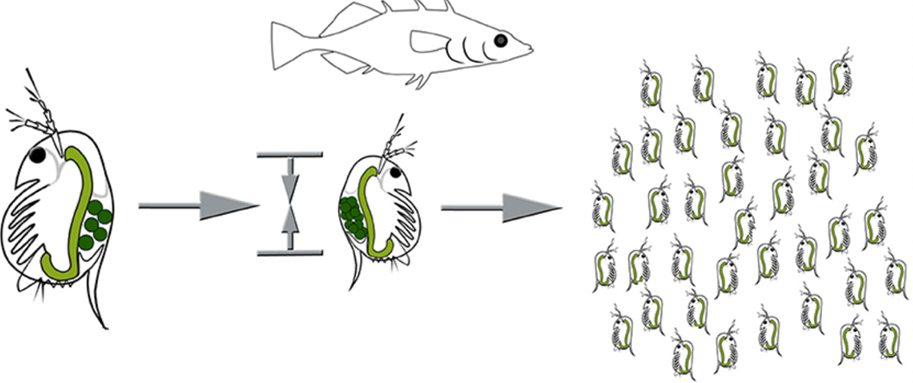
Figure 4: Life-history adaptations under fish predation, where resources are shifted from somatic growth to reproduction resulting in earlier sexual maturity at a smaller size and increased number of smaller offspring (Weiss, 2019).
Changes in Behavioural Patterns
Behavioral patterns of Daphnia are also significantly impacted by kairomone concentrations. Such chemical substances not only alert Daphnia to the presence of predators but also communicates information about the surrounding predator density. The amount of kairomones detected by Daphnia correlates strongly with their defensive behaviors. For instance, higher concentrations of kairomone drive their diel vertical migration (DVM) which is a type of phototactic behavior to descend deeper into the ocean during daylight hours to elude predators that rely on light to hunt effectively (Van Gool & Ringelberg, 2002). This migration is particularly pronounced in lakes where light penetration decreases with depth due to the absorption and scattering of the water. As light intensity decreases at night, Daphnia returns to near the surface. Daphnia possess sophisticated decision-making mechanisms that allow them to adjust their swimming depth in response to changing light conditions, which is a clear indication of their ability to interpret and respond to environmental cues. In fact, this behaviour highlights an assessment of risk versus reward. That is, in dimmer light, the reduced risk of predation allows Daphnia to venture nearer to the surface to access a greater abundance of food. This pattern additionally suggests the presence of an internal biological clock as Daphnia align their movements with the diurnal cycle, which altogether demonstrates their adaptive behaviour and survival strategy optimization. However, it’s essential to recognize that DVM comes at a cost as the energy expenditure and potential for missing out on food at higher layers are considerations Daphnia must balance against the reduced risks of predation (Van Gool & Ringelberg, 2002). Notably, DVM is triggered only when perch predators exceed 12 mm in length, suggesting a size threshold for this defense strategy (Van Gool & Ringelberg, 2002). Research indicates that Daphnia do not react to newly hatched fish as they release lower levels of kairomone and, thus, do not pose a significant threat. As the fish grow, they emit more kairomones, signaling a greater danger to Daphnia, and once the fish reach a certain size capable of predating Daphnia, their kairomone concentration becomes sufficient to initiate DVM or morphological changes. In fact, the intensity of kairomone released is closely related to the fish’s maturity and predatory capabilities, allowing Daphnia to effectively gauge the risk of predation and respond accordingly with adaptive behaviors (Van Gool & Ringelberg, 2002). Moreover, swarming behavior in Daphnia is a collective movement and grouping mechanism that serves as an important defense strategy for these small aquatic crustaceans. By swarming, they enhance their survival chances significantly since the dense formation of a swarm complicates a predator’s ability to detect and target individual Daphnia as opposedto when they are dispersed and more vulnerable (Pijanowska & Kowalczewski, 1997). This swarming can also disrupt a predator’s ability to predict individual movements, decreasing the success rate of predation. In ways, swarming of Daphnia takes advantage of the sensory overload a predator may experience when faced with a large, moving group of Daphnia, making it arduous to single out one individual to attack (Pijanowska & Kowalczewski, 1997).
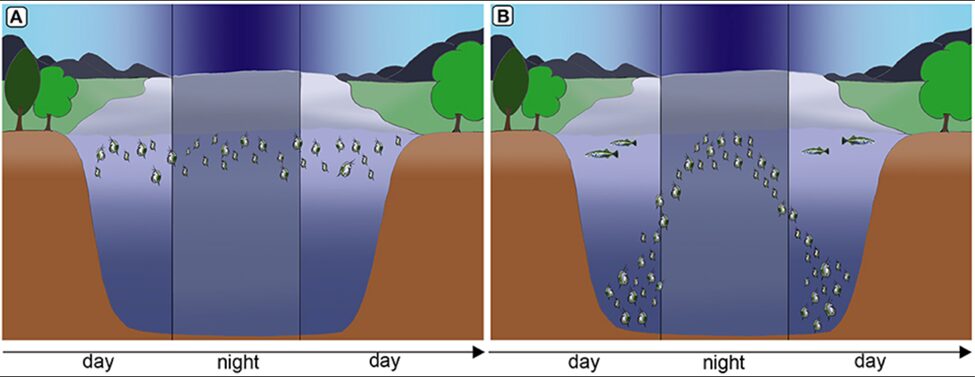
Figure 5: In the absence of fish predation, Daphnia remain in warm and nutritious water strata (A) but under predation, Daphnia swim to deeper water seeking refuge from visual predators and during the night they migrate to nutrient rich and warmer strata for feeding (B) (Weiss, 2019).
Impact of Other Chemicals on Daphnia
The growing concern over the effects of pollutants on aquatic life has highlighted how monitoring indicator species can offer a meaningful understanding of the impacts of environmental stressors within such fragile ecosystems. D. magna, a Daphnia species, has long acted as a biomonitor by indicating the impacts of chemicals such as metals, pharmaceuticals, and pesticides on aquatic settings. When confronted with stressors from its environment, the tiny water flea can react in various ways, such as alterations in both its behaviour and reproduction alongside impacts to its endurance. From analyzing these responses, one can measure both the condition of the surroundings as well as the intensity of the troubling force. Biomonitoring species such as D. magna serve as invaluable instruments for environmental monitoring and assessment by revealing insights into the prolonged impacts of environmental stressors on ecosystems and their rich diversity through the information provided.
Daphnia as a Biomonitoring Species
The impact of chemicals such as metals, drugs, pesticides, and other pollutants on D. magna can induce changes in behaviour and other physiological responses (Ren et al., 2009) (Egan et al., 2023). For example, the influences of temperature, pH, and turbidity on the behavioural responses of D. magna have been studied (Ren et al., 2009). The toxicological reactions of D. magna to environmental pollutants have been well characterized, and the use of “omics” technologies exposed to real-world ambient chemical mixtures has shown improvements at detecting bioactive components of chemical mixtures, determining the potential effects of untested chemicals within mixtures, and identifying targets of toxicity (Abdullahi et al., 2022). Omics technologies help better understand the toxicological reactions of Daphnia magna to various pollutants, providing insights into the effects of contaminants on this organism. For example, a study conducted by (Oliveira Pereira et al., 2023) demonstrated that Daphnia magna sub-lethal exposure to phthalate pollutants elicited disruptions in amino acid and energy metabolism, highlighting the use of targeted metabolomic approaches to understand the toxic effects of pollutants on this organism (Oliveira Pereira et al., 2023). The ecotoxicological review of microplastics’ effects on Daphnia magna underscored the necessity of comprehending the multilevel qualities of Daphnia in response to pollutants, signifying the applicability of omics technologies in evaluating the influence of microplastics on this organism (Yin et al., 2023). Therefore, omics technologies have significantly contributed to the understanding of toxicological reactions of Daphnia magna to environmental pollutants, providing valuable insights into the effects of contaminants on this important bioindicator organism. Studies, like the one conducted by (Egan et al., 2023), have shown that exposure to chemicals can affect the behaviour of D. magna. For instance, pesticides of concern like CHL and IMI, as components of agricultural surface water, can alter the swimming behaviour of D. magna (Egan et al., 2023). Additionally, D. magna exposed to gradient concentrations of deltamethrin, chlorothalonil, and nitrogen showed changes in their behavioural responses (Ren et al., 2009). Figures 5, 6, and 7 illustrate the impact of deltamethrin, chlorothalonil, and nitrofen on the behaviour of D. magna during a 48-hour exposure period. In the control group, there were no significant alterations in behavioural responses. However, when exposed to deltamethrin, there were changes in the strength of behaviour over time and with increasing concentrations. Higher concentrations led to more pronounced behavioural responses and a shorter time for the initial phase of behaviour, which was identified as avoidance behaviour. Interestingly, even at sublethal concentrations (0.1 TU), the initial phase of behavioural response was observable.
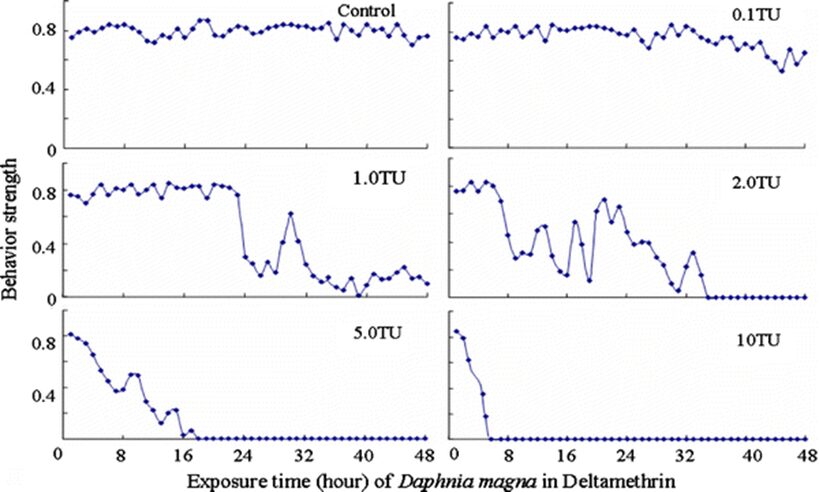
Figure 6: The effects of deltamethrin on the behavioral responses of D. magna in 48-h exposure (*p ≤ 0.05) (Ren et al., 2009).
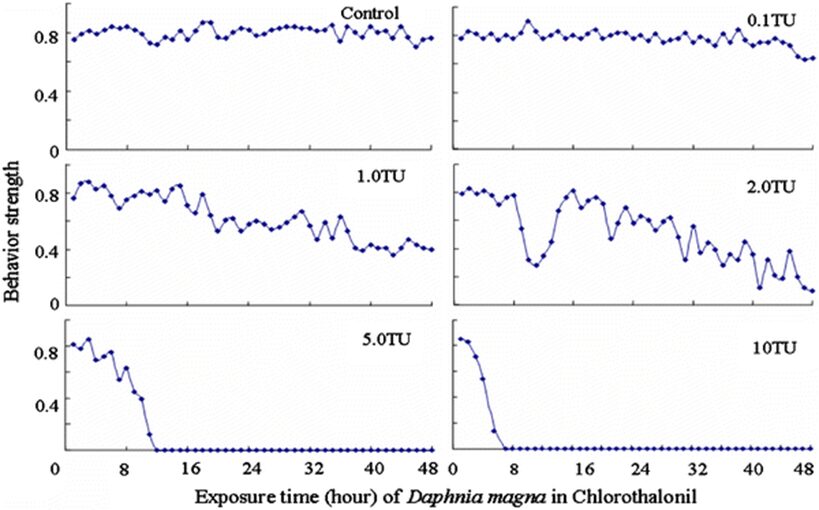
Figure 7: The effects of chlorothalonil on the behavioral responses of D. magna in 48-h exposure (*p ≤ 0.05) (Ren et al., 2009).
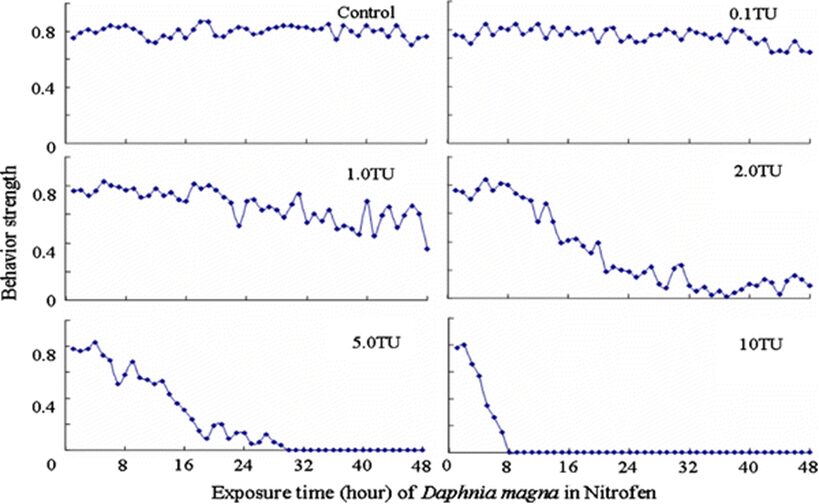
Figure 8: The effects of nitrofen on the behavioral responses of D. magna in 48-h exposure (*p ≤ 0.05) (Ren et al., 2009).
In sum, an assortment of elements such as metals, medications, pesticides, and additional contaminants are capable of inducing modifications in conduct as well as physiological reactions inside the D. magna owing to their introduction into its environment. The toxicological responses of D. magna to environmental pollutants have been extensively studied. Applying “omics” technologies to Daphnia exposed to real-world ambient chemical mixtures has improved the detection of bioactive components, determination of potential effects of untested chemicals within mixtures, and identification of toxicity targets.
Example of a Technology Using Daphnia as a Sentinel Species
It is known that Daphnia can react to chemicals released by predators in their environment; however, like most systems, they are also able to react to other different chemicals that they may absorb. For example, collecting water containing contaminants from nearby sources and exposing species of Daphnia to the contaminants induces changes in the organisms, whether it may be physiological or behavioral. Observing these behavioral changes may be very useful for humans as a means of detecting pollutants or other chemical substances. When using Daphnia in this way, the organism can be called a sentinel species.
Sentinel species are animals used by humans in specific situations to detect harmful substances for people. An example of sentinel species would be the canaries used in mines or caves. Miners would bring a canary in a cage with them on expeditions to find out if there were any toxic gases in the environment. While seemingly cruel, by observing the state of the canary and if it is agitated or not, miners would be able to determine whether it was safe to continue (Abdullahi et al., 2022). The usage of Daphnia as a sentinel species would not be as harmful to the animal as it was for the canaries in the example, since Daphnia are able to adapt themselves to many different chemicals in its environment.
The researchers proceeded with a 3-step process to identify the presence of contaminants in different samples. The first step was using different identification techniques to find out what contaminants were present in the water. When explaining how Daphnia can be used in detection of dangerous substances, the identity of the specific molecules that were observed is not important. In the second step, Daphnia are placed in the different water samples to observe putative molecular key events. Putative molecular key events are, put simply, the expression of co-response genes and metabolites which are the cause behind the induced changes in the animal. Finally, step three is the correlation between the chemicals present in the different samples and the induced changes in the microorganisms. (Abdullahi et al., 2022) Using these observations, placing the Daphnia in other samples in the future would help determine whether there is presence of the contaminants in the samples. The report published shows which chemicals induce which reactions, which can be helpful when looking into the use of these organisms as chemical detectors in other water sources with similar contaminants. For example, D. magna used for the experiments have displayed six putative molecular key events, primarily caused by three pharmaceuticals: carbamazepine, erythromycin, and trimethoprim. (Abdullahi et al., 2022) The molecular key events were detected via co-expression network analysis, which is a “data analysis technique that helps identify groups of genes with similar expression patterns across several different conditions” (Montenegro, 2022). It is important to note however that not all the contaminants present in the samples induced a change in the Daphnia. As such, Daphnia is only effective as a sentinel species for certain chemical environments. For this reason, if the organism is to be used in other locations, tests should be done first to find out if the water contains contaminants inducing key molecular reactions. Besides being able to show the presence of the contaminants, the organism’s reaction allows us to determine if the contaminants are dangerous in human systems, since “all animals, including humans, share genes that underpin their responses to environmental changes including exposure to pollution” (Water fleas as ‘canaries in a coal mine’ offer key to managing chemical pollution, 2022). Observations of changes in these genes give precious information, beneficial for the health of surrounding populations.
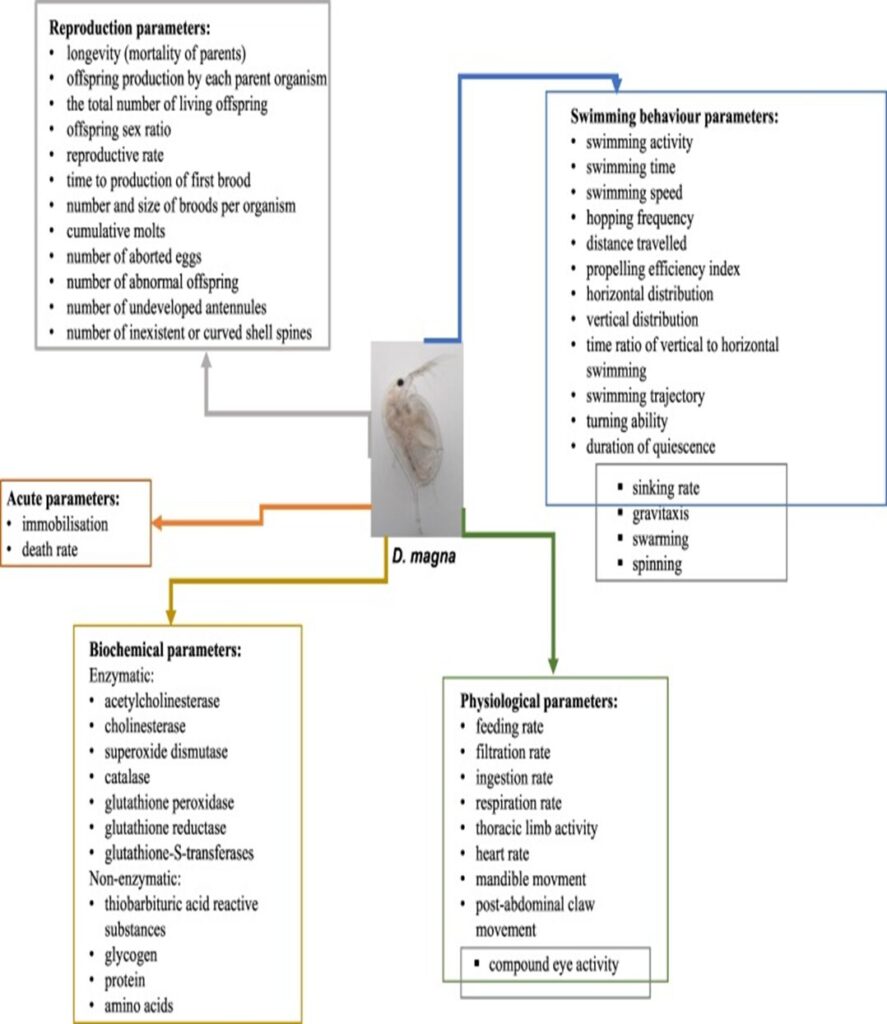
Figure 9: Observed parameters in Daphnia during the assessments of pharmaceuticals (Tkaczyk et al., 2021).
Daphnia as a Bioremediator
Other than being a possible biomonitoring species for certain contaminants in water sources, Daphnia also have bioremediation potential. “Bioremediation is a branch of biotechnology that employs the use of living organisms, like microbes and bacteria, in the removal of contaminants, pollutants, and toxins from soil, water, and other environments” (Mitchell, 2022). An experiment done at the University of Birmingham shows the possibility of reducing contaminants in water through simple steps. A pool of Daphnia (Dearn & Orsini, 2021) is simply added to a water source containing some pollutants. After filtering the water for a while, the pool of Daphnia is removed, and a decrease in the number of contaminants can be observed (Dearn & Orsini, 2021). An experiment made in Shanghai showed that the presence of Daphnia in a water sample brought a significant decrease in the amounts of total nitrogen, ammonium nitrogen, nitrate nitrogen, nitrite nitrogen, total phosphorus, and reactive phosphate (Huo et al., 2010). Another experiment done in Nordic waste waters showed that Daphnia can be used not only to filter chemical substances but can also get rid of some microalgae. “After 48 h, Daphnia had removed 80% of M. griffithii, 70% of H. pluvialis, [and] 20% of Selenastrum sp. from the wastewater” (Stevčić et al., 2020). This is only possible because Daphnia feed on some microalgae. In fact, the absorption of the different types of nitrogen is done first by the species of algae mentioned above, which can be inserted into the water source that is to be purified. In this case, Daphnia can be considered a bioremediator, but for the number of microalgae present in the water, not the chemicals. This shows the potential usage of Daphnia as a bioremediator when paired with other common bioremediators such as these algae. This is efficient when the chemicals that are present in the water is not easily absorbed by the organism as it might not be useful for its metabolism.
Another case, however, shows that Daphnia, on their own, are also good to reduce the quantity of certain chemicals present in wastewater. An experiment done in the United Kingdom compares the efficiency of Daphnia as bioremediators to the efficiency of the bacteria that are usually present in the water and to that of a certain population of algae commonly used as bioremediation agents. The results were taken after 48 hours of exposure to the environment which contained concentrations of arsenic, a biocide called atrazine and an industrial chemical; PFOS. The tests, repeated a couple of times, showed that Daphnia, on average, removed “47.3% of PFOS, 60% of atrazine, and 60% of arsenic” (Abdullahi et al., 2022). This is possible thanks to certain genes in their genome, which express detoxification and catabolic functions. The average measured was the result of 4 subgenus of Daphnia, which are used in different environments and under different chemical stress. When observing each subgenus separately, the scientists found different maximal removal rates for each species of Daphnia. This is likely explained by the fact that populations of Daphnia which have had multiple generations exposed to certain chemicals have already adapted to their environment and the pollution in the water. Through reproduction, the genotype which is primarily detectable is the one which expresses the adaptation of the organisms to the pollutants. Because of this, these populations are more susceptible to side effects when in contact with different contaminants from those they have adapted to, since there is less genotypic diversity (Abdullahi et al., 2022). This indicates that individuals deriving from subgenus who adapted to certain water environments and chemicals present are less likely to be able to interact with new chemicals. So, when presenting Daphnia already possessing a tolerant system towards PFOS, one can observe a maximum reduction of such chemicals in the water. Through this experiment, the adaptation of the Daphnia’s genome through generations can be observed, and more can be learned about the organism’s adaptation to chemicals in its environment.
Different Chemicals and Pollutants and their Effects on Daphnia
Some of the pollutants that were found to be detected by Daphnia are various metals (Kimberly & Salice, 2015), different nanoparticles (Fan et al., 2019) (Seitz et al., 2015) and microplastics (Guilhermino et al., 2021) (Yuan et al., 2020). These can have various effects and can be studied in different ways. According to research, the effects of the pollutants can be on reproduction, swimming behavior, they can be physiological, biochemical or even result in death (Tkaczyk et al., 2021). On top of that, Daphnia can adapt to those changes and these adaptations can be transmitted to their offspring as there can be transgenerational effects of exposure to pollutants. In general, when an organism is exposed to contaminants, it develops tolerance. There are two types of tolerance that are observed by biologists in organisms that can be summarized as acclimation and adaptation. Acclimation happens when the physiology of said organism changes to augment tolerance. Adaptation is a genetic change that will have effects on the offspring of the organism exposed to the pollutant. There can also be an increase in sensitivity because of the accumulation of stress. This could be caused by bioaccumulation which is the accumulation of a contaminant in an organism (Kimberly & Salice, 2015). Daphnia usually tend to adapt to the contaminant because they have an ecoresponsive genome (Colbourne et al., 2011). This means that they can do both acclimation and adaptation. It can sometimes happen that they decrease in fitness due to long exposure. Furthermore, a consequence of pollution on a given Daphnia population itself would be that the chemicals and particles can interfere with Daphnia’s chemical communication (with kairomones for example). Therefore, Daphnia cannot navigate properly as they do not have the chemical cues to know where to go to find food for example (Campbell, 2021). Some contaminants as well as Daphnia’s ecoresponsive genome will be explained in the following paragraphs.
Metals
The effects of a metal on Daphnia depend on the metal itself. For example, copper was found to affect both growth and reproduction (De Schamphelaere & Janssen, 2004). Daphnia was also used to study the effects of nickel toxicity at different temperatures (since it is a model organism, commonly used in various tests) and it was found that the toxicity of nickel indeed changes with the temperature (Pereira et al., 2019). Additionally, cadmium was found to decrease Daphnia’s reproductive output. Daphnia that were exposed to a high cadmium concentration were found to have gained a higher tolerance to the metal while organisms that were exposed to a lower quantity exhibited a decrease in tolerance to the metal. What scientists think is that they will reduce their reproduction rate as an energy-saving technique. In fact, when an organism is exposed to a stressor, to increase survival and reproductive success, it can cut off on different functions to direct more energy towards baseline metabolic activities. Also, the energy saved can be used to augment tolerance to the stressor. The scientists that conducted this research also realized that even when the cadmium was removed from the water the studied Daphnia group was in, they did not go back to “normal.” In fact, they still had a reduced reproduction rate. On top of that, some of the offspring showed sign of their parents’ past contamination. This leads to believe that the effects of contaminants can be transgenerational. This would mean that a given population that was once acclimated to a given contaminant may have trouble adjusting to a new pollutant as all their energy is concentrated on developing tolerance to a previous one. This tolerance is due to the development of protective molecules. A possible biological pathway that could undergo in Daphnia populations in contact with cadmium (or other metals) is that the metal will bind to metallothionein (MT) (Kimberly & Salice, 2015). MT is mostly used by cells as they bind to metal ions to avoid metal poisoning. This protein is responsible for increasing metal tolerance in different organisms. In D. pulex, a study identified four MT genes (MT1, MT2, MT3, MT4) (Asselman et al., 2012). The MT gene expression happens when Daphnia is in contact with many different metals: zinc, copper and cadmium. MT’s structure is composed of many cysteine residues as well as thiol groups. The parts that bind to the metal are the thiol groups. When they bind to the metal ion, it reduces itself as the metal cannot be absorbed by the organism. Therefore, the MT genes are more expressed when a Daphnia is in an environment with a high metal concentration. A proof of that is that scientists can use MT expression as a biomarker to measure metal contamination in different environments (Arao et al., 2020).
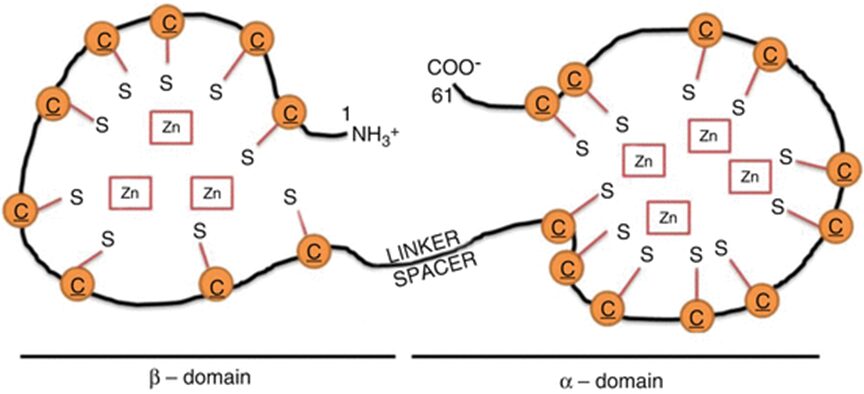
Figure 10: Structure of MT. Here, it bounded to zinc, but it could be another metal. The orange “C”s are the cysteines and the “S”, the thiol groups (Dziegiel et al., 2016).
Furthermore, as it was previously mentioned, Daphnia that are exposed to a low concentration of metal in water seem to acclimate less than another Daphnia that would be exposed to a high concentration of the same metal. Therefore, it would be acceptable to believe that the higher the concentration, the higher the protective response. However, this may be true only after a certain threshold meaning that a minimum concentration of a metal is needed to activate the expression of the MT gene, for example, and lead to a more acclimated organism. Finally, a higher expression of these genes may be transmitted down to the offspring of a Daphnia living in a contaminated environment as the effects of cadmium exposure persisted on at least one generation when scientists tested it (Kimberly & Salice, 2015).
Nanoparticles
As nanoparticles (NP) are more and more present in technology, it has been found that they can affect ecological environments. When released in the environment, because they have special properties (which is why their use is increasing in technology), nanoparticles will interact with other contaminants and sometimes the effects of that contaminant will change. These properties can change depending on the type of NP type, but their properties may be summarized into three categories: being highly mobile, having an extremely high specific surface area, and they can exhibit quantum effects (Dobson et al., 2010).Additionally, NP will occasionally release ions which can be toxic for the organisms. For example, nano-TiO2 (NPTiO) were found to adsorb arsenic. As everyone knows, arsenic is a very toxic element. The effects of arsenic depend on its oxidation state. Daphnia were found to be more sensitive to arsenic with an oxidation state of +5 (As(V))*[1]. As(V) will then be transported in the cells by the phosphate transport channels and replace it within the cell. Therefore, it will interfere with biochemical reactions that use phosphate groups like glycolysis and oxidative phosphorylation (which is obviously bad) (Fan et al., 2019). These are steps of cellular respiration which is the process happening in mitochondria during which ADP is transformed into ATP, ATP being the main source of energy for many different activities in organisms. Failure to synthesize ATP is an issue as various processes cannot be carried out without it (Wakim & Grewal).
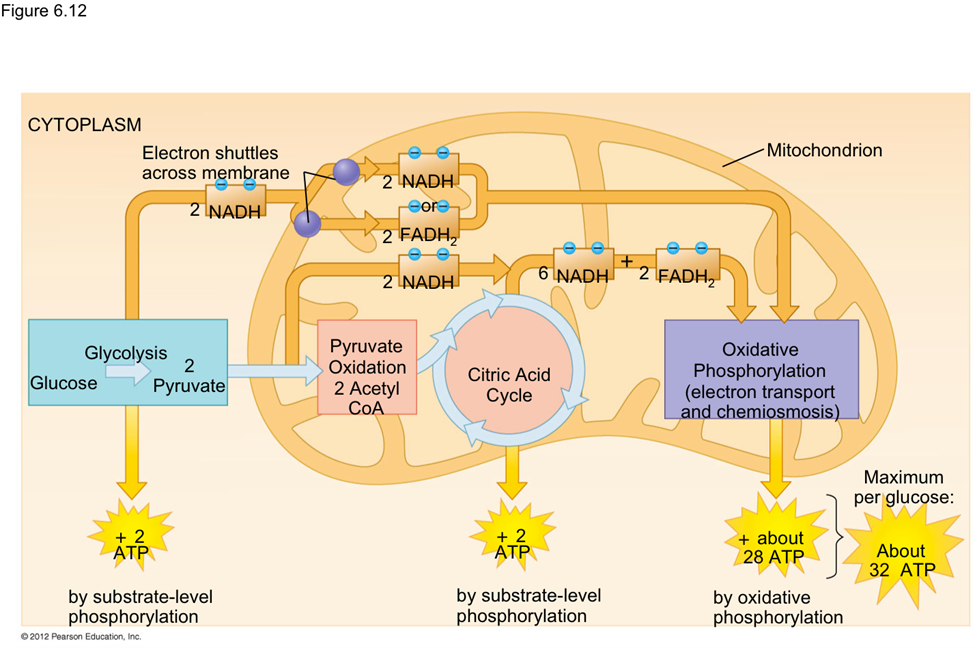
Figure 11: Scheme showing the steps of cellular respiration in mitochondria with the number of ATP molecules synthesized at each step. It is possible to see glycolysis and oxidative phosphorylation which can be impacted by As(V) (Cellular Respiration Steps and Location).
However, when As(V) or As(III)** are adsorbed by NPTiO, the effects will be decreased. In fact, NPTiO is used as a carrier in NP technologies as it adsorbs metals. Although the combination of NPTiO will increase the bioaccumulation of arsenic in Daphnia because of the adsorbent effects, the toxicity of the metal will be alleviated. This is because Daphnia’s gut has a pH of 6.8 to 7.2, which is more acidic than freshwater pH levels. On top of that, a lower pH increases the positive charges of NPTiO which is what happens in Daphnia’s gut. Because of the increasing positive charges of NPTiO, it will bind to more arsenic and therefore, the metal will become less bioavailable as it will not be metabolised by Daphnia. (Fan et al., 2019) On the other hand, silver nanoparticles (AgNP) do not have a positive effect on Daphnia populations. What happens with AgNP is that they can release Ag+ which is toxic to Daphnia. Ag+ can disturb the gill system of Daphnia by taking the place of an endogenous ion. Scientists have not yet understood the process by which the gill system is impacted, but possible hypotheses are that it creates oxidative stress, or it damages proteins as it is not supposed to be there and takes the place of another ion in the metabolism. They may also react with enzymes, and therefore, enzymes would not be able to continue doing their functions as it will be modified by Ag+ (Seitz et al., 2015).
[1] *As(V): Arsenic with an oxidation state of +5
**As(III): Arsenic with an oxidation state of +3
***Ag+: Silver ion (with a plus one charge)
Microplastics
As we know, microplastics (MP) have become an increasing environmental concern in the past few years. Zooplanktons (like Daphnia) are impacted by microplastic pollution. The effects of MP pollution were found to be mortality as well as reduced growth in the young generation and in population in general. What happens with MP is that they can transport different contaminants on their surface and accumulate in organisms and in the food chain as they are consumed by primary consumers like Daphnia. The MP themselves can cause negative effects on the organisms and when they transport toxic chemicals or particles, the effects are even worse. Therefore, MP ingestion reduces Daphnia fitness as it can be seen in Figure 12. In fact, this Daphnia is full of MP, and it stuck in its gills as well.
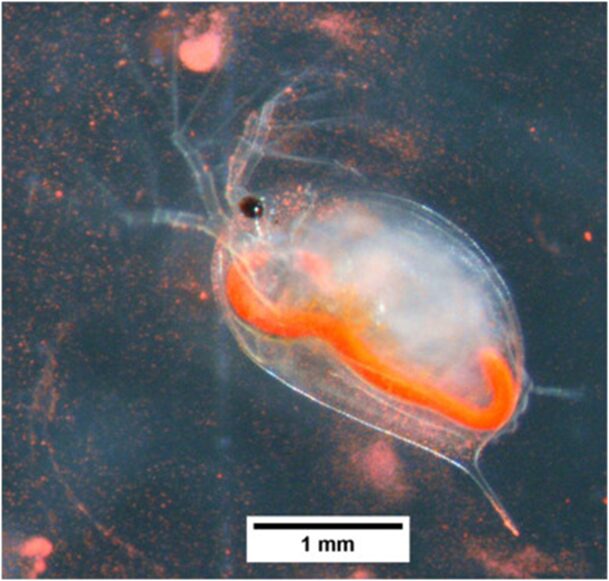
Figure 12: Picture of a Daphnia full of microplastics. It is possible to see that some of its transparency was lost (Guilhermino et al., 2021).
MP contain toxic chemicals that can cause toxicity. For example, bisphenol A (BPA) can have many different effects on Daphnia like death and effects on the endocrine system. Effects of MP on Daphnia are neurotoxicity, oxidative stress, and damage (Guilhermino et al., 2021). Neurotoxicity is done through the inhibition of acetylcholinesterase activity. This enzyme is found at postsynaptic neuromuscular junctions and plays a role in neuronal transmission and signaling. How it does that is by breaking down acetylcholine (a neurotransmitter) after it has transmitted its information to make sure that it doesn’t go around activating nearby receptors (Trang & Khandhar, 2023). If that happens, the neurotransmitters are not controlled and can go around activating other nerves, creating unwanted electrical signals. Therefore, the consequences of MP on Daphnia are both physical and chemicals.
Ecoresponsive genome
As it was talked about in a previous paper, Daphnia possess phenotypic plasticity. This is due to 30,907 genes that it contains. On top of that, Daphnia have quite a unique genome because over 33% of it does not have any homologs in another genus’ genome. Also, Daphnia possess many paralogs that gain different functions quickly after duplication. Indeed, as the genome of D. pulex was examined, many gene with unknown homologs (with other species) were found. Also, these genes (unique to Daphnia) seemed over-represented during transcription, but they varied with ecological conditions. Therefore, gene expression will change depending on the challenges as it can be seen on Figure 13.
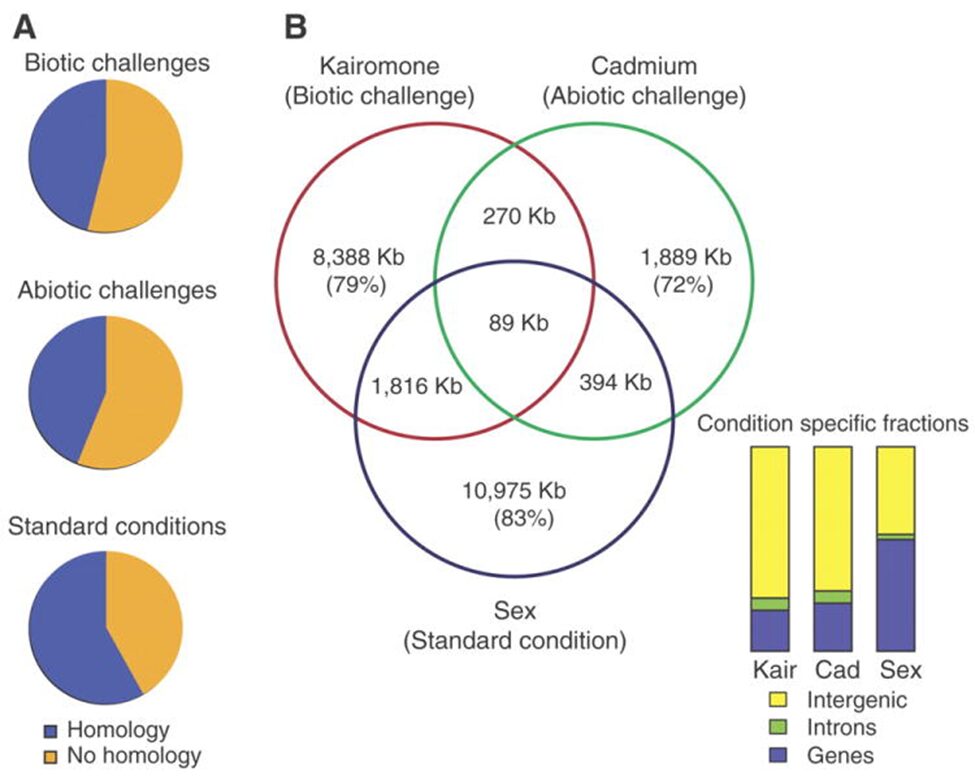
Figure 13: The different challenges that Daphnia face and the type of gene expression (homologous or non-homologous) In the Venn diagram, there are examples of challenges that they can face as well as the response and how similar they are with each other (Colbourne et al., 2011).
This means that the genes expressed depend on the external conditions. On top of that, the percentage of genes unique to Daphnia get higher when they face a challenge (whether it is a biotic or an abiotic challenge). Furthermore, as it was said previously, most of Daphnia’s paralogs will differentiate right after they were duplicated or close to that time. These genes are believed to response to the given environmental conditions. Scientists called that preservation by entertainment. This means that this process increases the chances of preserving a duplicated gene if it is used. This is an evolutionary advantage as they can become more fit to their environment and to the challenges they face. If a paralog is proven to be useless or disadvantageous, it will most likely get lost in the genome. On the other hand, if the gene is useful, it will most likely continue to be expressed (Colbourne et al., 2011).
Effects of Temperature on Daphnia
Inducible Morphological Changes
Temperature changes affect freshwater organisms in many ways such as their physiology, development, and fitness. There are three non-exclusive types of organismal responses to climate change: time of occurrence, space (distribution), and self (changes in organisms’ physiology, not related to spatial or temporal changes) (Dziuba et al., 2020).
In the case of Daphnia, they must deal with temperature changes of around 10°C during their vertical migration to avoid predators. High temperature tolerance is essential for Daphnia’s survival under temperature increase and it is highly dependent on the ability of oxygen transport mechanisms to meet the demand for oxygen in metabolism. Under sudden temperature increases, there is an increasing demand for oxygen, along with less oxygen being available because of the reduced solubility in the water. Since hemoglobin (Hb) is the central element in oxygen transport in the system, an intense synthesis of Hb is therefore needed. The Hb adjustment is not immediate, therefore, a sudden rise in temperature will lead Daphnia into the upper pessimum range that causes the mismatch between the energy need and the oxygen transport that will ultimately cause the transition to anaerobic metabolism and the collapse in physiological functions in Daphnia. On the other hand, when the water temperature is low, the oxygen demand is reduced, followed by the cessation of ventilation and perfusion and the muscular activity needed for the correct supply of oxygen. Consequently, PO2 in the blood diminishes and anaerobic metabolism is activated. Therefore, as can be seen from Figure 14, regardless of populations in different regions, Daphnia individuals are less active and have low survival rates under extremely high and low temperatures. Studies have also shown that Daphnia individuals are more vulnerable to an increase in temperature than a decrease (Müller et al., 2018).
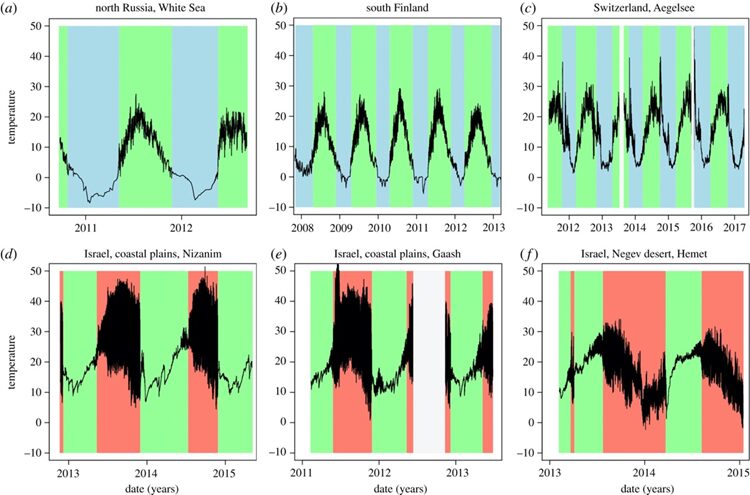
Figure 14. Seasonal temperature dynamics of water bodies from three summer-active northern populations (a–c) and three winter-active southern populations (d–f) of Daphnia. The black line marks temperature recorded in 30 min intervals. Time periods with water conditions suitable for planktonic Daphnia have a green background; time periods with unsuitable conditions have a blue (less than 5°C, northern populations) or a red background (dry, southern populations). Data logger failure (low battery and vandalism) is shown in white (Seefeldt & Ebert, 2019).
The temperature-induced change in physiology can be considered in two aspects: genetic responses and phenotypic responses under predator cues. Daphnia’s metabolic rate increases with the rise in temperature. At higher temperatures, there’s an upregulation of digestive proteases like serine peptidases to assist proteolysis in the digestive system, the process by which peptide bonds in proteins are broken generating free amino acids. This upregulation shows Daphnia’s accommodation of higher feeding rates in response to increased metabolism. At the same time, genes related to visual perception are also upregulated by temperature and the predators’ kairomones. Visual perception is associated with the vertical migration of Daphnia for predator avoidance and since higher temperatures can be regarded as a proxy for shallower depths and therefore higher incoming radiation, the phototransduction genes are enriched for better predator avoidance. (Oliver et al., 2022)
Phenotypic plasticity is the ability of a single genotype to produce multiple phenotypes in response to environmental change to achieve adaptation. Synergistic interactions between temperature and predation (detection of kairomone) for some traits represent an important mechanism for organisms to adapt to the changing environment. Figure 15 illustrates the effects of increasing water temperature on Daphnia. As the water temperature increases in the tolerable range, the predation risks, swimming speed and thus the encounter rate between predator and prey significantly increase. The interaction between temperature and Ca concentration is essential in influencing variation in movement rates of Daphnia. Individuals exposed to high Ca concentration also have high average speed. For example, low temperatures increase intracellular Ca concentrations by preventing calcium from exiting the cell, thus affecting cell excitability. In muscle cells, high intracellular Ca concentration can decrease adenosine triphosphate availability. Both responses can lead to reduced muscle contractions and consequently reduced locomotor ability (Betini et al., 2016). Similar mechanism applies to warmer water conditions. As a result, Daphnia shows a seasonal morphological change in warmer water conditions in summer, including a reduction in the shape and size of the head when the food resources are abundant to create a smaller hydrodynamic disturbance and ultimately decrease encounter rate. At the same time, since high temperature increases metabolic rate, Daphnia also shows a larger reproductive investment and a smaller size at maturity and earlier age of first reproduction (Riessen, 2015).
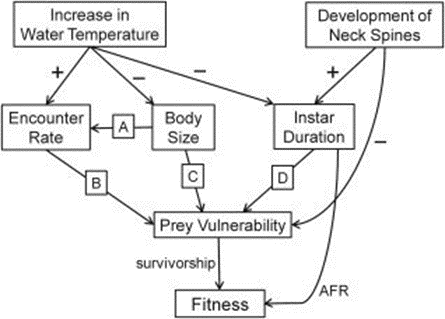
Figure 15: The effects of an increase in water temperature and the development of defensive neck spines on Daphnia fitness. Arrows indicate the direction of impacts (Riessen, 2015).
Effects of Climate change on Daphnia Populations
Temperature change is the main component of climate change, and it may promote the establishment of pre-adapted invaders but may also induce evolutionary adaptations in native populations that increase their resistance to invasion. Considering the effect of temperature rise on Daphnia’s time of occurrence and space (distribution), studies have shown that the structure of zooplankton communities, including the composition of a community, overwintering strategies, and sexual reproduction, may be changed for adaptation. The temperature-induced population composition change can be supported by a study on Daphnia galeata and Daphnia longispina. As can be seen from Figure 16, while D. galeata is more common to be found in warmer water environments and D. longispina is usually in normal water conditions, it is suggested that there is a gene flow between populations in different temperature conditions through short distances and waterways connecting the lakes, allowing Daphnia or their resting eggs to be transported. On the other hand, the competitive exclusion of some Daphnia species in increased temperatures may be averted through hybridization, which could preserve their gene pool for a period of time in lakes undergoing warming. Hybridization could also produce novel genetic variants to enhance the resistance to elevated temperature of the entire community. Consequently, if the temperature of the water environment increases, D. galeata will become dominant through the gene flow and thus the community composition will be reconstructed to adapt to climate change (Dziuba et al., 2020).
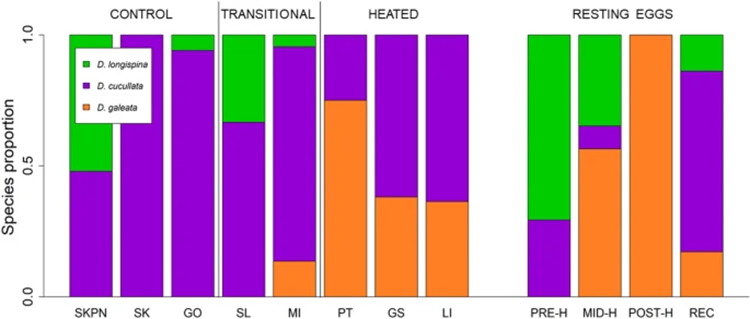
Figure 16: Species composition in the investigated lakes of contemporary samples and resting eggs of Daphnia. Contemporary samples are grouped as follows: three control lakes (SKPN, SK, GO), two transitional heated lakes (SL, MI), and three heated lakes (PT, GS, LI). Resting eggs are grouped as follows: PRE-H—produced before the onset of heating, MID-H—produced after the launch of the first plant but before the launch of the second power plant, POST-H—produced in the ca. 15 years following the launch of the second power plant, REC—produced recently, i.e. within 15 years of core collection (Dziuba et al., 2020).
On the other hand, climate change may also change sexual reproduction mechanism in Daphnia communities, as shown in Figure 17. In warm water environments, high population growth and asexual reproduction can be observed and as the population quickly reaches the carrying capacity, the efficiency of asexual reproduction declines. In extreme cold environment, a shift from asexual to sexual reproduction can be observed and it leads to a higher number of ephippial females, which carry eggs with protective cases and correlate positively with Daphnia’s population size. The protective case is a structure formed from the chitinous carapace with three layers, the external and internal layers of the wall cover cell-like compartments of the middle layer which are made up of multiple polyhedrons similar in structure to a honeycomb, which provides protection for dormant eggs and allows the ephippium to float to the surface when filled with air or sink to the bottom of the reservoir when filled with water. This contributes to the safety of the eggs while providing resistance to extreme temperatures (Bernatowicz et al., 2018). This also indicates an overwintering strategy of D. galeata from production of sexual resting eggs to parthenogenesis. Maintaining parthenogenesis over mild winters provides Daphnia with an advantage over competitors in spring. In an environment subjected to shift between cold and warm conditions, two regimes are included: synchronous (uniform time lags between cold and warm conditions) and stochastic (random changes). In the synchronous regime, Daphnia’s reproduction is initially both asexual and sexual and later sexual reproduction diminished, which suggests that Daphnia communities can detect the timing and duration of favorable temperature conditions to ensure the population vitality. In the stochastic regime, a clear shift between asexual and sexual reproduction can be observed and the population size remains lower than the starting size. Hence, by shifting between sexual and asexual reproduction, Daphnia populations can remain relatively stable in climate and temperature change (Adamczuk, 2020).
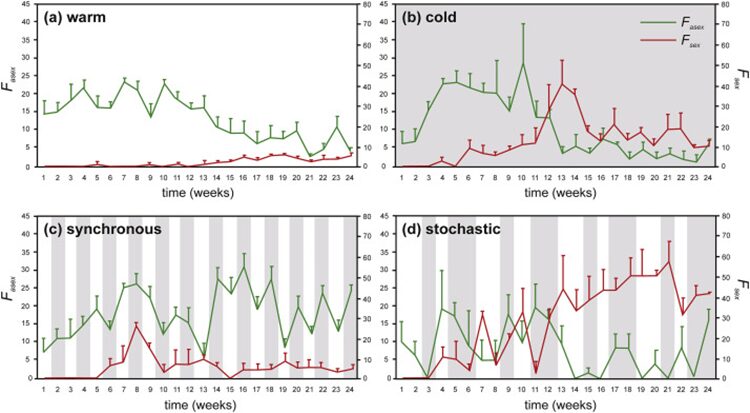
Figure 17: Asexual (Fasex) and sexual (Fsex) reproduction of D. magna under different thermal regimes. Reproductive effort is expressed as percentage quotient of the number of embryo-bearing parthenogenetic females or ephippial females to total number of individuals. Shaded regions indicate cold episodes (Adamczuk, 2020).
Conclusions
Daphnia’s chemical interactions with its environment are numerous. In fact, they interact through kairomones and with each other through various signals; different chemicals and substances can influence them and so does temperature. It is also important to note that the interactions are not only one-sided. Indeed, Daphnia can also do bioremediation, changing the chemical properties of their surroundings. As a longstanding genus, Daphnia has had time to perfect their strategies to have a better chance at surviving life and augmenting their fitness. Facing many conundrums, the Daphnia genus has developed many design solutions to thrive. Firstly, a problem Daphnia has is that it has a very poor vision as explained in our previous paper. Therefore, it has trouble detecting its predators. The solution found to this problem is the use of kairomone detection. This also affects their reproduction and phenotype to ensure that they are the most viable as individuals with anatomical plasticity but also with the use of sexual reproduction to diversify the population genetic diversity. Also, they will undergo diel vertical migration to make sure that they do not encounter predators. Furthermore, kairomone detection is tuned enough to be concentration dependent as Daphnia will exhibit different behavior and morphological changes depending on the concentration of kairomone in their environment. Another conundrum would be that in the environment, some metals naturally end up in the water. Because these are often toxic, Daphnia needed to find a solution to stay fit even though these can be present. This is why they developed metallothionein genes to be able to synthesize MT to make metal ions unavailable biologically. This seems to start at a certain threshold and has transgenerational effects so that the offspring of a parent living in an environment with a high metal concentration are acclimated to it when they are born. Also, with constantly changing environmental conditions, species need to adapt. Therefore, Daphnia developed an ecoresponsive genome with a high paralog number that undergoes preservation by entertainment. Due to the risk of predation, Daphnia must undergo DVM. Because they change their depth in the water, temperature and resources change as well. Therefore, Daphnia developed mechanisms to gain a high temperature tolerance as well as a metabolism adapted to these frequent changes. On top of that, because temperature changes, Daphnia switch between sexual and asexual reproduction to ensure the preservation of their population. To conclude, an important takeaway of this essay is that some chemicals, contaminants and temperature changes are just too much for Daphnia to adapt and result in the death of the organisms. This is because those are due to human activity. In fact, Daphnia are acclimated to their usual environmental conditions because we all know how long it takes for natural selection to do its job. However, because of human activity, things are changing too fast for them to adapt. With NPTiO, it is only because of luck that it reduces the impact of arsenic on them. However, most of the contaminants caused by humans are lethal to them. This also proves that the design solutions are due to natural solution and that they all serve a purpose as they have found a solution to everything except the very recent conundrum: human activity. Developing new design solutions to new problems takes time and could also be useless in such a fast-changing world.
REFERENCES
Abdullahi, M., Li, X., Abdallah, M. A., Stubbings, W., Yan, N., Barnard, M., Guo, L. H., Colbourne, J. K., & Orsini, L. (2022). Daphnia as a Sentinel Species for Environmental Health Protection: A Perspective on Biomonitoring and Bioremediation of Chemical Pollution. Environ Sci Technol, 56(20), 14237-14248. https://doi.org/10.1021/acs.est.2c01799
Adamczuk, M. (2020). Population dynamics and life history traits of Daphnia magna across thermal regimes of environments. Science of The Total Environment, 723, 137963. https://doi.org/https://doi.org/10.1016/j.scitotenv.2020.137963
Arao, T., Kato, Y., Nong, Q. D., Yamamoto, H., Watanabe, H., Matsuura, T., Tatarazako, N., Tani, K., Okamoto, A., Matsumoto, T., & Watanabe, H. (2020). Production of genome-edited Daphnia for heavy metal detection by fluorescence. Scientific Reports, 10(1), 21490. https://doi.org/10.1038/s41598-020-78572-z
Asselman, J., Glaholt, S. P., Smith, Z., Smagghe, G., Janssen, C. R., Colbourne, J. K., Shaw, J. R., & De Schamphelaere, K. A. C. (2012). Functional characterization of four metallothionein genes in Daphnia pulex exposed to environmental stressors. Aquatic Toxicology, 110-111, 54-65. https://doi.org/https://doi.org/10.1016/j.aquatox.2011.12.010
Bernatowicz, P., Radzikowski, J., Paterczyk, B., Bebas, P., & Slusarczyk, M. (2018). Internal structure of Daphnia ephippium as an adaptation to dispersion. Zoologischer Anzeiger, 277, 12-22. https://doi.org/https://doi.org/10.1016/j.jcz.2018.06.006
Betini, G. S., Roszell, J., Heyland, A., & Fryxell, J. M. (2016). Calcium interacts with temperature to influence Daphnia movement rates. R Soc Open Sci, 3(12), 160537. https://doi.org/10.1098/rsos.160537
Campbell, D. (2021). Pollution disrupts water fleas’ ‘chemical conversations’, distrupts food chain: U of T study. University of Toronto. Retrieved November 5 from https://www.utoronto.ca/news/pollution-disrupts-water-fleas-chemical-conversations-disrupts-food-chain-u-t-study
Cellular Respiration Steps and Location. Animal Wallpaper I Love Wallpaper. Retrieved November 13 from https://awesomeanimewallpaperhd.pages.dev/cellular-respiration-steps-and-location
Colbourne, J. K., Pfrender, M. E., Gilbert, D., Thomas, W. K., Tucker, A., Oakley, T. H., Tokishita, S., Aerts, A., Arnold, G. J., Basu, M. K., Bauer, D. J., Cáceres, C. E., Carmel, L., Casola, C., Choi, J. H., Detter, J. C., Dong, Q., Dusheyko, S., Eads, B. D., . . . Boore, J. L. (2011). The ecoresponsive genome of Daphnia pulex. Science, 331(6017), 555-561. https://doi.org/10.1126/science.1197761
De Schamphelaere, K. A. C., & Janssen, C. R. (2004). Effects of chronic dietary copper exposure on growth and reproduction of Daphnia magna. Environmental Toxicology and Chemistry, 23(8), 2038-2047. https://doi.org/https://doi.org/10.1897/03-411
Dearn, K., & Orsini, L. (2021). Using Daphnia for bioremediation WO2021116229).
Dobson, P., Jarvie, H., & King, S. (2010). Nanoparticles. Britannica. Retrieved November 13 from https://www.britannica.com/science/nanoparticle#ref288108
Dziegiel, P., Pula, B., Kobierzycki, C., Stasiolek, M., & Podhorska-Okolow, M. (2016). Metallothioneins: Structure and Functions. In Metallothioneins in Normal and Cancer Cells (pp. 3-20). Springer International Publishing. https://doi.org/10.1007/978-3-319-27472-0_2
Dziuba, M. K., Herdegen-Radwan, M., Pluta, E., Wejnerowski, Ł., Szczuciński, W., & Cerbin, S. (2020). Temperature increase altered Daphnia community structure in artificially heated lakes: a potential scenario for a warmer future. Scientific Reports, 10(1), 13956. https://doi.org/10.1038/s41598-020-70294-6
Egan, N., Stinson, S. A., Deng, X., Lawler, S. P., & Connon, R. E. (2023). Swimming Behavior of Daphnia magna Is Altered by Pesticides of Concern, as Components of Agricultural Surface Water and in Acute Exposures. Biology (Basel), 12(3). https://doi.org/10.3390/biology12030425
Fan, W., Liang, D., Wang, X., Ren, J., Xiao, S., & Zhou, T. (2019). Two-generational effects and recovery of arsenic and arsenate on Daphnia magna in the presence of nano-TiO2. Ecotoxicology and Environmental Safety, 172, 136-143. https://doi.org/https://doi.org/10.1016/j.ecoenv.2019.01.072
Guilhermino, L., Martins, A., Cunha, S., & Fernandes, J. O. (2021). Long-term adverse effects of microplastics on Daphnia magna reproduction and population growth rate at increased water temperature and light intensity: Combined effects of stressors and interactions. Science of The Total Environment, 784, 147082. https://doi.org/https://doi.org/10.1016/j.scitotenv.2021.147082
Huo, Y. Z., He, W. H., Luo, K., Wang, Y. Y., Zhang, Y. J., Tian, Q. T., & He, P. M. (2010). [Bioremediation efficiency of applying Daphnia magna and submerged plants: a case study in Dishui Lake of Shanghai, China]. Ying Yong Sheng Tai Xue Bao, 21(2), 495-499.
Kimberly, D. A., & Salice, C. J. (2015). Multigenerational contaminant exposures produce non-monotonic, transgenerational responses in Daphnia magna. Environmental Pollution, 207, 176-182. https://doi.org/https://doi.org/10.1016/j.envpol.2015.09.020
Mikhaltsov, A. (2016). Дафния в тёмном поле. Wikimedia Commons. Retrieved November 7 from https://commons.wikimedia.org/wiki/File:%D0%94%D0%B0%D1%84%D0%BD%D0%B8%D1%8F_%D0%B2_%D1%82%D1%91%D0%BC%D0%BD%D0%BE%D0%BC_%D0%BF%D0%BE%D0%BB%D0%B5.jpg
Mitchell, C. (2022). What Is Bioremediation, and How Does It Work (With Examples)? Investopedia. Retrieved November 5 from https://www.investopedia.com/terms/b/bioremediation.asp
Miyakawa, H., Sato, T., Song, Y., Tollefsen, K. E., & Iguchi, T. (2018). Ecdysteroid and juvenile hormone biosynthesis, receptors and their signaling in the freshwater microcrustacean Daphnia. The Journal of Steroid Biochemistry and Molecular Biology, 184, 62-68. https://doi.org/https://doi.org/10.1016/j.jsbmb.2017.12.006
Montenegro, J. D. (2022). Gene Co-expression Network Analysis. In D. Edwards (Ed.), Plant Bioinformatics: Methods and Protocols (pp. 387-404). Springer US. https://doi.org/10.1007/978-1-0716-2067-0_19
Müller, M. F., Colomer, J., & Serra, T. (2018). Temperature-driven response reversibility and short-term quasi-acclimation of Daphnia magna. PLoS One, 13(12), e0209705. https://doi.org/10.1371/journal.pone.0209705
Oliveira Pereira, E. A., Labine, L. M., Kleywegt, S., Jobst, K. J., Simpson, A. J., & Simpson, M. J. (2023). Daphnia magna sub-lethal exposure to phthalate pollutants elicits disruptions in amino acid and energy metabolism. Aquatic Toxicology, 257, 106432. https://doi.org/https://doi.org/10.1016/j.aquatox.2023.106432
Oliver, A., Cavalheri, H. B., Lima, T. G., Jones, N. T., Podell, S., Zarate, D., Allen, E., Burton, R. S., & Shurin, J. B. (2022). Phenotypic and transcriptional response of Daphnia pulicaria to the combined effects of temperature and predation. PLoS One, 17(7), e0265103. https://doi.org/10.1371/journal.pone.0265103
Otte, K. A., Fröhlich, T., Arnold, G. J., & Laforsch, C. (2014). Proteomic analysis of Daphnia magna hints at molecular pathways involved in defensive plastic responses. BMC Genomics, 15, 306. https://doi.org/10.1186/1471-2164-15-306
Pereira, C. M. S., Blust, R., & De Schamphelaere, K. A. C. (2019). Effect of temperature on nickel uptake and elimination in Daphnia magna. Environmental Toxicology and Chemistry, 38(4), 784-793. https://doi.org/https://doi.org/10.1002/etc.4352
Pijanowska, J., & Kowalczewski, A. (1997). Predators can induce swarming behaviour and locomotory responses in Daphnia. Freshwater Biology, 37(3), 649-656. https://doi.org/https://doi.org/10.1046/j.1365-2427.1997.00192.x
Ren, Z., Li, Z., Ma, M., Wang, Z., & Fu, R. (2009). Behavioral Responses of Daphnia Magna to Stresses of Chemicals with Different Toxic Characteristics. Bulletin of Environmental Contamination and Toxicology, 82(3), 310-316. https://doi.org/10.1007/s00128-008-9588-1
Riessen, H. (2015). Water temperature alters predation risk and the adaptive landscape of induced defenses in plankton communities. Limnology and Oceanography, 60. https://doi.org/10.1002/lno.10150
Seefeldt, L., & Ebert, D. (2019). Temperature- versus precipitation-limitation shape local temperature tolerance in a Holarctic freshwater crustacean. Proceedings of the Royal Society B: Biological Sciences, 286(1907), 20190929. https://doi.org/doi:10.1098/rspb.2019.0929
Seitz, F., Rosenfeldt, R. R., Storm, K., Metreveli, G., Schaumann, G. E., Schulz, R., & Bundschuh, M. (2015). Effects of silver nanoparticle properties, media pH and dissolved organic matter on toxicity to Daphnia magna. Ecotoxicology and Environmental Safety, 111, 263-270. https://doi.org/https://doi.org/10.1016/j.ecoenv.2014.09.031
Stevčić, Č., Pulkkinen, K., & Pirhonen, J. (2020). Efficiency of Daphnia magna in removal of green microalgae cultivated in Nordic recirculating aquaculture system wastewater. Algal Research, 52, 102108. https://doi.org/https://doi.org/10.1016/j.algal.2020.102108
Tkaczyk, A., Bownik, A., Dudka, J., Kowal, K., & Ślaska, B. (2021). Daphnia magna model in the toxicity assessment of pharmaceuticals: A review. Science of The Total Environment, 763, 143038. https://doi.org/https://doi.org/10.1016/j.scitotenv.2020.143038
Trang, A., & Khandhar, P. B. (2023). Physiology, Acetylcholinesterase. National Library of Medicine. Retrieved November 5 from https://www.ncbi.nlm.nih.gov/books/NBK539735/
Van Gool, E., & Ringelberg, J. (2002). Relationship between fish kairomone concentration in a lake and phototactic swimming by Daphnia. Journal of Plankton Research, 24(7), 713-721. https://doi.org/10.1093/plankt/24.7.713
Wakim, S., & Grewal, M. Cellular Respiration. Libre Texts Biology. Retrieved November 13 from https://bio.libretexts.org/Bookshelves/Human_Biology/Human_Biology_(Wakim_and_Grewal)/05%3A_Cells/5.09%3A_Cellular_Respiration
Water fleas as ‘canaries in a coal mine’ offer key to managing chemical pollution. (2022). Science Daily. Retrieved November 5 from https://www.sciencedaily.com/releases/2022/09/220928113004.htm
Weiss, L. C. (2019). Sensory Ecology of Predator-Induced Phenotypic Plasticity [Review]. Frontiers in Behavioral Neuroscience, 12. https://doi.org/10.3389/fnbeh.2018.00330
Weiss, L. C., Leimann, J., & Tollrian, R. (2015). Predator-induced defences in Daphnia longicephala: location of kairomone receptors and timeline of sensitive phases to trait formation. J Exp Biol, 218(Pt 18), 2918-2926. https://doi.org/10.1242/jeb.124552
Yin, J., Long, Y., Xiao, W., Liu, D., Tian, Q., Li, Y., Liu, C., Chen, L., & Pan, Y. (2023). Ecotoxicology of microplastics in Daphnia: A review focusing on microplastic properties and multiscale attributes of Daphnia. Ecotoxicology and Environmental Safety, 249, 114433. https://doi.org/https://doi.org/10.1016/j.ecoenv.2022.114433
Yuan, W., Zhou, Y., Chen, Y., Liu, X., & Wang, J. (2020). Toxicological effects of microplastics and heavy metals on the Daphnia magna. Science of The Total Environment, 746, 141254. https://doi.org/https://doi.org/10.1016/j.scitotenv.2020.141254