Abstract
Skin, a keratin-based tissue, is the largest organ in humans. This essay examines the molecular structural function of keratins, their evolutionary origins, and functions in scales and scale-derived structures. The organisms examined are pangolins, reptiles, and birds. The hierarchical structure of keratin proteins is described for alpha and beta keratins, which polymerize to form tough protective structures stabilized by disulfide bridging, coiled-coil tertiary structures, and hydrophobic interactions that make them ideal for protecting the integumentum of animals. The unique triple layered structure of pangolin keratinization is described along with the structure’s water-based repair behavior. Reptile scales are made of alpha and beta keratins in the epidermis, giving them improved rigidity, and their composition and resultant properties vary between species. Various models describe the beta-keratin structure of feathers, which are hypothesized to be derived from reptilian scales. Finally, different biomimetic applications for each of these complex keratin-based structures are discussed in the context of engineering design.
Introduction
An animal’s evolutionary fitness is based on its ability to pass on genetic material. To do this, a creature must survive until sexual maturity. To ensure survival, animals must avoid predation and survive the dangers of their environment. The external layers of an animal are critical for maintaining the homeostatic conditions required for life and protecting it from the environment and its predators. The top layer of the epidermis, also known as the stratum corneum, coats a majority of an animal’s body, thus protecting the animal. This external layer of skin is primarily composed of basal keratinocyte cells (Gilbert, 2000). These cells produce keratin proteins throughout their lifespan until they reach the stratum corneum, where they fully keratinize and die (Gilbert, 2000). This gives them improved strength and toughness. Keratin is not a single unique protein; it is a family with different varieties based on its chemical properties and uses. It is also a key component of intermediate filaments in cells, where it improves structural strength.
The scales and feathers of most land vertebrates are keratin-based and formed through the epidermis through different processes. Pangolins, lizards, and birds use keratin-based scales or feathers as part of their epidermis; however, they evolved these scales in many different environments. The exact structure of these scales differs between these groups, but keratin proteins are integral for all of these scales. This paper examines the environmental-evolutionary relationship that led to the development of animals’ unique keratin usages.
Chemical Structure of Keratin
Keratin is a family of fibrous structural proteins that are all highly insoluble in water (Zhang & Fan, 2021). This prevents them from being dissolved by water which is important for ensuring their long-term stability on the outside of animals and allowing them to aid their role of making the epidermis water resistant. It also allows them to self-assemble more easily in the aqueous solutions in which life occurs. Keratins can be divided into groups based on their primary properties. Alpha-keratins are dominated by alpha-helical secondary structures and are common in all animals, while beta-keratins are exclusive to reptiles and birds and contain many beta sheets. Type I keratins are acidic and tend to be smaller, while type II keratins are larger and have basic charges (Albers, 1996).
Alpha-keratin shows complex hierarchical structure domination by helical structures. There are three zones in alpha-keratins fibrils and the two termination domains. This is shown in Figure 1, with the terminal domains represented by N and C with the fibral domain in the middle.
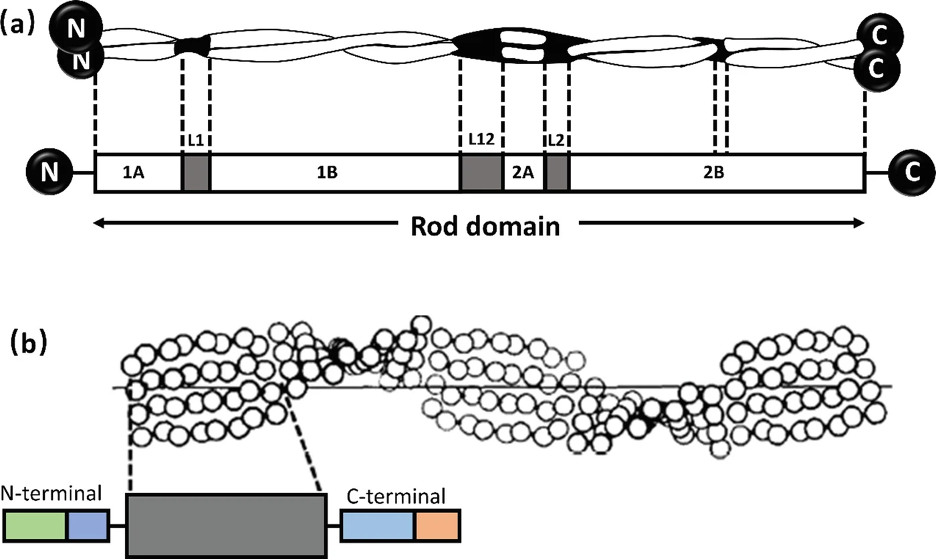
Fig. 1 (a) Genetic representation of the secondary structure of keratin; (b) Molecular view of the fibril portion of keratin (Zhang & Fan, 2021).
The fibrils consist of long, highly conserved repeating stretches of polypeptides with repetition every four amino acids. This allows the formation of alpha-helices (Albers, 1996). These are stabilized with hydrogen bonds between the amino group and carboxyl groups of the peptide backbone. Bonds are formed between an amino acid and the acid 4 places upstream of the initial place. The hydrogen bonding of alpha helices is shown in Figure 2.
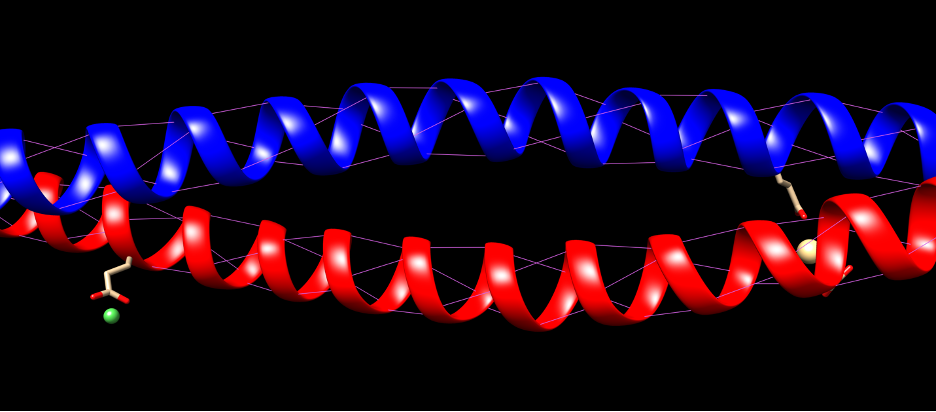
Fig. 2 Hydrogen bonding of KRT1 and KRT10. Purple indicates hydrogen bonds.
This creates a stable and strong structure that can be used as the foundation for many structural proteins. The termination domains are disordered regions used for bonding with other proteins or structures, allowing polymerization to occur effectively (Albers, 1996).
Alpha-helices are the most common secondary structure in proteins, so their presence is not solely responsible for the unique strength of keratins. Keratins form coiled structures with one alpha-helix wrapped around another, thereby increasing their stability and strength. Alpha-keratins are obligatory heteropolymers because they require type I and type II keratins in a 1:1 molar ratio to form this secondary structure (Albers, 1996). This results in most keratins evolving in pairs with the same pair of type I and II conserved across many cell types (Albers, 1996). Ribbon and space-filling diagrams of this are shown in Figures 3 and 4.
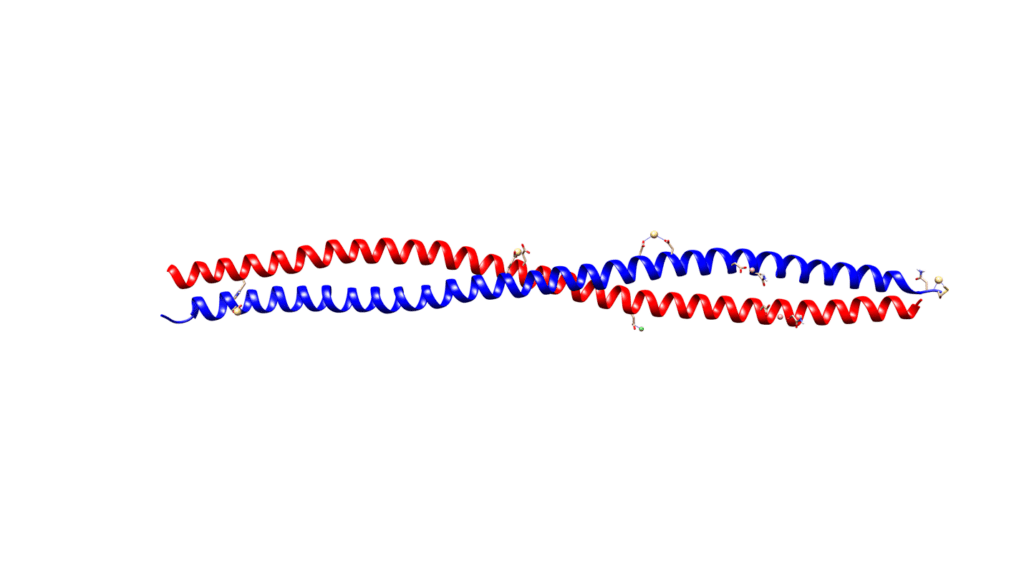
Fig. 3 Ribbon diagram of KRT1 forming a complex with KRT10 created using UCSF Chimera
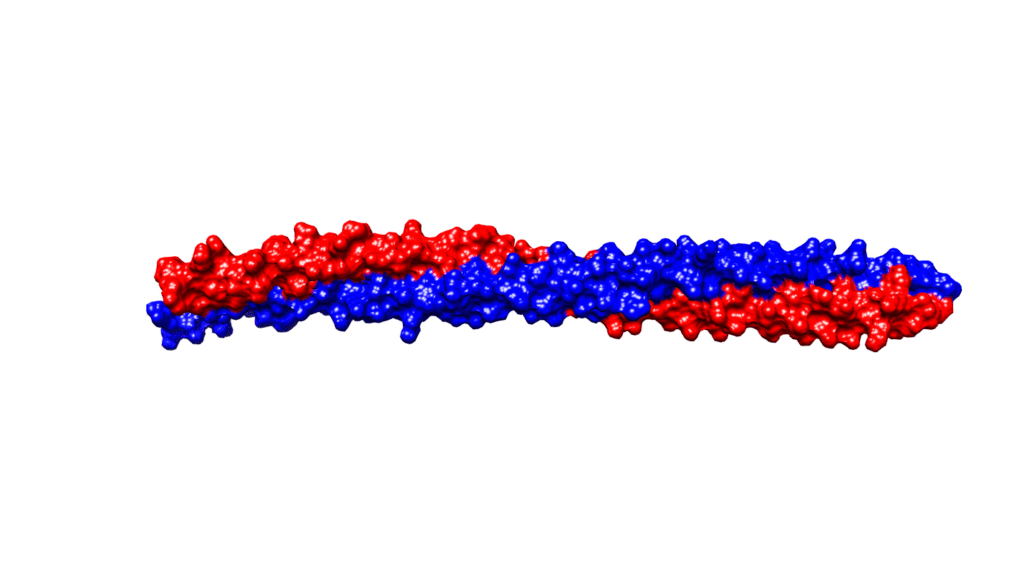
Fig. 4 Space diagram of KRT1 forming a complex with KRT10 created using UCSF Chimera
This structure is further strengthened with the repetition of non-polar amino acids every four nucleotides (Albers, 1996). This creates an internal layer of hydrophobic residues that improve stability and strength. This is illustrated in Figure 5.

Fig 5. Hydrophobicity of KRT1 and KRT10 created using UCSF Chimera. Pink indicates hydrophobic amino acids.
Alpha-keratins can also be hard or soft, depending on Sulphur content. This allows keratin properties to be adjusted for specific purposes within an organism by modifying sulfur content. The keratin found on the external layer of the epidermis is considered soft and tends to have more flexibility than hard keratin (Zhang & Fan, 2021). Hard keratins are present in the fingernails, claws, and horns of mammals and have a much higher ratio of cysteine residues, meaning they have higher sulfur content (Zhang & Fan, 2021). Cysteine is an interesting amino acid, because it contains a thiol group that can be used for forming di-sulfide bridges. These bridges allow the type I and II keratins to bond even more tightly, increasing the overall strength of the structures they form; however, this takes away a significant amount of the protein’s flexibility.
Beta-keratin is most common in birds and reptiles; this makes sense because birds evolved from reptiles over 66 million years ago (Change et al., 2009). Beta keratin is harder and less flexible than alpha-keratin because it has a secondary structure mainly comprising beta sheets. A beta sheet is the second most common structural motif in the secondary structure of proteins, where parallel rows of amino acids bind to the next row using hydrogen bonds. An example of a beta sheet is shown in region F of Figure 6. These proteins are much shorter than the long fibril proteins created using alpha helices, so they are stacked on top of each other to produce larger structures (Alibradi, 2016). This results in unique gene structures for these proteins. In the keratinocytes that produce large amounts of keratins, many beta-keratins must be synthesized to allow the polymerization processes to occur. As a result, there tend to be several genes encoding the same protein clustered together on chromosomes, as shown in part c of Figure 6. Figure 6b shows how many copies of the beta-keratin mRNA can be created from one region of a chromosome, resulting in high protein concentration. Additionally, 6E highlights the similarities of alpha and beta keratin structure with long disordered regions at the end allowing them to polymerize more effectively while the highly ordered middle regions provide the protein with its physical properties.
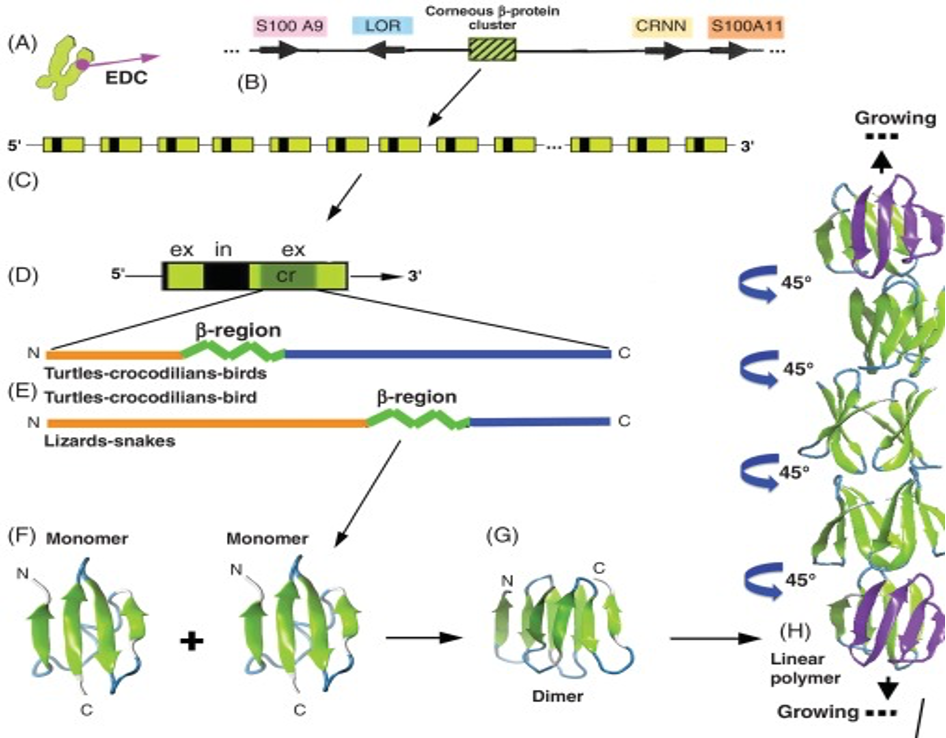
Fig. 6 A diagram of the chromosomal placement of beta-keratin genes and their polymerization process (Alibardi, 2016)
Similarly to alpha-keratins, beta-keratins contain disordered regions at their ends to allow them to bond to other proteins when forming structures. This region varies heavily between different species as the structures being formed have different purposes and require different properties from their constituent proteins. Throughout the rest of this paper, the role of keratins in the structure of pangolin, reptile, and bird scales will be examined with a focus on each animal’s unique lifestyles and how they have altered the keratin they use.
Pangolin scales
Pangolins are mammals that live in Africa and Asia (see Figure 7). They are the most trafficked mammal in the world because of their meat and the use of their scales in traditional Chinese medicine, even though their scales do not have any proven medicinal value (Choo et al., 2016; Jin et al., 2020). The eight species of pangolins that exist can be subdivided into two categories; ground pangolins, like the Chinese pangolin, that sleep in holes they created in the soil, and arboreal pangolins, like the African tree pangolin, that sleep inside trees (Wang et al., 2016).
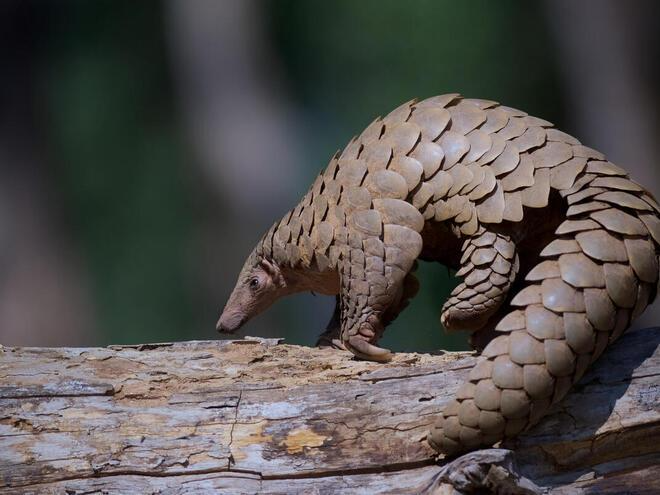
Fig. 7 A pangolin (World Wildlife Fund, n.d.)
These placental mammals are almost completely covered in scales that range in size from 4 to 6 centimeters (Zhou et al., 2020). The underside of their legs, stomach, and feet are the only parts not containing scales. Their scales, along with their skin, accounts for 25% of their weight (Wang et al., 2016). Different pangolin species have scales of different sizes and slightly different shapes. Also, scales do not overlap in the same proportion from species to species, but nevertheless the disposition of scales is similar with all pangolins. Scales in pangolins are arranged in a hexagonal pattern and in such a way that each scale is covered by three scales and covers three other scales. The upper area of each scale is embedded in the skin (Wang, 2016). Figure 8 shows the scale disposition for two species of pangolins.

Fig. 8 (A) shows scales on a Chinese pangolin. (B) shows scales on an African tree pangolin. In both (A) and (B), the black outline demonstrates the part of the scale that is connected to the skin, the yellow line delimits the part of the scale that is covered by other adjacent scales and the part of the scale that is exposed. The blue dotted lines outline the part of the scale that covers the adjacent scales. The green dotted lines show the part of the scale that overlaps with the scale directly beneath (Adapted from Wang, 2016).
This specific scale arrangement ensures that the body of this nocturnal mammal is completely covered by scales that overlap for protection from their environment and predators, even when the animal is moving or curling up (Wang, 2016). In Figure 8, it can be seen that the proportion of overlapping in scales on African tree pangolins is greater than the overlapping in Chinese pangolins. In fact, there is a 19% overlap in the external part of the scales, and 30% overlap for the internal part for Chinese pangolins. For African tree pangolins, the external surfaces overlap by 43% and the internal by 70% (Wang, 2016; Wang & Sullivan, 2017).
The pangolin scales are made of ridges containing 2 to 5 layers of flattened keratinized cells (Wang & Sullivan, 2017). These layers are called the cuticle layer, which is made of keratinized cells and protects the inside of the scale. Pangolin scales are composed of 18 amino acids and are made of both alpha- and beta-keratin (Tong et al., 1995). The proportion of amino acids in pangolin scales can be visualized in Figure 9.

Fig. 9 The percentage of the weight of each amino acid present in pangolin scales. The percentage of each amino acid of the keratin in bird feathers of another edentulous vertebrate, which will be discussed in a later section, is also shown (Adapted from Tong et al., 1995).
The inside of the scale is composed of three layers: dorsal, middle, and ventral (Wang, 2016). Although the percentages might change depending on where the measurements are taken on the scale, the outermost layer, called the dorsal layer, makes up 8% of the scale. The intermediate layer makes up 54% of the scale, and the ventral layer constitutes 38% (Liu et al., 2016b). The difference between the three layers is the orientation of the lamellae, as can be seen in Figure 10. A lamella is a layer of flattened keratinized cells. The cells in the dorsal and ventral layers are flattened and the lamellae are parallel to the surface of the scale. Oppositely, the lamellae in the middle region are angled at about 45° but become almost parallel near the ventral layer (see Figure 10) (Wang, 2016; Wang & Sullivan, 2017; Liu et al., 2016b). Also, the lamellae are crossed, which makes the fibers cross between lamellae. The internal elements have different directions, which means that they are anisotropic. This improves the isotropy of the scale structure; the interior material of the scale is not uniform anymore. Therefore, if any damage is done to the scale by a predator, it would be harder for the fissure to travel down many lamellae (Wang & Sullivan, 2017).
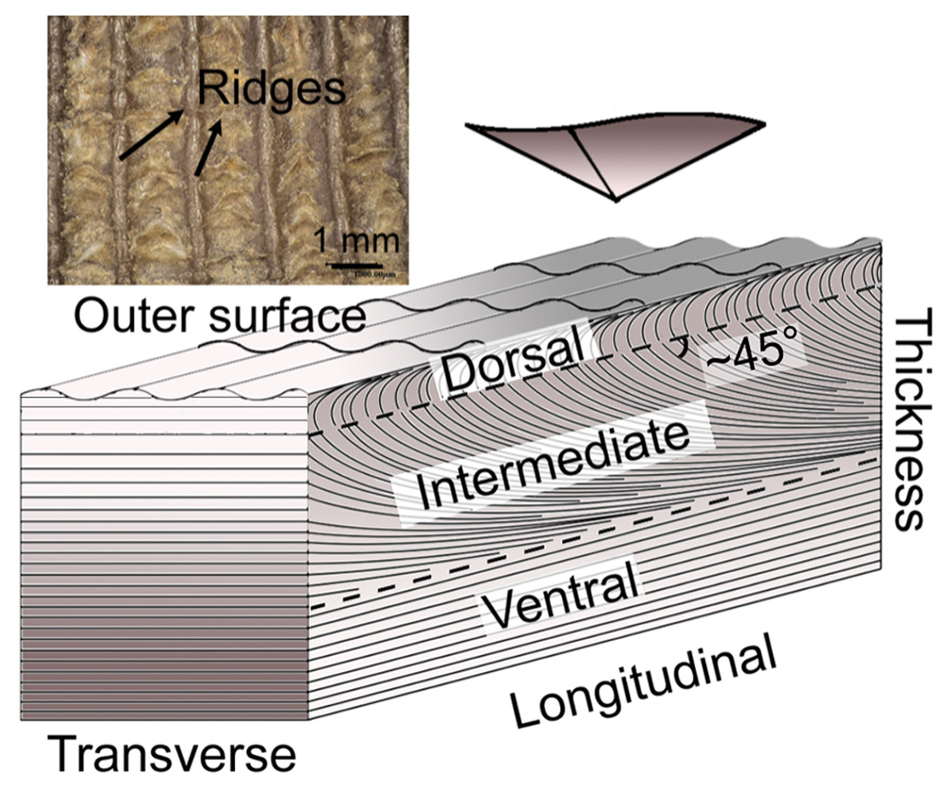
Fig. 10 Dorsal, intermediate and ventral layers are shown, as well as the ridges on the outer surface (adapted from Liu et al., 2016b)
Ground pangolins dig burrows in soil. The ridges and grooves help to drain dirt down their scales. Ridges are separated by a distance of 0.7 millimeters and are aligned parallel to their growth direction (Zhou et al., 2020). It is also important to note that ridges help with wear resistance and the overall structural strength of the scale (Liu et al., 2016b; Zhou et al., 2020). When pangolins dig burrows, their scales are subject to a continuous motion that wears down their scales. This leads their scales to be subject to penetration and bending damage. Their scales, therefore, have wear-resistance and anti-adhesion properties both due to their ridges (Liu et al., 2016a).
When a pangolin is attacked by a predator, it curls up and the sharp edges of the scales facing out offer protection, as seen in Figure 11. The scales are therefore a primary means of protection against predators, and the overlapping of scales ensures that all of the pangolin’s body is covered when sleeping or curling up.
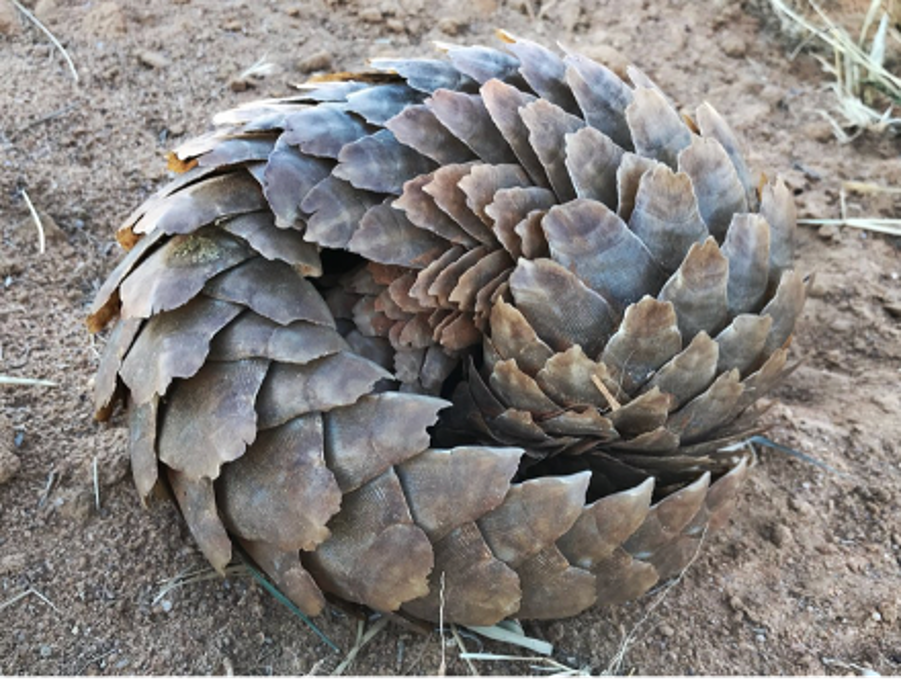
Fig. 11 When a pangolin curls up, its scales serve as protection (“What is a Pangolin”, n.d.).
Similar to hair, pangolin scales keep growing throughout their life, but are trimmed down when these mammals rub against soil and rocks when burrowing (Liu et al., 2016b). Moreover, pangolin scales do not molt. Another method must be employed to fix and regenerate pangolin scales that are damaged by predators or damage done when burrowing. Indeed, hydration can heal damaged pangolin scales. If an indentation is made due to penetration by a predator, hydration will, after 30 hours, regenerate the scale (Liu et al., 2016a). Figure 12 shows an indentation in a pangolin scale diminishing over time after being submerged in water.
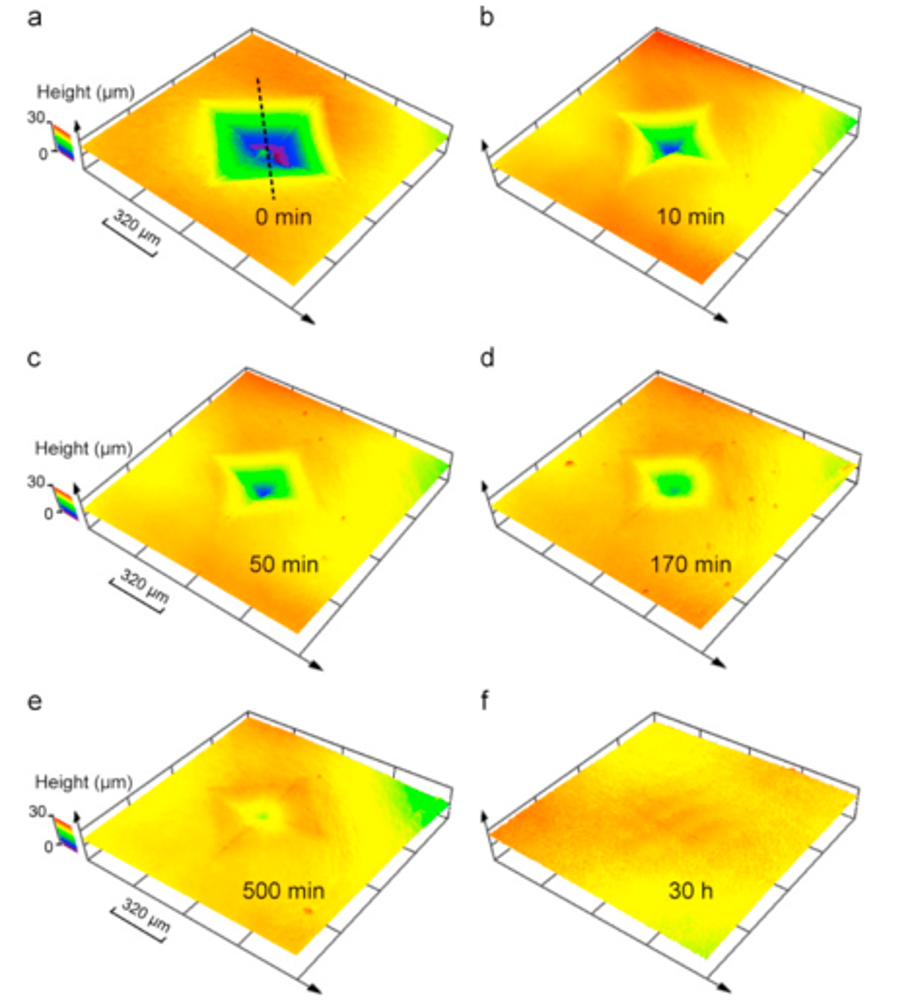
Fig. 12 Morphology of a pangolin scale at various times after it has been in water after an indentation (Liu et al., 2016a)
When the scales are submerged in water, H2O molecules enter the scale, interact with proteins by creating H-bonds with them, as seen in figure 13. Water is a plasticizer, meaning it makes the inside of the scale softer and easier to mold. Water therefore increases the volume of the scale and helps the scale to go back to its original state (Zhou et al., 2020).
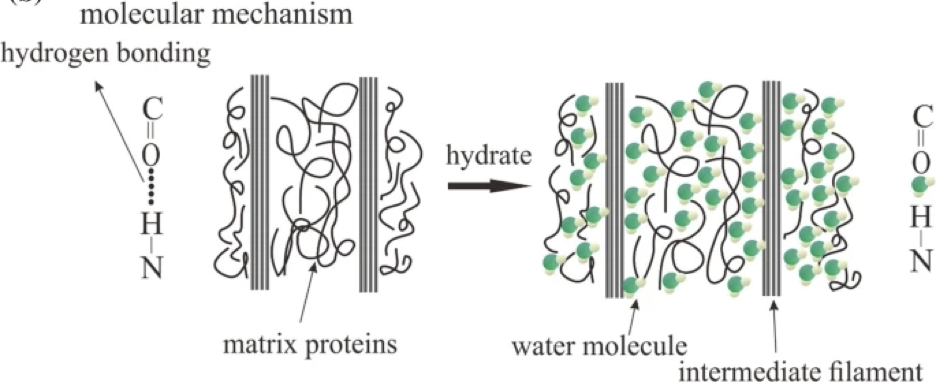
Fig. 13 Water molecules interact with matrix proteins (adapted from Zhou et al., 2020)
On another note, the evolution of scales in pangolins can be explained by looking at their genes. Pangolins have less interferon genes than other mammals – genes which help with infection, inflammation, and skin healing. For instance, only two families of interferon genes are found in the Chinese pangolins, whereas three families are found in the Malayan pangolins. The lack of varieties of interferon genes generates a weaker immunity provided by the skin. Moreover, in other placental animals, there is an interferon epsilon gene IFNE present mainly in skin epithelial cells, which are cells located at the surface of the skin and serve as a barrier to protect the inside cells. The IFNE gene therefore protects cells from any pathogens, similar to a barrier. However, the IFNE gene is absent in Asian and African pangolins because of pseudogenization (Choo et al., 2016). Pseudogenization happens due to evolution and is due to a gene loss by a change in the regulatory elements or coding region of a protein. A pseudogene is thus inactive. This leads to gene loss and loss of function. Gene loss is a pivotal aspect in evolution (Grus & Zhang, 2008). In the case of the pangolin, a mutation produces a premature stop codon. This means that the protein expressed by the IFNE gene is truncated. This leads to many crucial parts being deleted in the IFNE protein. This protein therefore loses its intended function of resisting infection. Pangolin scales are thus an innovation due to the lack of immunity provided by their few interferons and the pseudogene IFNE. The role that the scales play in defense helps shield the pangolin from infections, injuries, and invasion of pathogens (Choo et al., 2016).
As mentioned previously, pangolin scales possess anti-adhesion and anti-friction attributes due to the ridges. This has inspired a solution to a problem in electrosurgery, where tissues are cut using a high-frequency current and an electrosurgical blade, depicted in Figure 14. The problem encountered is that heat is released by the electrosurgical blade, which makes the tissue adhere to this tool. This hinders the precision and efficacy of the cut being made and can therefore be an unsafe procedure (Li et al., 2019).

Fig. 14 Electrosurgical knife (adapted from Li et al., 2019)
Pangolin scales thus raise the idea to replicate microgrooves on the electrosurgical blade. A laser was used to engrave biomimetic scales. This modified blade has similar anti-adhesion properties to pangolin scales. In fact, when tested on a pig’s liver, the biomimetic blade has a friction coefficient that is 14.9% smaller than a normal electrosurgical blade. Also, the mass of the tissue that adhered to the biomimetic blade and the unmodified blade was weighed; the mass of tissue that sticks to the blade is lessened by 16.5% with the biomimetic blade. This further supports that biomimicking pangolin scales for their anti-adhesion property is efficient. Figure 15 shows the difference in friction coefficients and mass when both blades are used.
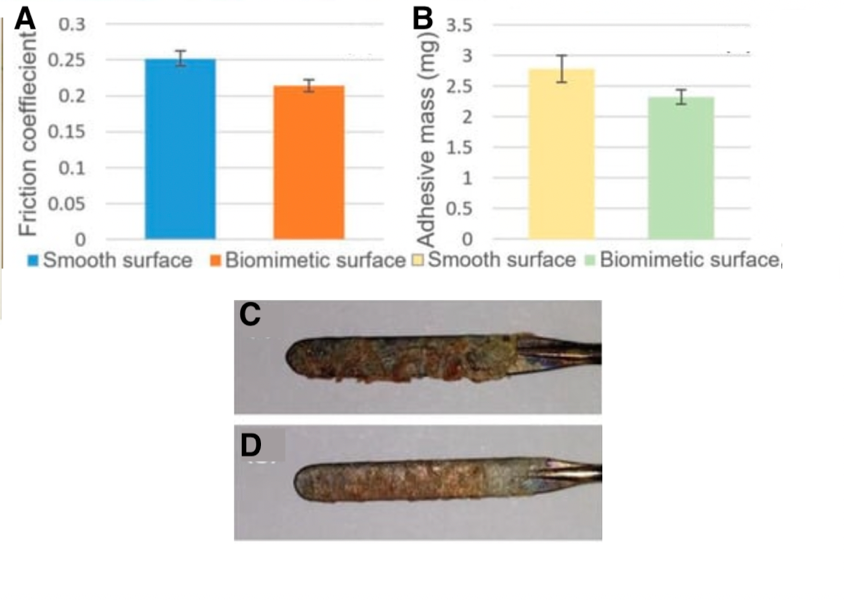
Fig. 15 (A) shows the friction coefficient between the unmodified blade and the pig’s liver in blue, as well as the biomimetic blade and the pig’s liver in orange. (B) shows the mass of the pig’s liver tissue that stuck to the unmodified blade after one cut in yellow (2.78mg). That mass is 2.38 mg for the biomimetic blade, shown in green. The amount of liver tissue that adhered to the unmodified blade can be seen in (C) and to the biomimetic blade in (D) (adapted from Li et al., 2019).
It is interesting to know that jackets have also been made out of pangolin scales, as pictured in Figure 16.

Fig. 16 A coat made out of pangolin scales (Royal Collection Trust, n.d.)
Reptilian
Reptiles are a class of vertebrates which diverged from amphibians approximately 340 million years ago (Rutland et al., 2019). These tetrapods differed from their amphibian successors in that they evolved scales, which allowed them to withstand the harsh conditions of terrestrial life (Rutland et al., 2019). In fact, a new class of proteins, beta-keratins, emerged during this period; these proteins had the function of hardening the corneous layer of skin in reptilian groups, thus producing scales (Alibardi & Toni, 2006). Reptiles’ amphibian ancestors lived in moist environments with little need for external protection from harsh terrain. However, when moving to terrestrial environments, reptiles developed these scales as a protection mechanism against UV radiation, rough terrain, and loss of moisture in their body due to evaporation and transpiration (Chang et al., 2009). For different orders and species of reptiles, the shape, composition and spacing of scales or scutes (large and plate-like scales as can be visualized in Figure 17) differ depending on the requirements for functionality and mobility (Chang et al., 2009; Rutland et al., 2019).

Fig. 17 The scutes of a Nile crocodile (Goetgheluck, 2011). These hard scales are some of the largest among the reptilian class and have little overlap.
For all reptiles, scales are formed in the epidermis and are not supported by bony structures attached to the dermis. Epidermal scales are folds in the surface epidermis which are separated by a junction acting as a flexible hinge and providing the flexibility necessary for movement, as can be seen in Figure 18 (A) (Rutland et al., 2019). These epidermal scales can grow into protrusions and specialize in fulfilling certain functions. They can become toe pads, pits, sensory receptors, spines, horn-like scales, crests, scutes, plastrons and carapaces (Chang et al., 2009; Rutland et al., 2019).
For different orders of reptiles, the histological organization of the epidermis can differ. For all species, the epidermis possesses basal live keratinocytes, which form the stratum basale (germinativum), stratum granulosum, and stratum corneum layers of the epidermis. In contrast, the outermost layer is composed of dead fully differentiated keratinocytes (Rutland et al., 2019). These variable organizations are schematized in Figure 18.

Fig. 18 Histological schematic drawing of the epidermis of various reptile orders (Adapted from Toni et al., 2007). (A) Illustrates the folds in the surface epidermis of reptile scales and the hinge region between these scales. (B) Shows the histological structure of lepidosauria scales in the boxed region indicated in (A), which comprise alternating alpha- and beta-keratin layers, which shed periodically. (C) and (D) illustrate the histological structure of chelonians and crocodilians, respectively, which have hard corneous layers made of beta-keratins and which shed for certain chelonian species.
Keratin is produced by cuboidal dividing cells in the stratum germinativum. Indeed, for most reptile species, Alpha-keratin is produced by alpha cells in the suprabasal layer of the epidermis in the hinge region, in between scales (see Figure 18 A) (Alibardi & Toni, 2006). These alpha keratins in the hinge regions are responsible for the flexibility of reptile skin and allow the scales to move with the movement of the animal. Alpha keratins are also responsible for the water barrier in the skin (Rutland et al., 2019). In the main region of the scales, however, many suprabasal layers can be produced, and the beta-cells in these layers are compacted as they keratinize and mature to constitute the stratum corneum layer, which is the solid layer responsible for physical resistance (Alibardi & Toni, 2006).
For lepidosaurians (a subclass containing lizards, snakes, and sphenodontidae), the keratinocytes in the stratum germinativum layer produce alternating hard (beta) and soft (alpha) keratin layers. For squamates (who belong to the lepididosaurian subclass), the corneous layer of the scales is sloughed periodically, with 5 to 20 moltings per year. During molting, the micro-ornamented oberhäutchen layer is formed first, and the beta, mesos, and alpha layers follow (Alibardi & Toni, 2006). For lepidosaurians, the oberhäutchen layer is responsible for producing species-specific, micro-ornamentation of scales (see Figure 19). The beta layer is made of stratified synthesizing cells and the mesos layer is made of thin cells that stop producing beta-keratin and serves as a transition between the underlying beta layer and the following alpha-layer (Alibardi & Toni, 2006). This histological organization can be seen in Figure 18 B. When molting occurs, the stratum basale duplicates these oberhautchen, beta, mesos, and alpha layers, which push up the previous layers. White blood cells then invade the region between the old and new layers (shedding plane) and help to separate these layers so that the animal can shed its old skin (Rutland et al., 2019).
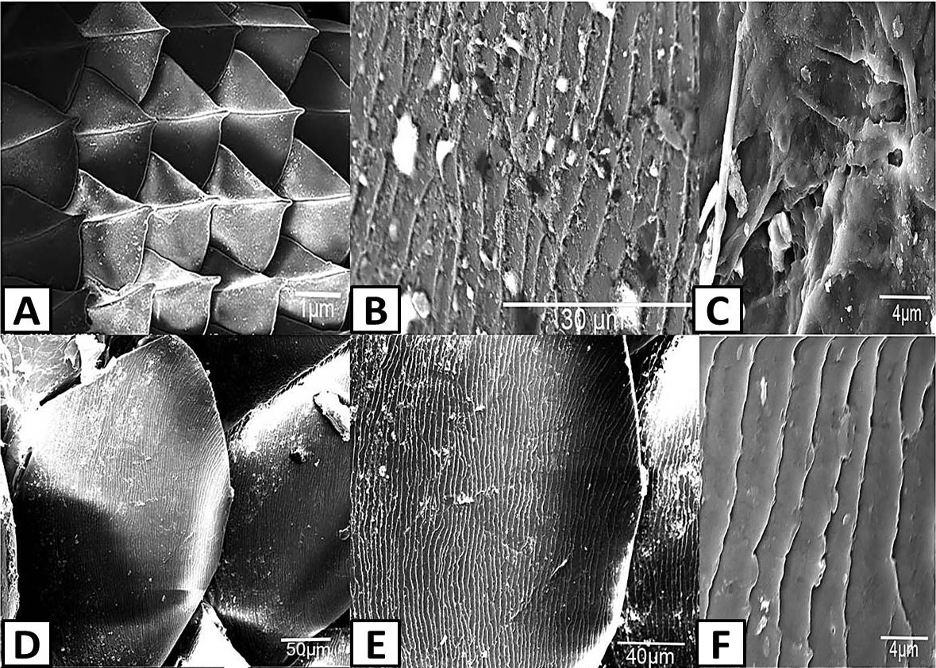
Fig. 19 Scanning electron photomicrographs of scale ornamentation in different lizard species. (Adapted from Abdel-Hady et al., 2021). (A) Shows the scales on the skin of Acanthodactylus opheodurus (snake-tailed fringe-toed lizard) (10x). (B) and (C) Illustrate the micro-ornamentation of pits on the skin of A. opheodurus (3000x and 10000x, respectively). (D), (E) and (F) illustrate the pits on the scales of Mesalina guttulata guttulata (small-spotted lizard) (500x, 1000x and 10000x, respectively).
However, for the shells of chelonians and the scutes of crocodilians, variably thick beta-keratin corneous layers are formed in the epidermis (Toni et al., 2007). Crocodilian scales are generally large and mildly overlapped and are mainly composed of hard beta-keratinized, stratified epidermis, as can be visualized in Figure 18 D. The hinge region is thinner and composed of mainly alpha keratins (Chang et al., 2009). For marine turtles of the chelonian order, there is softer skin in the limbs, tail, neck, and a scaled skin in the shell, which resembles the histological structure of crocodilians (see Figure 18 C) (Chang et al., 2009). For terrestrial turtles, hard scales containing beta-keratin can be found, but there is little beta-keratin in the hinge regions. There are multi-layered corneous layers in the carapace and plastron (Chang et al., 2009). For tuatara and certain turtle species, shedding occurs one to five times per year in a similar way as discussed for lepidosauria. However, for most chelonians and crocodilians, which have more ancient evolutionary ancestors, a gradual wearing of the stratum corneum due to external forces occurs in the place of molting (Toni et al., 2007).
Another particularity of some reptiles is their ability to regrow a tail if the appendage is lost. In this case, the regrowth of scales does not follow the same steps as scale development but rather consists of the invagination of the epidermis into the dermis (Wu et al., 2014).
Reptile skin has thus evolved to be a highly effective protection for these land animals. The particular structure of snake and lizard skin, which is more supple on the inside and tougher in the outer layers, has inspired biomimetic applications for resistant materials which reduce friction. This variable toughness of tissue causes efficient traction between the ground and the skin of reptiles like snakes, which has inspired the design of tires and metallic mechanical materials which are durable and cause little friction (Sanchez et al., 2021).
Feather keratin
- Link between feather and scale in the aspect of keratin
The feathers of contemporary birds are regarded as one of the most complicated skin appendages, and their origin is still a heated discussion topic (Sawyer et al., 2003). A hypothesis suggests that the feathers evolved from reptilian scales (Sawyer et al., 2003). The assumption is supported by the finding of feather-type beta keratins in alligator embryos (Sawyer et al., 2003). Also, the observation of feather-like structures in the archosaurian integument suggests feather appendages evolve before birds, implying the correctness of the hypothesis (Sawyer et al., 2003). Research on epidermal development has shown that, during embryogenesis, the skin of squamates autonomously creates stratified supra-basal cell populations (Sawyer et al., 2003). Studies also demonstrated that scale and feather share similar developmental processes (Sawyer et al., 2003). As shown in Figure 20, some strata contain alpha keratins and are referred to as alpha stratum, while some contain beta keratin and are called beta stratum (Sawyer et al., 2003). Many researchers have shown that the beta stratum constitutes plate-like skin appendages on the scutate scales and is induced from the scale ridge surface during 12 days of embryogenesis (Sawyer et al., 2003). Studies have shown that, after hatching, the strata above the dotted line in Figure 20 will slough off (Sawyer et al., 2003). Therefore, after hatching, feathers only retain feather-like keratin, while scales only keep scale-like keratin (Sawyer et al., 2003). According to Figure 20, as feather-type keratins can be found in periderms of both developing scutate scales and feathers, especially in the sub-periderm of the embryonic scutate scale, it is suggested that cells in scutate periderm and sub-periderm share have a homologous relationship with those in feather’s periderm and barb ridge respectively (Sawyer et al., 2003). Similar cell differentiation supports this idea in the alpha stratum and marginal plate (Sawyer et al., 2003). Therefore, some researchers claimed the evolution of feathers from reptilian scales (Sawyer et al., 2003).

Fig. 20 Diagram for the scutate feather and scale in the embryonic stage: “1。” stands for “primary,” “2。” stands for “secondary,” “PD” refers to periderms, “SH” refers to periderm, “AP” refers to the axial plate, “BR” refers to barb ridge, “MP” refers to marginal plates, “SB” refers to stratum basal, “SP” refers to sub-periderm, “AS” refers to alpha stratum, “BS” refers to beta stratum, “FβK” refers to feather-type beta keratin, “ScβK” refers to scale-type beta keratin, and “αK” refers to alpha keratin (Sawyer et al., 2003). It is shown that periderms from both embryonic feather and scutate scales contain feather-like beta keratins (Sawyer et al., 2003). The sub-periderm of the developing scale also contains feather beta keratin (Sawyer et al., 2003).
- The structure of feather beta keratin
The determination of the feather beta keratin structure has experienced a long term of heated discussions, and various models have been established for it.
The first model was suggested by R. Schor and S. Krimm in 1961. It is found that 12 percent of feather keratin is made up of proline residues (Schor & Krimm, 1961). From that result, Schor and Krimm first assumed those proline residues are arbitrarily attributed along the peptide chain (Schor & Krimm, 1961). However, the structure constructed from that peptide chain cannot follow the ordered beta structure observed from the x-ray diffraction result (Schor & Krimm, 1961). So, they hypothesized that the prolines are periodically aligned along the peptide chain, and this type of peptide proposes the keratin structure of helical conformation named beta-helix structure (Schor & Krimm, 1961). By hydrogen bonds, those helix structures can aggregate and coil together to form cylindrical units (Schor & Krimm, 1961). As shown in Figure 21, those cylindrical sections can be packed to create a cable-like structure (Schor & Krimm, 1961). The model followed the calculation results of the cylindrical Patterson function, equatorial and meridional diffraction pattern, characteristics of elongation, dichroism of infrared, radial Fourier synthesis, chemical reaction’s effects, and density (Schor & Krimm, 1961). Therefore, they claimed that the basic structure of the feather keratins follows their beta-helix model (Schor & Krimm, 1961).
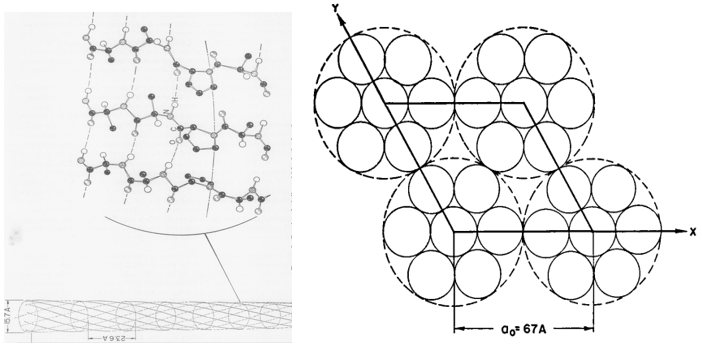
Fig. 21 The cross-section diagrams describe the model of Schor and Krimm (Schor & Krimm, 1958). In the left diagram, the structure of the cylindrical structure is shown (Schor & Krimm, 1958). In the right diagram, a possible packing of cylindrical units (comparatively small solid circles) for forming the cables (comparatively large, dotted circle) is demonstrated (Schor & Krimm, 1961).
Later, Ramachandran claimed that the feather keratin is in a structure of a trigonal helix, which is a collagen-type structure (Astbury & Beighton, 1961). However, the NH-stretching frequency is 3330 cm^ (-1), higher than the acceptable range of that data for other keratins, including the feather one (about 3300 cm^ (-1),) showing the unreliability of the whole model (Astbury & Beighton, 1961).
After the proposition of a model of Schor and Krimm, W. T. Astbury and E. Beighton claimed another model. The x-ray diffraction shows that the feather keratin is in the beta type with a residue length of 3.1 Å (Astbury & Beighton, 1961). The residue length is different from that of other regular beta keratin and silk fibroin, which are about 3.34 Å and 3.5 Å, respectively, showing that feather keratin is a special type of beta keratin (Astbury & Beighton, 1961). Bear and Rugo found that the feather keratin contains a net of nodes with the main chain and side chain direction (Bear & Rugo, 1951). Based on that finding, Astury and Beighton suggested the basic unit of feather keratin is a cyclic polypeptide with 32 amino acid residues (Astbury & Beighton, 1961). As feather keratin has a high proportion (about 1/10) of proline residues, they also supposed the peptides are parallel polar (Astbury & Beighton, 1961). More precisely, all peptide chains run in the same direction instead of screwing together, and all their CO groups are on one side and all their NH groups are on another side (Astbury & Beighton, 1961). Because of the prevention by the proline residues, it is unavailable to make the side-to-side connection of loops (Astbury & Beighton, 1961). Therefore, they assumed that, by the hydrogen bond between the carboxylic group and amino group, there are three cyclic polypeptide units in beta conformation, like pleated sheets (Astbury & Beighton, 1961). Based on this model, the limiting corpuscle weight calculated is 6400 g/mol, slightly lower than Woodin’s physio-chemical estimate of about 10000 g/mol, and the calculated density is 1.32 g/mL, like the observed density of 1.27 g/mL (Astbury & Beighton, 1961). As the difference of the above data is comparatively small for a higher polymer, Astbury and Beighton claimed their model is accurate (Astbury & Beighton, 1961). The visualization of their model is shown in Figure 22.

Fig. 22 Diagrams describing the model of Astbury and Beighton. Diagram (a) shows the single cyclic polypeptide made up of 32 amino acid residues (dotted points) (Astbury & Beighton, 1961). The “u” means that, in the direction of pointing out the page, there is an amino acid from another cyclic unit making a hydrogen bond with it, while the “d” means that there is an amino acid from another loop unit forming a hydrogen bond with it in the direction of pointing inside the page (Astbury & Beighton, 1961). Diagram (b) shows a possible arrangement of the pleated sheets, and the loop in the diagram represents the cyclic unit in the pleated sheets (Astbury & Beighton, 1961).
In 1971, R. D. B. Fraser, T. P. MacRae, D. A. D. Parry, and E. Suzuki claimed their model (Fraser et al., 1971). The model somehow makes a compromise between that of Schor and Krimm and that of Astbury and Beighton, and it is currently the most cited model. By blocking the thiol groups to avoid oxidation and decreasing the number of crystal residues, soluble materials with over 80 percent emu feather can be derived, and those extracts can create a film for producing purified x-ray diffraction (Fraser et al., 1971). As a result, the purified diffraction pattern at 4.8 Å and 2.4 Å can be shown from the films, suggesting the feather keratin is in a cross-beta structure (Fraser et al., 1971). It presents the possibility that, in the feather keratin, pleated sheets are getting together side to side, like the model of Astbury and Beighton (Fraser et al., 1971). As Schor and Krimm have shown the helix structure of the feather keratins with evidence, Fraser’s team hypothesizes a tilt in the cross-beta structure without destroying the hydrogen bond, which is shown in Figure 23 (Fraser et al., 1971). The tilting makes the whole structure a distorted helical conformation with an empty axial region (shown in Figure 24) (Fraser et al., 1971). And it is also found that if it is not empty in the axial region, the calculated diffraction pattern based on the model will not follow the observed x-ray diffraction data, proving the existence of the empty axial regions (Fraser et al., 1971). Based on the x-ray diffraction analysis, they get the calculation results that the basic structure of the feather keratins contains two parallel sheets with an inter-chain distance of approximately 4.7 Å and with about four peptide chains per sheet, and each chain has eight amino acid residues (Fraser et al., 1971). Subsequently, they proposed that the structure constitutes an asymmetrical unit with the distance between two sheets of about 5 Å (Fraser et al., 1971). Then, it was concluded that the basic keratin structure would be a double helical configuration with four repeating asymmetric units for each turn, fully explaining the existence of the empty axial region and the distorted single helical structure (Fraser et al., 1971). Therefore, Fraser’s team suggested the structure of the feather beta keratin should be a helical conformation with two strands, just like the one shown in Figure 24 (Fraser et al., 1971). From Figure 25, the electron micrograph provides the model of Fraser’s team with strong evidence (Fraser & Parry, 2008; Filshie & Rogers, 1962).
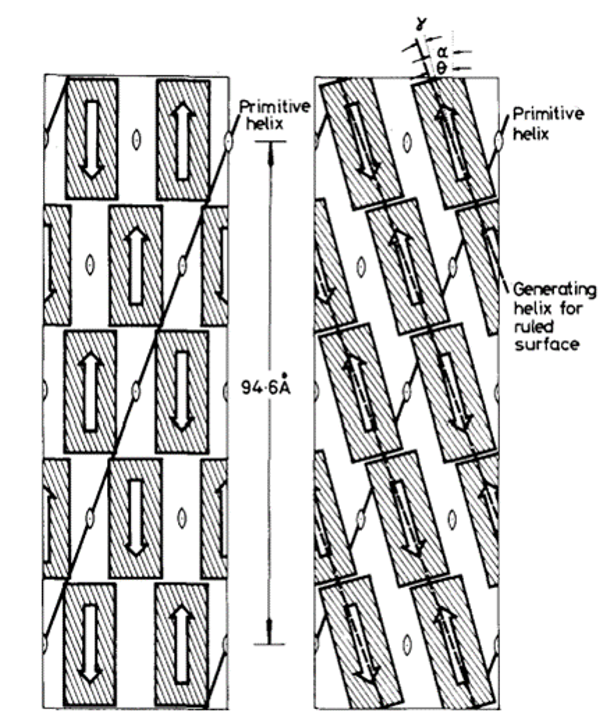
Fig. 23 The diagram shows how Fraser tilts the originally assumed structure (Fraser et al., 1971).
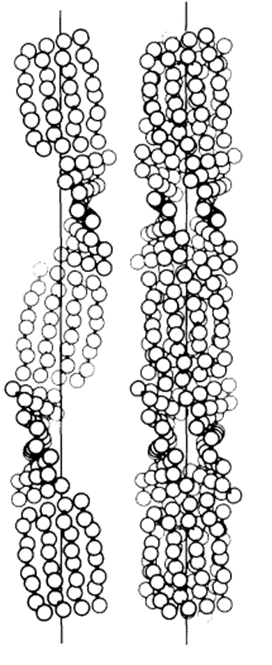
Fig. 24 The left diagram shows the distorted single helical structure, and the right diagram demonstrated the complete model of the double-strand helix structure (Fraser et al., 1971).
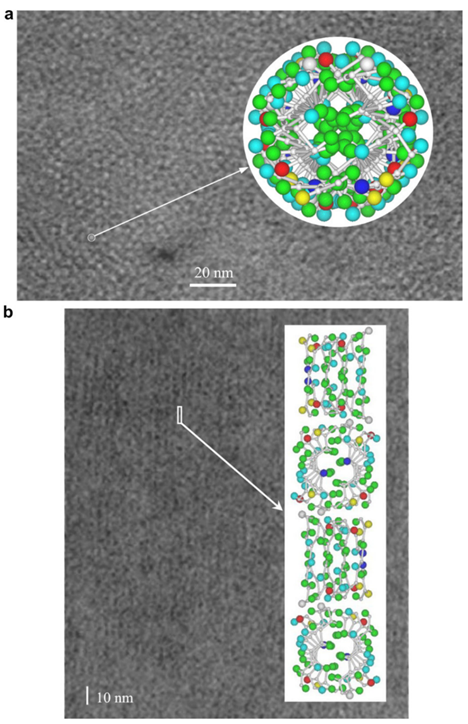
Fig. 25 The electron micrograph of feather keratin (Fraser & Parry, 2008; Filshie & Rogers, 1962). Picture (a) demonstrates the detail in the cross-section of the feather rachis, while picture (b) shows the details of seagull’s feather rachis in the longitudinal orientation (Fraser & Parry, 2008; Filshie & Rogers, 1962). Both pictures are compared with the diagrams for the model of Fraser’s team (Fraser & Parry, 2008; Filshie & Rogers, 1962). And both pictures proved that the actual structure of keratins followed the model of Fraser’s team (Fraser & Parry, 2008; Filshie & Rogers, 1962).
- Applications of the feather keratins
It is shown that feather keratins can be used for improving the quality of scaffolds or films utilized for growing cells, enhancing the effectiveness of skin and bone tissue engineering (Saravanan et al., 2013; Das et al., 2021; Esparza et al., 2017). As feathers, especially chicken feathers, are one of the main wastes in poultry, this application would be at a comparatively low cost and suggest a solution for addressing those wastes (Saravanan et al., 2013).
The feather keratin can participate in the improvement of a chitosan scaffold in tissue engineering. Through crosslinking reactions between feather keratin and glutaraldehyde, researchers get the spherical keratin nanoparticles shown in Figure 26 (Saravanan et al., 2013). Those nanoparticles can well combine with the chitosan to form a new scaffold for cells to grow (Saravanan et al., 2013). The microstructures of chitosan scaffolds and the new scaffold are shown in Figure 27 (Saravanan et al., 2013). It was observed that the existence of the keratin particles along the scaffold’s pores can incline the adhesion of the cell to the scaffold (Saravanan et al., 2013). The chitosan’s semi-crystalline property was not changed after the combination with the keratin particles, so the new scaffolds are still stiff enough to hold cells (Saravanan et al., 2013). Furthermore, osteoblastic cells were implanted into the new scaffold, and no significant cytotoxicity was shown, meaning that this new scaffold can be used for tissue engineering in bones without severe side effects (Saravanan et al., 2013). The new scaffold can be degraded by lysozyme by cutting the glycosidic connections between the N-acetyl glucosamine groups (Saravanan et al., 2013).
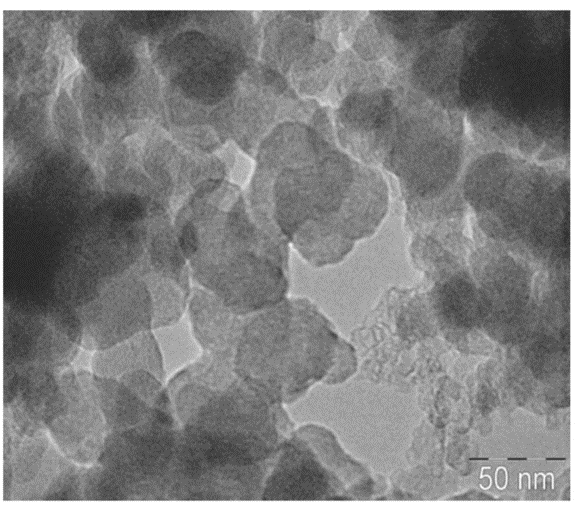
Fig. 26 The picture of keratin nanoparticle’s transmission electron microscopic analysis (Saravanan et al., 2013). From the picture, the nanoparticles are spherical (Saravanan et al., 2013).
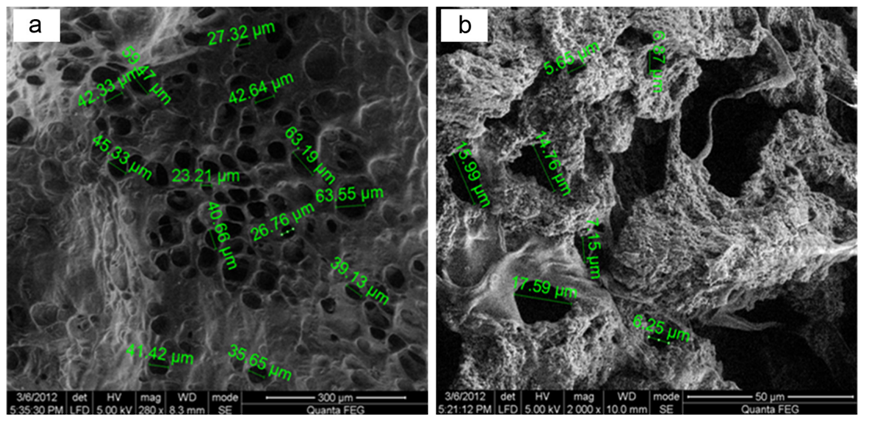
Fig. 27 The left scanning electron microscopic picture shows the microstructure of the chitosan scaffold, while the right one presents the microstructure of the new scaffold (Saravanan et al., 2013). The sizes of pores in each scaffold are noted (Saravanan et al., 2013). Although the pore in the new scaffold is smaller than the original one, the new scaffold swills when meeting body fluid, and the enlarged pores will fit most of the body cells (Saravanan et al., 2013).
Some researchers also invented a unique method of building scaffolds with feather keratins (Esparza et al., 2017). Researchers solubilized feather keratins in an alkaline solution and mixed them with aqueous polyvinyl alcohol (PVA) and citric acid solution by thermal crosslinking of PVA’s hydroxyl groups (Esparza et al., 2017). Then, they used electrospinning to form polymer fibers shown in Figure 28 (Esparza et al., 2017). Like the previous technology, keratin can enhance fiber’s adhesion to cells by further improving the hydrophilicity of the polymers (Esparza et al., 2017). As shown in the Figure 29, after comparing the fibers with keratin to PVA ratios of 0 percent, 10 percent, 20 percent, and 30 percent, the scaffolds formed by fiber with keratin to PVA ratio of 20 percent can provide a comparatively suitable environment for cells to grow (Esparza et al., 2017). According to Figure 30, in the test of incubating osteoblasts in the scaffold of the four different ratios, the one with 20 percent feather keratins gives rise to more cell population (Esparza et al., 2017). Therefore, scaffolds with fibers of the 20 percent ratio can also participate in cell engineering in bones (Esparza et al., 2017). Although a scaffold made by such a type of fiber is packed and forms relatively small pores to body cells, air deposition, and controlling charges in the polymer solution could allow the whole scaffold to be less packed, encouraging cell penetrations (Esparza et al., 2017).
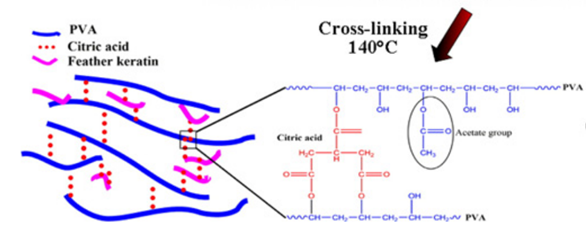
Fig. 28 The diagram shows the connections between PVA feather keratins, and citric acid (Esparza et al., 2017).
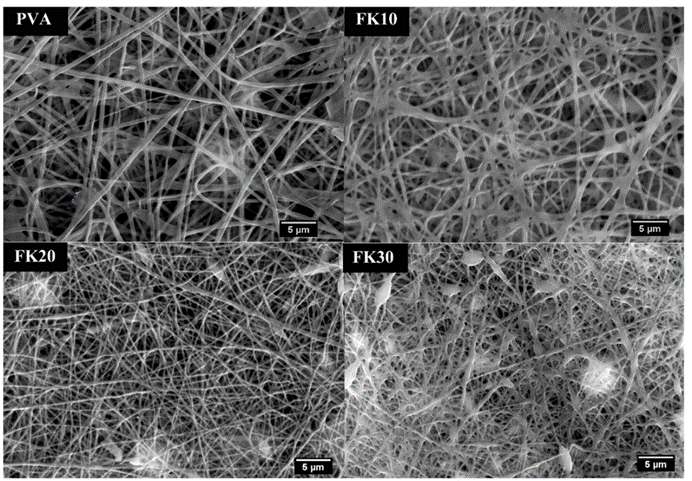
Fig. 29 The scanning electron microscopic pictures for fibers with keratin to PVA ratio of 0 percent (PVA,) 10 percent (FK10,) 20 percent (FK20,) and 30 percent (FK30) (Esparza et al., 2017). It is shown that, for fiber with the ratio from 0 to 20 percent, the fiber gradually goes thinner, providing more space for cell proliferation (Esparza et al., 2017). However, when the ratio reaches 30 percent, the fibers start to clump together, which is unbeneficial for cell survival (Esparza et al., 2017). The scaffold with fibers of the ratio 20 percent can provide better space for cell growth (Esparza et al., 2017).
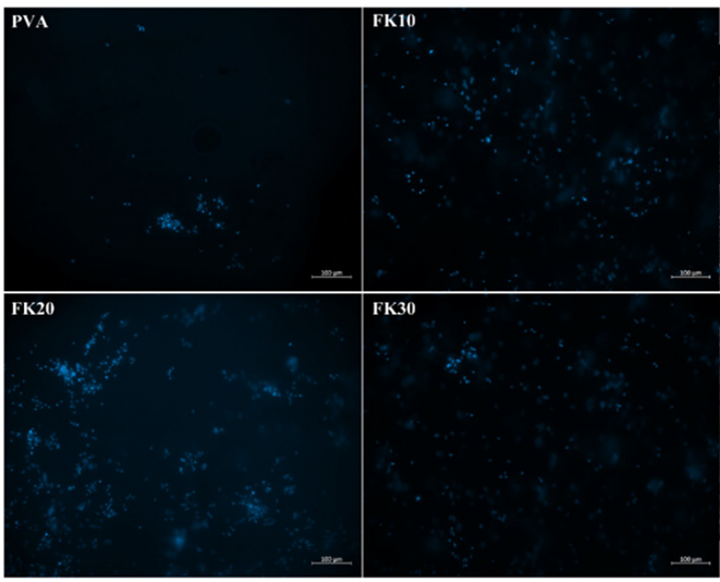
Fig. 30 Fluorescence micrographs compare cell proliferation of osteoblasts in scaffolds by fibers with keratin to PVA ratio of 0 percent (PVA,) 10 percent (FK10,) 20 percent (FK20,) and 30 percent (FK30) (Esparza et al., 2017). Those blue points are cell nuclei (Esparza et al., 2017). It is demonstrated that the scaffold made up of fibers with the ratio 30% provides the best cell population (Esparza et al., 2017).
The feather keratins can also react with and improve films for tissue engineering (Das et al., 2021). As shown in Figure 31, by reacting guar gum with aprotic solvent and Hofmeister ions, guar gum indole acetate (GGIA) is produced (Das et al., 2021). According to Figure 32, feather keratins from chickens are hydrolyzed and crosslinked to the GGIA, producing a bio-based film scaffold (Das et al., 2021). Glycerol in the film plays a role in increasing the elongation at break, inclining the film’s flexibility and extensibility (Das et al., 2021). And the complex interaction between glycerol’s hydroxyl groups and polypeptide chains enhances the polymer’s mobility in the scaffold (Das et al., 2021). Consequently, the scaffold has a tensile strength of 6.4 MPa at the breaking point and 63 percent porosity, showing crystalline properties enough for cell incubation (Das et al., 2021). Like the technology previously mentioned, the moderately hydrophilic properties of the keratins improve cell adhesion and migration on the film (Das et al., 2021). And, mildly hydrophilic characteristics of the feather keratins can reduce the water loss of the scaffold, providing adequate hydration for the cell growth on the film (Das et al., 2021). The film will also swell when meeting body fluid, providing enough space for cells to interact during the regeneration of tissues (Das et al., 2021). Furthermore, the film demonstrates the antimicrobial interaction on 130 μg/mL E. coli and 211 μg/mL S. aureus, meaning the scaffold can inhibit bacterial growth, as shown in Figures 33 and 34 (Das et al., 2021). According to Figure 35, it is shown that the scaffold is biocompatible with human dermal fibroblast cells, meaning the film scaffolds can be applied in skin tissue engineering (Das et al., 2021).
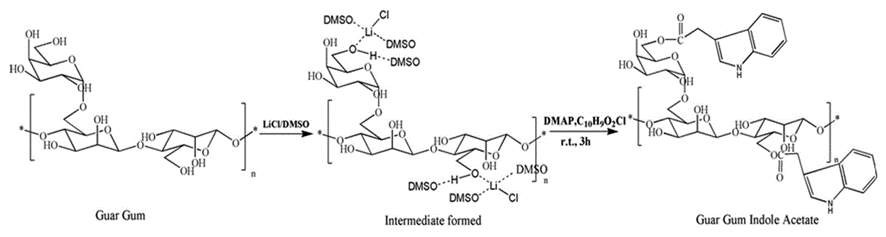
Fig. 31 The reaction scheme shows the synthesis of guar gum indole acetate (Das et al., 2021).
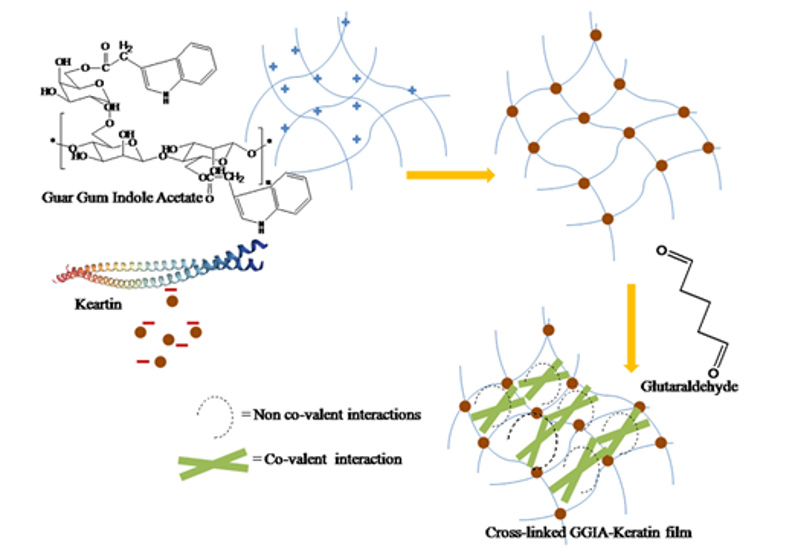
Fig. 32 The diagram shows the production of the GGIA-Keratin film (Das et al., 2021).
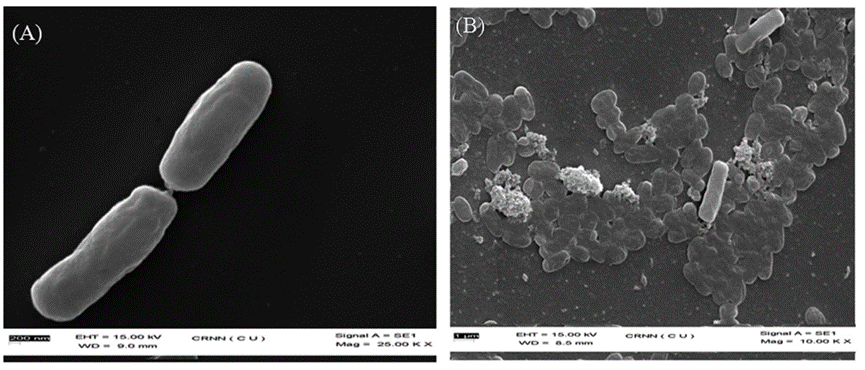
Fig. 33 The scanning electron microscopic images for E. coli (A) and E. coli. Treated with the GGIA-Keratin film (B) (Das et al., 2021). Massive elimination of E. coli is present on the film (Das et al., 2021).
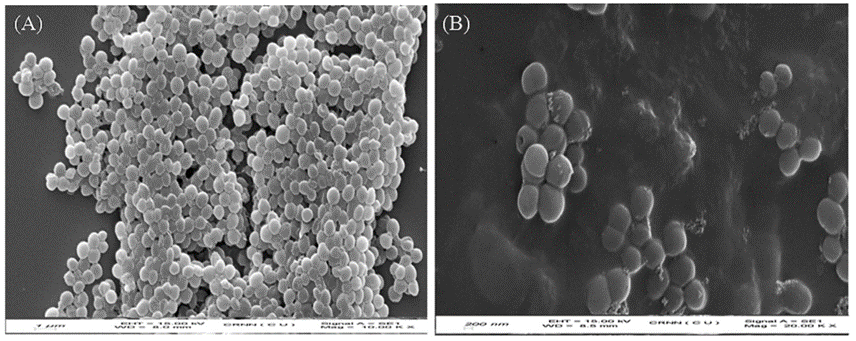
Fig. 34 The scanning electron microscopic images for the S. aureus (A) and S. aureus treated with GGIA-Keratin film (B) (Das et al., 2021). Numerous deaths of S. aureus demonstrated on the film (Das et al., 2021).

Fig. 35 The pictures show the incubation of human dermal fibroblast cells on the GGIA-Keratin film in 72 hours (Das et al., 2021). The cell nuclei are blue-stained (Das et al., 2021). Cell proliferation and qualified biocompatibility is demonstrated on the film (Das et al., 2021).
Conclusion
This essay compared the composition and evolutionary origins of pangolin and reptile scales and evoked the similarities of these reptile and mammalian scales with bird feathers, an epidermal protrusion evolved from scales. Specifically, this comparison focuses on the structural role of keratins and the unique keratins used in all three of these structures.
Keratins are a family of proteins dominated by either alpha-helix or beta-sheet secondary structures that polymerize to form resilient structures. The stability of keratins is further improved by disulfide bridging, coiled-coil tertiary structures, and hydrophobic interactions. The toughness of keratin makes it an ideal protein for use in the protective structures on the outside of the epidermis, such as scales and feathers. The structural role of these proteins was thus explored in detail for all pangolins, reptiles and birds, as well as the particularities of each of these structures on a larger functional scale.
Pangolin scales are made of three different layers, all made of keratinized scales. Moreover, the unique layout of their scales ensures protection from any predator. Pangolin scales can be healed from damages inflicted by predators by being submerged in water. The evolution of scales in pangolins is explained through their genes. Essentially, these animals do not possess interferon genes that typically help other mammals with immunity, inflammation, and skin healing, so their scales evolved to make up for this lack of defenses. Pangolin scales have anti-adhesion properties due to their digging behaviors, which has inspired the design of an electrosurgical blade that does not stick to tissues.
Reptile scales are formed from the folding of the epidermis of the animal and are primarily made of beta-keratins for the scales and alpha-keratins in the hinge region between the scales (Rutland et al., 2019). These reptile scales were an evolutionary adaptation which emerged when the amphibian ancestors of reptiles progressed to terrestrial terrain; they were advantageous as physical protection and as a barrier to prevent moisture loss on land (Rutland et al., 2019). The histology of reptile epidermal scales varies between orders and species of reptiles. Crocodilian and chelonian scales have simpler organizations which comprise beta keratins and do not molt for most species. Meanwhile, lepidosauria have alternating alpha and beta keratin layers which make scales more supple and molt periodically.
Due to the existence of feather-type beta-keratin in embryonic scales and feathers, a theory suggested the evolution from scales to feathers (Sawyer et al., 2003). Several models are proposed to explain the structure of feathers (Schor & Krimm, 1961; Astbury & Beighton, 1961; Fraser et al., 1971). The most popular model suggests that the basic structure of feather keratin in such a double strand helix structure is quite different from that of the common feather keratin (Fraser et al., 1971). The application of feather keratin includes the participation in building scaffolds for bone tissue engineering and films for skin tissue engineering (Saravanan et al., 2013; Das et al., 2021; Esparza et al., 2017).
References
Abdel-Hady, A. E., El-Amir, S. M., Al-Muttri, N. S., & El-Hariri, H. M. (2021). Scale Microornamentation in Some Lizard Species. Pakistan Journal of Zoology, 54(1). https://doi.org/10.17582/journal.pjz/20200421090425
Albers, K. M. (1996). Keratin biochemistry. Clinics in dermatology, 14(4), 309-320.
Alibardi, L. (2016). The process of cornification evolved from the initial keratinization in the epidermis and epidermal derivatives of vertebrates: a new synthesis and the case of sauropsids. International review of cell and molecular biology, 327, 263-319.
Alibardi, Lorenzo & Toni, Mattia. (2006). Cytochemical, biochemical and molecular aspects of the process of keratinization in the epidermis of reptilian scales. Progress in Histochemistry and Cytochemistry, 40(2), 73–134. https://doi.org/10.1016/j.proghi.2006.01.001
Astbury, W. T., & Beighton, E. (1961). Structure of feather keratin. Nature, 191(4784), 171-173. https://doi.org/10.1038/191171b0
Bear, R. S., & Rugo, H. J. (1951). The results of X-ray diffraction studies on keratin fibers. Annals of the New York Academy of Sciences, 53(3), 627-648. https://doi.org/10.1111/j.1749-6632.1951.tb31964.x
Chang, C., Wu, P., Baker, R. E., Maini, P. K., Alibardi, L., & Chuong, C.-M. (2009). Reptile scale paradigm: Evo-Devo, pattern formation and regeneration. The International Journal of Developmental Biology, 53(5–6), 813. https://doi.org/10.1387/ijdb.072556cc
Choo, S. W., Payko, M., Tan, T. K., Hari, R., Komissarov, A., Yee Wee, W., Yurchenko, A. A., Kliver, S., Tamazian, G., Autunes, A., Wilson, R. K., Warren, W. C., Koepfli, K. Minx, P., Krasheninnikova, K., Kotze, A., Dlaton, D. L., Vermaak, E., Paterson, I. C., … Wong, G. J. (2016). Pangolin genomes and the evolution of mammalian scales and immunity. Genome Research, 32(9), 1312-1322. https://doi.org/10.1101/gr.203521.115
Das, A., Das, A., Basu, A., Datta, P., Gupta, M., & Mukherjee, A. (2021). Newer guar gum ester/chicken feather keratin interact films for tissue engineering. International Journal of Biological Macromolecules, 180, 339-354. https://doi.org/10.1016/j.ijbiomac.2021.03.034
Esparza, Y., Ullah, A., Boluk, Y., & Wu, J. (2017). Preparation and characterization of thermally crosslinked poly(vinyl alcohol)/feather keratin nanofiber scaffolds. Materials & Design, 133, 1-9. https://doi.org/10.1016/j.matdes.2017.07.052
Filshie, B. K., & Rogers, G. E. (1962). An electron microscope study of the fine structure of feather keratin. Journal of Cell Biology, 13(1), 1-12. https://doi.org/10.1083/jcb.13.1.1
Fraser, R. B., & Parry, D. A. (2008). Molecular packing in the feather keratin filament. Journal of Structural Biology, 162(1), 1-13. https://doi.org/10.1016/j.jsb.2008.01.011
Fraser, R., Macrae, T., Parry, D., & Suzuki, E. (1971). The structure of feather keratin. Polymer, 12(1), 35-56. https://doi.org/10.1016/0032-3861(71)90011-5
Gilbert, S. F. (2000). The epidermis and the origin of cutaneous structures. Developmental biology, 6.
Goetgheluck, P. (2011) Skin of a young Nile crocodile [Photograph]. Science Photo Library. https://www.sciencephoto.com/media/453396/view
Grus, W. E., & Zhang, J. (2008). Human Lineage-specific Gene Inactivation. Encyclopedia of Life Sciences. Retrieved October 25, 2022, from http://websites.umich.edu/~zhanglab/publications/2008/Grus_2008_ELS.pdf.
Jin, X., Chua, H. Z., Wang, K., Li, N., Zheng, W., Pang, W., Yang, F., Pang, B., Zhang, M., & Zhang, J. (2021). Evidence for the medicinal value of Squama Manitis (pangolin scale): A systematic review. Integrative medicine research, 10(1), 100486. https://doi.org/10.1016/j.imr.2020.100486
Kandyel, R. M., Elwan, M. M., Abumandour, M. M. A., & El Nahass, E. E. (2021). Comparative ultrastructural-functional characterizations of the skin in three reptile species; Chalcides ocellatus, Uromastyx aegyptia aegyptia, and Psammophis schokari aegyptia (FORSKAL, 1775): Adaptive strategies to their habitat. Microscopy Research and Technique, 84(9), 2104–2118. https://doi.org/10.1002/jemt.23766
Li, C., Yang, Y., Yang, L., & Shi, Z. (2019). Biomimetic Anti-adhesive Surface Microstructures on Electrosurgical Blade Fabricated by Long-Pulse Laser Inspired by Pangolin Scales. Micromachines, 10(12), 816-825. https://doi.org/10.3390/mi10120816
Liu, Z., Q., Jiao, D., Weng, Z. F., & Zhang, Z. F. (2016a). Water- assisted self-healing and property recovery in a natural dermal armor of pangolin scales. Journal of the Mechanical Behavior of Biomedical Materials, 56, 14-22. https://doi-org.proxy3.library.mcgill.ca/10.1016/j.jmbbm.2015.10.031
Liu, Z., Q., Jiao, D., Weng, Z. F., & Zhang, Z. F. (2016b). Structure and mechanical behaviors of protective armored pangolin scales and effects of hydration and orientation. Journal of the Mechanical Behavior of Biomedical Materials, 56, 165-174. https://doi-org.proxy3.library.mcgill.ca/10.1016/j.jmbbm.2015.11.013
Royal Collection Trust. (n.d.). Coat of scale armour. https://www.rct.uk/collection/38059/coat-of-scale-armour
Rutland, C. S., Cigler, P., Kubale, V., Rutland, C. S., Cigler, P., & Kubale, V. (2019). Reptilian Skin and Its Special Histological Structures. In Veterinary Anatomy and Physiology. IntechOpen. https://doi.org/10.5772/intechopen.84212
Sánchez, J. C., Estupiñán, H., & Toro, A. (2021). Friction response of bioinspired AISI 52100 steel surfaces texturized by photochemical machining. Surface Topography: Metrology and Properties, 9(1), 014001. https://doi.org/10.1088/2051-672X/abe090
Saravanan, S., Sameera, D., Moorthi, A., & Selvamurugan, N. (2013). Chitosan scaffolds containing chicken feather keratin nanoparticles for bone tissue engineering. International Journal of Biological Macromolecules, 62, 481-486. https://doi.org/10.1016/j.ijbiomac.2013.09.034
Sawyer, R. H., Salvatore, B. A., Potylicki, T. F., French, J. O., Glenn, T. C., & Knapp, L. W. (2003). Origin of feathers: Feather beta (β) keratins are expressed in discrete epidermal cell populations of embryonic scutate scales. Journal of Experimental Zoology, 295B(1), 12-24. https://doi.org/10.1002/jez.b.5
Schor, R., & Krimm, S. (1961). Studies on the structure of feather keratin. Biophysical Journal, 1(6), 489-515. https://doi.org/10.1016/s0006-3495(61)86904-x
Tong, J., Ren, L.-Q., & Chen, B.-C. (1995). Chemical constitution and abrasive wear behaviour of pangolin scales. Journal of Materials Science Letters, 14, 1468-1470. https://doi.org/10.1007/BF00462216
Toni, M., Dalla Valle, L., & Lorenzo, A. (2007, August 18). Hard (Beta-)Keratins in the Epidermis of Reptiles: Composition, Sequence, and Molecular Organization (world) [Review-article]. ACS Publications; American Chemical Society. https://doi.org/10.1021/pr0702619
Wang, B., Sullivan, T. S. (2017). A review of terrestrial, aerial and aquatic keratins: the structure and mechanical properties of pangolin scales, feather shafts and baleen plates. Journal of the Mechanical Behavior of Biomedical Materials, 76, 4-20. https://doi-org.proxy3.library.mcgill.ca/10.1016/j.jmbbm.2017.05.015
Wang, B., Yang, W., Sherman, V. R., & Mayers, M. A. (2016). Pangolin armor: Overlapping, structure, and mechanical properties of the keratinous scales. Acta Biomaterialia, 41, 60-74. https://doi.org/10.1016/j.actbio.2016.05.028
What is a Pangolin? (n.d). Save Pangolins. Retrieved October 25, 2022, from https://www.savepangolins.org/what-is-a-pangolin
World Wildlife Fun. (n.d.). Pangolin. https://www.worldwildlife.org/species/pangolin
Wu, P., Alibardi, L., & Chuong, C.-M. (2014). Regeneration of reptilian scales after wounding: Neogenesis, regional difference, and molecular modules. Regeneration, 1(1), 15–26. https://doi.org/10.1002/reg2.9
Zhang, W., & Fan, Y. (2021). Structure of keratin. In Fibrous Proteins (pp. 41-53). Humana, New York, NY.
Zhou, T., Cai, Z., Li, Z., Li, W., & Zheng, J. (2020). Effect of hydration on mechanical characteristics of pangolin scales. Journals of Materials Science, 55(10), 4420-4436. https://doi.org/10.1007/s10853-019-04322-w